Abstract
On one hand, the application of anti-microbial peptides (AMPs) in the construction of AMPs-mediated drug delivery system has not yet been fully exploited; on the other hand, its non-selectivity in vivo has also limited its clinical application. In this work, we chose one pH-responsive peptide, [D]-H6L9, and functionalized it onto the surface of liposomes (D-Lip). The protonation of histidines in the sequence of [D]-H6L9 under pH 6.3 could switch the surface charge of D-Lip from negative (under pH 7.4) to positive (under pH 6.3), and the cellular uptake and tumor spheroids uptake were increased accordingly. Lysosome co-localization assay suggested that there was only little overlap of D-Lip with lysosomes in 12 h, which indicated that D-Lip could escape lysosomes effectively. In vivo biodistribution assay on C26 tumor-bearing BALB/C mice showed that DiR-labeled D-Lip could reach tumors as much as PEG-Lip, and both tumor slices and quantitative measurement of dispersed cells of in vivo tumors by flow cytometry demonstrated that D-Lip could be taken up by tumors more efficiently. Therefore, we have established an anti-microbial peptide-mediated liposomal delivery system for tumor delivery.
Introduction
Anti-microbial peptides (AMPs), also termed as host-defense peptides, as the names suggests, are a class of peptides that possess direct anti-microbial activities (Zasloff, Citation2002; Reddy et al., Citation2004; Di Francesco, Citation2013). These peptides generally existed in almost all the life forms and were known for not only its antimicrobial activity but also their innate modulation on the immune response through a myriad of mechanisms (Hancock & Sahl, Citation2006). The exact mechanism through which they exerted their antimicrobial property was yet unknown, but it was accepted generally that most of them have membrane-perturbing effect and could traverse through the bio-membrane and killed targeted microbe (Brown & Hancock, Citation2006; Guaní-Guerra et al., Citation2010; Mangoni, Citation2011).
On one hand, currently, several AMPs were under clinical evaluations (Guaní-Guerra et al., Citation2010); on the other hand, many researchers shifted their attention on utilizing AMPs as potential alternatives in cancer treatment due to their membrane-destabilization ability (Nguyen et al., Citation2011; Riedl et al., Citation2011; Yeung et al., Citation2011; Attoub, Citation2013), for their major target of action was the cellular membranes, therefore, avoiding the formation of resistance of tumor cells (Papo & Shai, Citation2005). Introducing AMPs into therapeutics required both sufficient activity of peptides against targeted microbes or tumor cells and most importantly, satisfactory safety (Fjell et al., Citation2011). [D]-K6L9, a peptide with potent therapeutic capacity, could still cause significant systemic toxicity at concentrations slightly higher than the therapeutic one (Papo et al., Citation2006; Makovitzki et al., Citation2009). However, substituting all the lysines in the peptide sequence into histidines ([D]-H6L9), a well-known amino acid with an imidazole ring and protonation effect, could accord pH-responsiveness to the peptide and reduce its non-selective penetration under pH 7.4, decreasing systemic cytotoxicity (Makovitzki et al., Citation2009). In fact, this methodology has been widely employed in designing peptides with pH-dependent activity, be it AMP or cell penetrating peptide (CPP) (Tu et al., Citation2009; Zhang et al., Citation2011; Chen et al., Citation2012; Jiang et al., Citation2012; Tian & Bae, Citation2012; Zhao et al., Citation2012), as solid tumors were notable for their high acidity due to low oxygen tension and anaerobic glycolysis (Tannock & Rotin, Citation1989; Hancock & Sahl, Citation2006).
Now that it has been reported that [D]-H6L9 could permeate tumor cell membranes under acidified environment with its pH-selective membrane-activity, we thereby made a bold and reasonable hypothesis that if [D]-H6L9 could be modified onto certain drug delivery vehicles, it could afford the vehicles with its pH-triggered cellular membrane penetration activity and result in pH-responsive delivery of its cargos into acidic tumor area instead of other normal tissue with normal pH. Moreover, [D]-H6L9 could cause pH-dependent cellular membrane lysis, which meant ideally it was possible for [D]-H6L9 or [D]-H6L9-modified vehicle to escape from lysosomes to realize excellent delivery outcome. [D]-H6L9-modified vehicle has not yet been investigated before, and we chose liposomes as the candidate vehicle in this article to preliminarily study the properties of [D]-H6L9-modified liposomes (D-Lip).
Materials and methods
Materials and animals
SPC (soybean lecithin) was purchased from Shanghai Taiwei Chemical Company (Shanghai, China). Cholesterol was purchased from Kelong Chemical Company (Chengdu, China). DSPE-PEG2000 and 2-dioleoyl-sn-glycero-3- phosphoethanolamine-N-(carboxyfluorescein) (CFPE) were purchased from Avanti Polar Lipids (Alabaster, AL). DSPE-PEG2000-Mal was purchased from Shanghai Advanced Vehicle Technology (AVT) L.T.D. Company (Shanghai, China). [D]-H6L9 peptide with a terminal cysteine (LHLLHHLLHHLLHLL-Cys, the underlined letters were D-amino acids) was synthesized according to the standard solid phase peptide synthesis by ChinaPeptides Co. Ltd. (Shanghai, China). Poly-lysine, amiloride, chlorpromazine, and genistein were all purchased from Sigma-Aldrich (St. Louis, MO). 1,10-Dioctadecyl-3,3,30,30-tetramethylindotricarbocyanine iodide (DiR) and 1,1′-dioctadecyl-3,3,3′,3′-tetramethylindodicarbocyanine, and 4-chlorobenzenesulfonate salt (DiD) were purchased from Biotium (Hayward, CA). Lysotracker™ was obtained from Invitrogen (Carlsbad, CA). 6-Diamidino-2-pheylindole (DAPI) was purchased from Beyotime Institute Biotechnology (Haimen, China). Plastic cell culture dishes and plates were purchased from Wuxi NEST Biotechnology Co. (Wuxi, China). Other chemicals and reagents were of analytical grade.
BALB/C mice purchased from experiment animal center of Sichuan University (PR China). All animal experiments were performed in accordance with the principles of care and the use of laboratory animals was approved by the experiment animal administrative committee of Sichuan University.
Synthesis of DSPE-PEG2000-[D]-H6L9
DSPE-PEG2000-Mal (3 μmol) was dissolved in chloroform containing triethylamine, and [D]-H6L9 with a terminal cysteine (4.5 μmol) was dissolved in methanol and added into the chloroform solution of DSPE-PEG2000-Mal. The reaction was allowed to react with gentle stirring in the protection of nitrogen for about 24 h in darkness. After the confirmation of complete consumption of DSPE-PEG2000-Mal by thin-layer chromatography, the organic solvent was filtered and the filtrate was evaporated by rotary evaporation. The purification of production was carried out by re-dissolving the residue in chloroform followed by filtration. Then the supernatant was evaporated by rotary evaporation and stored under −20 °C until used. The yielded product was subjected to the detection of MALDI-TOF mass spectrometry (MALDI-TOF MS).
Preparation and characterization of liposomes
Liposomes were prepared as follows. For the preparation of [D]-H6L9 labeled liposomes (D-Lip), cholesterol, SPC, DSPE-PEG2000, and DSPE-PEG2000-[D]-H6L9 with a molar ratio of 33:(63 − X):4:X (X represented the amount of DSPE-PEG2000-[D]-H6L9 in the liposomes, and in our case, they were 3%, 4%, 5%, 6%, and 8%, respectively, in searching for the optimal formulation) were dissolved in the mixture of chloroform and methanol. The organic solvents were removed by rotary evaporation, and a thin lipid film was formed and further dried under vacuum overnight for the removal of remaining solvents. The lipid film was hydrated with appropriate volume of 5% glucose solution and incubated under 37 °C with shaking for 30 min. The obtained liposomes were further dispersed by being intermittently sonicated by a probe sonicator. The PEGylated liposomes (PEG-Lip) were prepared with DSPE-PEG2000-[D]-H6L9 replaced by DSPE-PEG2000. Similarly, appropriate amount of CFPE, DiR, and DiD was added to the lipid solution, respectively, to form the fluorescently labeled liposomes.
The mean particle sizes and zeta potentials of D-Lip and PEG-Lip were measured by Malvern Zetasizer Nano ZS90 instrument (Malvern Instruments Ltd., Malvern, UK). In order to characterize the serum stability of liposomes, variations in turbidity were measured with a microplate reader (Thermo Scientific Varioskan Flash, Waltham, MA). About 100 μL liposomes were mixed with 100 μL fetal bovine serum (FBS) under 37 °C with gentle oscillation, and turbidity was read at each predetermined time points.
Cell line and cell culture
C26 cells (murine colon tumor cells) were maintained in RPMI-1640 medium supplemented with 10% FBS, 100 U/mL streptomycin, and 100 U/mL penicillin at 37 °C in a humidified 5% CO2 atmosphere.
Cell viability assay
About 4 × 103 C26 cells were planted into each well of a 96-well plate. After the cells were attached to the plate, cell culture medium was evacuated and liposome-containing medium was applied to each well. Twenty-four hours later, cytotoxicity was evaluated with MTT assay, with the absorbance read at 570 nm. Non-treated cells were used as controls. All the measurements were repeated in triplicates.
In vitro cellular uptake assay
For the quantitative study, C26 cells were seeded at a density of 1 × 105 cells/well into 6-well plates and allowed to grow for 24 h. CFPE-labeled liposomes were added to each well with fresh cell culture medium, and liposome-free culture medium was considered as the control. Two hours after incubation under 37 °C, cells were washed with cold PBS for three times and then trypsinized and resuspended in 0.4 mL PBS. The fluorescent intensity of cells was measured by a by a flow cytometer (Cytomics™ FC 500, Beckman Coulter, Miami, FL) with the excitation wavelength at 495 nm and the emission wavelength at 515 nm. Ten thousand events were recorded for each sample.
For qualitative evaluation of cellular uptake, C26 cells were seeded at a density of 2 × 104 cells/well on gelatin-coated cover slip. After 24 h, CFPE-labeled liposomes were applied to each well and the incubation continued for another 2 h. Cells were then washed with cold PBS for three times and fixed with 4% paraformaldehyde at room temperature for 15 min, and the nuclei were stained with DAPI for 5 min. Finally, cells were subjected to observation by a laser scanning confocal microscope (TCS SP5 AOBS confocal microscopy system, Leica, Wetzlar, Germany).
Tumor spheroid uptake assay
C26 cells were trysinized and resuspended in 1640 cell culture medium at a density of 2 × 103 cells/100 μL and were added to2% agarose-coated 96-well plate. The formation of C26 tumor spheroids was monitored by optical microscope. Then the spheroids were incubated with CFPE-labeled liposomes for 2 h, and gently washed with cold PBS by pipet. Then they were fixed in 4% paraformaldehyde under room temperature and were imaged under confocal microscope (TCS SP5 AOBS confocal microscopy system, Leica, Wetzlar, Germany).
Effect of different inhibitory factors on cellular uptake
C26 cells were first treated with amiloride (1.48 mg/mL), chlorpromazine (20 μg/mL), genistein (56 μg/mL), poly-lysine (800 μg/mL), and free [D]-H6L9 peptide, respectively, for 30 min under 37 °C to study the effect of different inhibitors on cellular uptake. Then fresh cell culture media containing CFPE labeled liposomes were added and the incubation lasted for another 2 h under pH 6.3. For the exploration of temperature on cellular uptake, cells were incubated under 4 °C for 2 h. Then cells were harvested and treated as described in section Cell viability assay and the fluorescence intensity was determined.
Subcellular localization assay
C26 cells were seeded onto gelatin-coated cover slip at a density of 2 × 104 cells/well. Cells were applied with CFPE-liposome containing cell culture medium and incubated for 12 h. By the end of incubation, lysotracker red (50 nM) was added into each well and incubated for 30 min. Cells were then washed rapidly with ice cold PBS and fixed with 4% paraformaldehyde at room temperature for 15 min followed by nuclei were stained with DAPI for 5 min, and were prepared for visualization under confocal microscope (FV1000, Olympus, Center Valley, PA).
Tumor model and biodistribution study
About 6-week to 8-week old Balb/C mice were inoculated with 1 × 106 cells subcutaneously into the left flank. Tumors were allowed to grow to an average volume of 50–100 mm3 (tumor volume = length × width2 × 0.52) for the biodistrubition study.
Tumor-bearing Balb/C mice were randomly divided into different groups with three mice each. For the in vivo and ex vivo imaging, DiR-loaded liposomes were intravenously injected at a dose of 200 μg DiR/kg. Twenty-four hours after injection, mice were imaged with IVI® Spectrum system (Caliper, Hopkington, MA). Then the mice executed with cervical dislocation and vital organs and tissues (including hearts, livers, spleens, lungs, kidneys, and tumors) were taken out and also imaged. For the quantitative evaluation of tumor uptake of liposomes and the cyro-section observation, mice were injected with DiD-labeled liposomes at a dose of 500 μg DiR/kg. Twenty-four hours later, mice were sacrificed with heart perfusion of saline. Tumors were harvested and cyro-sectioned at a thickness of 10 μm, and sections were treated with 4% paraformaldehyde and DAPI, and were imaged under confocal microscope. For the quantitative measurement of tumor uptake of liposomes, tumors were excised and cut into small pieces and treated with dissociation solution (collagenase type IV [1 mg/mL] and DNase I [30 mg/mL] in PBS) for 1 h under 37 °C. Cell suspension was passed through a 70-μm mesh and washed with cold PBS for three times and was subsequently resuspended in PBS for flow cytometry measurement.
Statistical analysis
Data were presented as mean ± SD. Statistical differences were determined by the Student t test. *p Value <0.05 was considered as significantly significant.
Results
Preparation and characterization of liposomes
The synthesis of DSPE-PEG2000-[D]-H6L9 was confirmed by MALDI-TOF mass spectrometry (Figure S1), with the observed molecular weight at 4844 Da, which was close to theoretical molecular weight at 4903 Da. It could be observed from that the sizes of PEG-Lip and D-Lip under both pH 7.4 and 6.3 were around 100 nm, which was suitable for the follow-up in vitro and in vivo assays. There was an evident conversion in zeta potential from negative (−2.2 ± 0.8 mV) to positive (9.2 ± 1.1 mV), while the same transformation in zeta potential did not happen to PEG-Lip. This was clearly brought by the introduction of peptide [D]-H6L9. As for the turbidity monitoring (), D-Lip with the amount of DSPE-PEG2000-[D]-H6L9 ranging from 3% to 6% all exhibited turbidity above 90%, which showed that there was no formation of aggregation in the presence of serum, evidencing the serum stability of D-Lip. When the amount of DSPE-PEG2000-[D]-H6L9 increased to 8%, the turbidity started to decline below 90% from 4 h to 24 h, which was probably due to the excessive presence of [D]-H6L9 that was detrimental to the serum stability of liposomes, and this required further verification in the future.
Figure 1. (A) The serum turbidity monitoring after mixing liposomes with serum of the same volume and incubated under 37 °C. (B) The cellular uptake of D-Lip with different amount of DSPE-PEG2000-[D]-H6L9 in the lipid constitution. The horizontal ordinate suggested the ratio of DSPE-PEG2000-[D]-H6L9 in total lipid.
![Figure 1. (A) The serum turbidity monitoring after mixing liposomes with serum of the same volume and incubated under 37 °C. (B) The cellular uptake of D-Lip with different amount of DSPE-PEG2000-[D]-H6L9 in the lipid constitution. The horizontal ordinate suggested the ratio of DSPE-PEG2000-[D]-H6L9 in total lipid.](/cms/asset/a2c8038e-8508-4974-9bb2-fa20f8ddf248/idrd_a_1003665_f0001_c.jpg)
Table 1. The size and the zeta potential measurement of PEG-Lip and D-Lip under different pH conditions when the amount of the DSPE-PEG2000-[D]-H6L9 was 6% in the total lipids.
In vitro cellular uptake assay and cell viability assay
For the assays at pH 6.3, the cells were pre-adapted to culture media of pH 6.3. First, C26 cells were dosed with D-Lip with the amount of DSPE-PEG2000-[D]-H6L9 ranging from 3% to 8% in order to study the effect of the density of DSPE-PEG2000-[D]-H6L9 on cellular uptake. Results showed that the cellular uptake of D-Lip under pH 6.3 was in a [D]-H6L9 peptide density-dependent manner (); cellular uptake of D-Lip increased with the increased density of DSPE-PEG2000-[D]-H6L9 in the lipid formulation. However, the cellular uptake under pH 7.4 remained quite low for all the groups. Despite the declined serum stability of D-Lip with 8% DSPE-PEG2000-[D]-H6L9 (), its cellular uptake remained the highest, showing the strong potency of [D]-H6L9 in transferring the liposomes into cells under pH 6.3. Taking the results of serum stability into consideration, 6% of [D]-H6L9 peptide was opted for the preparation of D-Lip in the following assay.
PEG-Lip under both pH 7.4 and 6.3 showed no preference in cellular uptake; when it comes to D-Lip, although the cellular uptake efficiency under pH was quite close to PEG-Lip, it surprisingly augmented under pH 6.3 (increased by 12.1 ± 1.3-folds). As seen from , the conversion of zeta potential into positive charge could result in enhanced interaction between D-Lip and cellular membrane, facilitating the cellular entry of liposomes. MTT assay showed that under both low (as low as 50 μg/mL) and high (as high as 1 mg/mL) lipid concentrations, blank D-Lip exhibited minimal cytotoxicity against C26 cells, suggesting that it was safe to apply liposomes to cells under these concentrations ().
Figure 2. Parts (A) and (B) represent the cellular uptake on C26 cells of PEG-Lip and D-Lip under pH 7.4 and pH 6.3 determined by confocal microscopy and flow cytometry. (C) The cytotoxicity of blank D-Lip of different concentrations under both pH 7.4 and 6.3. The horizontal ordinate indicated the final total lipid concentration of liposomes in culture medium.
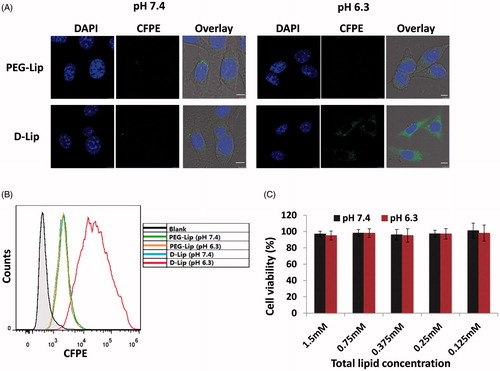
Tumor spheroid uptake
Tumor spheroids were usually used to mirror the therapeutically relevant pathophysiological gradients of the in vivo tumors () and were more reliable to predict the effect of certain delivery vectors on tumors. As seen from , similar to the cellular uptake results in , tumor spheroid uptake of D-Lip under pH 6.3 was also significantly higher than all the other groups.
Subcellular localization and cellular uptake mechanism assay
After 12 of internalization of D-Lip, it could be observed from that there was very little overlapping in fluorescence of CFPE-labeled liposomes with lysosomes, signifying the ability of D-Lip to escape or evade the lysosomes and successfully got into cytosol. As for the effect of different inhibitory factors on cellular uptake, first of all, low temperature exerted significant decrease in cellular uptake of D-Lip (to 63.54 ± 2.73% of the normal level), meaning that the cellular uptake of D-Lip was energy dependent. The marked inhibition by poly-lysine (18.99 ± 1.42% of the normal level) certified that D-Lip was mediated into cells after strong interaction of [D]-H6L9 on the surface of liposomes with cellular membranes. Amiloride, chlorpromazine, and genistein were all the inhibitors on macropinocytosis, clathrin-dependent pathway and caveolin-dependent pathway, respectively, and they all could inhibit the cellular uptake of D-Lip (78.27 ± 6.19%, 77.39 ± 2.31%, and 45.80 ± 1.07% of the normal level, respectively, ). This suggested that probably more than one pathway was implicated in the cellular uptake of D-Lip. On the contrary, the presence of free [D]-H6L9 peptide did not inhibit cellular uptake, showing that there should be no specific receptors on C26 cells for [D]-H6L9.
Figure 4. (A) The subcellular localization on C26 cells of CFPE-labeled liposomes (A2 and A6) and lysosomes (A3 and A7). Nuclei were stained by DAPI (A1 and A5). Scale bars represented 10 μm. (B) Cellular uptake of CFPE-labeled D-Lip on C26 cells inhibited by different factors. *indicates the significance between group Normal and all the other groups.
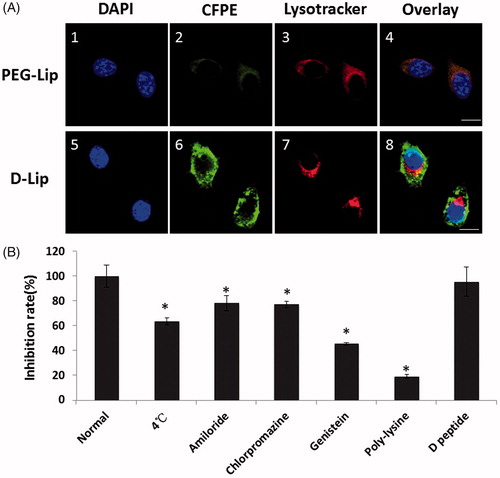
Biodistribution study
To evaluate the capacity of systemic delivery of D-Lip and PEG-Lip to tumors, C26 tumor-bearing mice models were established. In , it showed that D-Lip and PEG-Lip could both reach the tumor with effect, and the ex vivo images showed that their biodistribution in different organs and tumors was quite similar to each other. This should be contributed by the PEGylation of liposomes. However, after we collected tumors after heart perfusion and subjected them to both cofocal microscope observation and flow cytometry determination, it was found that the cellular internalization of D-Lip was significantly higher than that of PEG-Lip (), which should be owing to the enhanced cellular uptake of D-Lip in the acidified environment in tumors.
Figure 5. Biodistribution assay of D-Lip on C26 tumor-bearing Balb/C mice. Parts (A) and (B) display the in vivo and ex vivo fluorescent biodistribution of DiR-loaded liposomes; the black arrows in (A) indicated the location of the C26 tumor. (C) The quantitative determination of tumor uptake of DiD-loaded liposomes is shown, *indicating the significance between PEG-Lip and D-Lip and (D) the confocal images of tumor frozen sections are shown. Bars represent 50 μm.
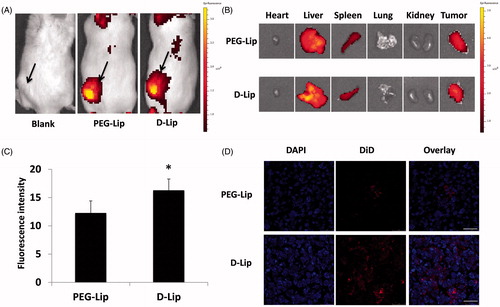
Discussion
Efforts have been devoted into the development of nano-sized vehicles carrying AMPs for the therapy of infections for their antimicrobial or antitumor effect (Kazemzadeh-Narbat et al., Citation2010; Almaaytah et al., Citation2012; Urbán et al., Citation2012; Kazemzadeh-Narbat et al., Citation2013). However, not enough endeavors have been made in establishing AMPs-mediated drug delivery system. Actually, these peptides also have great potential in drug delivery due to their own property. For example, melittin belonged to the family of AMPs and possessed cytolytic ability (Santo et al., Citation2013). Hou et al. (Citation2013) utilized melittin’s pore-forming ability in membrane bilayers to deliver siRNA, and results showed that it significantly increased transfection efficiency even in the presence of serum proteins. In order to lower the cytotoxicity of melittin, truncated peptides derived from melittin were designed, which could be considered as potential enhancers to improve gene transfection efficiency (Tan et al., Citation2012). Therefore, in our case, we selected the antimicrobial peptide [D]-H6L9 based on its pH-responsiveness to construct a novel pH-responsive liposomal delivery system. As could be concluded from the results in size and zeta potential measurements, the charge conversion from negative (−2.2 ± 0.8 mV) to positive (9.2 ± 1.1 mV) was clearly brought by histidines in the peptide sequence, while this kind of charge conversion did not happen for PEG-Lip. The imidazole ring in histidine was bound to protonate under acidified environment with pH 6.3 (mimicking the in vivo tumor environment, which was between pH 6.0 and 6.7) (Helmlinger et al., Citation1997; Bandekar et al., Citation2013), transforming the zeta potential into positive, which exerted major influence in the following study.
The purpose of serum stability as well as applying cells with D-Lip modified with different amount of [D]-H6L9 peptide was to screen for the optimal amount for DSPE-PEG2000-[D]-H6L9. In our study, we found that the more DSPE-PEG2000-[D]-H6L9 added into the formulation, the higher the cellular uptake would be; however, the large amount of DSPE-PEG2000-[D]-H6L9 was likely to influence the integrity and the stability of liposomes in serum, as implied by the elevated turbidity of D-Lip modified with 8% DSPE-PEG2000-[D]-H6L9. Therefore, 6% DSPE-PEG2000-[D]-H6L9 was determined for the formulation.
In the subsequent cellular and tumor spheroid uptake study, the uptake of D-Lip under pH 6.3 significantly enhanced compared with pH 7.4, as proved by both flow cytometry and confocal microscopy. Intriguingly, MTT assay showed almost no cytotoxicity of D-Lip under both pH 7.4 and pH 6.3 on C26 cells within 24 h. Although, according to Makovitzki et al. (Citation2009) peptide [D]-H6L9 displayed certain cytotoxicity over several cell lines, in our research, D-Lip almost showed no cytotoxicity over C26 cells. The reason might be that the concentration we adopted for peptide [D]-H6L9 was not high enough (about 5 μM for in vitro cellular assays). Besides, the PEG chains on the surface of liposomes were uneven in length due to the polymer nature of PEG (with an average molecular weight of 2000 Da), thus some of the [D]-H6L9 peptide might be unavoidably masked, further reducing the exposed [D]-H6L9 peptide to outside environment, guaranteeing that the amount of [D]-H6L9 was with a rather safe but effective range, which could permeate cells to attain improved cellular internalization under pH 6.3 without seriously damaging cells.
As for the subcellular localization of liposomes with lysosomes, it could be observed from that the co-localization of D-Lip with lysosomes was very limited. Moreover, the cellular uptake pathways of D-Lip on C26 were multi-faceted, as amiloride (inhibiting macropinocytosis), chlorpromazine (inhibiting clathrin-dependent pathway), and genistein (inhibiting caveolin-dependent pathway) all showed inhibitory effect to a different extent over the uptake of D-Lip. Some researchers said that clathin-mediated endocytosis would lead to lysosomal degradation, meanwhile macropinocytosis and caveolae-mediated endocytosis could avert this kind of lysosome fusion and ultimate degradation (Dreaden & El-Sayed, Citation2012; Xiang et al., Citation2012; Xu et al., Citation2013). If so, combing the results from , it was reasonable to conclude that since D-Lip was found to be taken up by C26 cells through multiple mechanisms, its lack of co-localization with lysosomes was caused by the uptake through macropinocytosis and caveolae-mediated endocytosis. Nevertheless, some other reports suggested otherwise (Xiang et al., Citation2012), arguing that the internalization process was quite complicated and the integration of endocytosed vesicles with lysosomes could take place not just in clathin-mediated endocytosis. Thereby, further study will be conducted in the future to obtain in-depth understanding of the mechanism through which D-Lip was taken up by cells.
We also performed in vivo fluorescent biodistribution study to investigate the in vivo behavior of D-Lip and PEG-Lip. The biodistribution patterns of them were quite similar to each other, and they could both reach tumor effectively as shown in . This should be owing to the PEGylation of liposomes, which avoided the recognition and uptake by the RES system (Yang et al., Citation2011; Perry et al., Citation2012; Zhang et al., Citation2012). Also, this should be owing to the anionic surface charge of D-Lip under pH 7.4, which meant that it could maintain negatively charged during blood circulation, repulsing the reaction of the serum protein in the blood. However, after tumors were dissected and measured, it could be seen that tumor cells would preferentially take up D-Lip in vivo as demonstrated in . This should be due to the fact that under the acidic environment of tumors, the surface charge of D-Lip could be turned to positive, so the tumor cellular internalization of D-Lip could be promoted compared with that of PEG-Lip. Being able to be timely taken up by tumor cells would be of utmost importance, because insufficient tumor cellular internalization would inevitably lead to loss of liposomes in tumor area since under such circumstances liposomes would get back into blood vessels (Manzoor et al., Citation2012), further impeding a better treatment outcome.
Some researchers suggested that AMPs and CPPs shared some similarities in their structures and mechanisms of action (Henriques et al., Citation2006; Splith & Neundorf, Citation2011). In this sense, AMPs should be investigated in establishing AMP-directed drug delivery system as well. As a matter of fact, some CPP-based transfection effect was often limited due to the sequestration of lysosomes (Veldhoen et al., Citation2006; Salomone et al., Citation2012). On the contrary, AMPs with strong membrane-destabilizing property could be counted as a novel drug delivery alternative (Hou et al., Citation2013; Huang et al., Citation2013). This potential for AMPs, and in our research the pH-responsive peptide [D]-H6L9, has not yet been fully exploited by now. We now show that liposomes modified with peptide [D]-H6L9 could prove itself to be a rather promising drug delivery system, and hope this work could be of interest for researchers in developing AMP-mediated drug delivery systems as well as pH-responsive tumor-targeted delivery systems.
Conclusion
In this study, we modified one pH-responsive anti-microbial peptide, [D]-H6L9 onto the surface of liposomes (D-Lip), thus establishing the liposomal delivery system with pH-responsiveness. Results indicated that the cellular uptake and the tumor uptake efficiency were both significantly promoted under pH 6.3 compared with pH 7.4, and it did not exhibit evident cytotoxicity towards C26 cells under the concentrations investigated. The lysosome staining assay suggested that it could escape the confinement of lysosomes successfully in 12 h, and its cellular uptake was mediated by multiple pathways. In vivo biodistribution assay also showed that D-Lip could reach tumor effectively and its tumor uptake was much higher than PEG-Lip, signifying that it could be preferentially be taken up by tumor cells under acidic tumor environment. D-Lip proved itself to be an excellent vehicle for pH-responsive tumor delivery.
Supplementary material available online
Supplementary Figure S1.
Supplemental Material.pdf
Download PDF (23.4 KB)Declaration of interest
This work was funded by the National Basic Research Program of China (973 Program, 2013CB932504) and the National Natural Science Foundation of China (81373337).
References
- Almaaytah A, Zhou M, Wang L, et al. (2012). Antimicrobial/cytolytic peptides from the venom of the north african scorpion, androctonus amoreuxi: biochemical and functional characterization of natural peptides and a single site-substituted analog. Peptides 35:291–9
- Attoub S, Mechkarska M, Sonnevend A, et al. (2013). Esculentin-2CHa: a host-defense peptide with differential cytotoxicity against bacteria, erythrocytes and tumor cells. Peptides 39:95–102
- Bandekar A, Zhu C, Gomez A, et al. (2013). Masking and triggered unmasking of targeting ligands on liposomal chemotherapy selectively suppress tumor growth in vivo. Mol Pharm 10:152–60
- Brown KL, Hancock RE. (2006). Cationic host defense (antimicrobial) peptides. Curr Opin Immunol 18:24–30
- Chen L, Tu Z, Voloshchuk N, et al. (2012). Lytic peptides with improved stability and selectivity designed for cancer treatment. J Pharm Sci 101:1508–17
- Di Francesco A, Favaroni A, Donati M. (2013). Host defense peptides: general overview and an update on their activity against chlamydia spp. Expert Rev Anti-Infect Therapy 11:1215–24
- Dreaden EC, El-Sayed MA. (2012). Emerging nanomaterials for targeting subcellular organelles. Acc Chem Res 45:1854–65
- Fjell CD, Hiss JA, Hancock RE, Schneider G. (2011). Designing antimicrobial peptides: form follows function. Nature Rev Drug Discov 11:37–51
- Guaní-Guerra E, Santos-Mendoza T, Lugo-Reyes SO, et al. (2010). Antimicrobial peptides: general overview and clinical implications in human health and disease. Clin Immunol 135:1–11
- Hancock RE, Sahl HG. (2006). Antimicrobial and host-defense peptides as new anti-infective therapeutic strategies. Nature Biotechnol 24:1551–7
- Helmlinger G, Yuan F, Dellian M, et al. (1997). Interstitial pH and pO2 gradients in solid tumors in vivo: high-resolution measurements reveal a lack of correlation. Nature Med 3:177–82
- Henriques ST, Melo MN, Castanho MA. (2006). Cell-penetrating peptides and antimicrobial peptides: how different are they? Biochem J 399:1–7
- Hou KK, Pan H, Lanza GM, Wickline SA. (2013). Melittin derived peptides for nanoparticle based siRNA transfection. Biomaterials 34:3110–19
- Huang C, Jin H, Qian Y, et al. (2013). Hybrid melittin cytolytic peptide-driven ultrasmall lipid nanoparticles block melanoma growth in vivo. ACS Nano 7:5791–5800
- Jiang T, Zhang Z, Zhang Y, et al. (2012). Dual-functional liposomes based on pH-responsive cell-penetrating peptide and hyaluronic acid for tumor-targeted anticancer drug delivery. Biomaterials 33:9246–58
- Kazemzadeh-Narbat M, Kindrachuk J, Duan K, et al. (2010). Antimicrobial peptides on calcium phosphate-coated titanium for the prevention of implant-associated infections. Biomaterials 31:9519–26
- Kazemzadeh-Narbat M, Lai BF, Ding C, et al. (2013). Multilayered coating on titanium for controlled release of antimicrobial peptides for the prevention of implant-associated infections. Biomaterials 34:5969–77
- Mangoni ML. (2011). Host-defense peptides: from biology to therapeutic strategies. Cell Mol Life Sci 68:2157–9
- Manzoor AA, Lindner LH, Landon CD, et al. (2012). Overcoming limitations in nanoparticle drug delivery: triggered, intravascular release to improve drug penetration into tumors. Cancer Res 72:5566–75
- Makovitzki A, Fink A, Shai Y. (2009). Suppression of human solid tumor growth in mice by intratumor and systemic inoculation of histidine-rich and pH-dependent host defense-like lytic peptides. Cancer Res 69:3458–63
- Nguyen LT, Haney EF, Vogel HJ. (2011). The expanding scope of antimicrobial peptide structures and their modes of action. Trends Biotechnol 29:464–72
- Papo N, Seger D, Makovitzki A, et al. (2006). Inhibition of tumor growth and elimination of multiple metastases in human prostate and breast xenografts by systemic inoculation of a host defense-like lytic peptide. Cancer Res 66:5371–8
- Papo N, Shai Y. (2005). Host defense peptides as new weapons in cancer treatment. Cell Mol Life Sci 62:784–90
- Perry JL, Reuter KG, Kai MP, et al. (2012). PEGylated PRINT nanoparticles: the impact of PEG density on protein binding, macrophage association, biodistribution, and pharmacokinetics. Nano Lett 12:5304–10
- Reddy KV, Yedery RD, Aranha C. (2004). Antimicrobial peptides: premises and promises. Int J Antimicrobial Agents 24:536–47
- Riedl S, Zweytick D, Lohner K. (2011). Membrane-active host defense peptides – challenges and perspectives for the development of novel anticancer drugs. Chem Phys Lipids 164:766–81
- Salomone F, Cardarelli F, Di Luca M, et al. (2012). A novel chimeric cell-penetrating peptide with membrane-disruptive properties for efficient endosomal escape. J Control Release 163:293–303
- Santo KP, Irudayam SJ, Berkowitz ML. (2013). Melittin creates transient pores in a lipid bilayer: results from computer simulations. J Phys Chem B 117:5031–42
- Splith K, Neundorf I. (2011). Antimicrobial peptides with cell-penetrating peptide properties and vice versa. Eur Biophys J 40:387–97
- Tan YX, Chen C, Wang YL, et al. (2012). Truncated peptides from melittin and its analog with high lytic activity at endosomal pH enhance branched polyethylenimine-mediated gene transfection. J Gene Med 14:241–50
- Tannock IF, Rotin D. (1989). Acid pH in tumors and its potential for therapeutic exploitation. Cancer Res 49:4373–84
- Tian L, Bae YH. (2012). Cancer nanomedicines targeting tumor extracellular pH. Colloids Surf B Biointerfaces 99:116–26
- Tu Z, Volk M, Shah K, et al. (2009). Constructing bioactive peptides with pH-dependent activities. Peptides 30:1523–8
- Urbán P, Valle-Delgado JJ, Moles E, et al. (2012). Nanotools for the delivery of antimicrobial peptides. Cur Drug Targets 13:1158–72
- Veldhoen S, Laufer SD, Trampe A, et al. (2006). Cellular delivery of small interfering RNA by a non-covalently attached cell-penetrating peptide: quantitative analysis of uptake and biological effect. Nucleic Acids Res 4:6561–73
- Xiang S, Tong H, Shi Q, et al. (2012). Uptake mechanisms of non-viral gene delivery. J Control Release 158:371–8
- Xu S, Olenyuk BZ, Okamoto CT, et al. (2013). Targeting receptor-mediated endocytotic pathways with nanoparticles: rationale and advances. Adv Drug Deliv Rev 65:121–38
- Yang K, Wan J, Zhang S, et al. (2011). In vivo pharmacokinetics, long-term biodistribution, and toxicology of PEGylated graphene in mice. ACS Nano 5:516–22
- Yeung AT, Gellatly SL, Hancock RE. (2011). Multifunctional cationic host defense peptides and their clinical applications. Cell Mol Life Sci 68:2161–76
- Zasloff M. (2002). Antimicrobial peptides of multicellular organisms. Nature 415:389–95
- Zhang W, Song J, Zhang B, et al. (2011). Design of acid-activated cell penetrating peptide for delivery of active molecules into cancer cells. Bioconjugate Chem 22:1410–15
- Zhang Y, Satterlee A, Huang L. (2012). In vivo gene delivery by nonviral vectors: overcoming hurdles &quest. Mol Therapy 20:1298–304
- Zhao BX, Zhao Y, Huang Y, et al. (2012). The efficiency of tumor-specific pH-responsive peptide-modified polymeric micelles containing paclitaxel. Biomaterials 33:2508–20