Abstract
Context: Polymeric delivery system is useful in reducing pharmacokinetic limitations viz., poor absorption and rapid elimination associated with clinical use of curcumin. Design of experiment is a precise and cost effective tool useful in analyzing the effect of independent variables and their interaction on the product attributes.
Objective: To evaluate the effect of process variables involved in preparation of curcumin-loaded polycaprolactone (PCL) nanoparticles (CPN).
Materials and methods: In the present experiment, CPNs were prepared by emulsification solvent evaporation technique. The effect of independent variables on the dependent variable was analyzed using design of experiments. Anticancer activity of CPN was studied using Ehrlich ascites carcinoma (EAC) model. In-situ implant was developed using PLGA as polymer.
Results and discussion: The effect of independent variables was studied in two stages. First, the effect of drug–polymer ratio, homogenization speed and surfactant concentration on size was studied using factorial design. The interaction of homogenization speed with homogenization time on mean particle size of CPN was then evaluated using central composite design. In the second stage, the effect of these variables (under the conditions optimized for producing particles <500 nm) on percentage drug encapsulation was evaluated using factorial design. CPN prepared under optimized conditions were able to control the development of EAC in Swiss albino mice and enhanced their survival time. PLGA based in-situ implant containing CPN prepared under optimized conditions showed sustained drug release.
Conclusion: This implant could be further evaluated for pharmacological activities.
Introduction
Curcumin is a “pleiotropic” molecule. Its clinical application is limited due to poor water solubility. Rapid metabolism and elimination further complicates in-vivo usage of curcumin. The bioavailability and site targeting could be enhanced if the curcumin is delivered using suitable biodegradable polymers (Anand et al., Citation2007; Yallapu et al., Citation2012). Previous studies show that curcumin loaded in polymers such as poly lactic-co-glycolic acid (PLGA) (Anand et al., Citation2009) and polycaprolactone (PCL) (Raveendran et al., Citation2013) are safe, pharmaceutically stable and effective in treating cancer. Half-life and efficacy are further improved by delivering curcumin in nanoformulation (Yallapu et al., Citation2013). Most of the studies on polymeric delivery of curcumin focuses on therapeutic application of curcumin encapsulated in polymers with less focus on product development. A consistent, reproducible result would be obtained if a product is prepared using quality by design (QbD) approach. These statistical tools help in preparing products with high quality attributes on a consistent base.
Design of experiments (DoE) is a valuable tool utilized in QbD approach for developing product (Lionberger et al., Citation2008). Effect of various process variables on the outcome can be effectively analyzed using DoE. In DoE, the experiments are designed such that the independent variables are concurrently changed and the combined effect on the outcome is studied. These kinds of designs are therefore useful in assessing the significance of interaction on the dependent variables (Anderson & Whitcomb, Citation2000). This will help in reducing the focus on unclassified parameters that are not critical for achieving the desired outcome and focusing more on critical parameters required to achieve the target (Lionberger et al., Citation2008). Although traditional trials that test single variability at a time is easy to understand, DoE is more precise and allows estimation of “experimental error” (Telford, Citation2007). This will help in “building the quality into the product rather than testing at the end” (Guideline, Citation2009). Few studies had reported the effect of process variables viz., drug–polymer ratio and PVA concentration on mean particle size and percentage drug encapsulation using one-factor-at-a-time (OFAT) design (Lee et al., Citation1999). But it is still required to analyze these variables using DoE as it will help in understanding the level of significance of each variable and the effect of interaction of the variables on the outcome. Further, it will help in deciding the conditions that will meet the objective of the new set of experiments. In the present work, experimental design tool was used for developing and evaluating curcumin-loaded PCL nanoparticles. The polymeric nanoparticles were tested for anticancer activity in mice using Ehrlich ascites carcinoma (EAC) model. Curcumin-loaded PCL nanoparticles (CPN) developed under optimized conditions were used in developing PLGA based in-situ implant. In-vitro release of the drug release from the implant was further studied.
Materials and methods
Chemicals
PCL (molecular weight 70 000–90 000 by GPC) and polyvinyl alcohol (cold water soluble) were procured from Sigma, St. Louis, MO, USA. PLGA 50:50 (Resomer®, 503H) was purchased from Boehringer Ingelheim Pharma Bmbh & Co., Ingelheim, Germany. Curcumin (95% purity) was purchased from Venbiotech Private Limited, Chennai, India. Analytical grade solvents were purchased from Merck, Mumbai, India.
Methods
Design of experiment
The reliance of percentage drug encapsulation, size and zeta potential on process variables involved in preparation of curcumin-loaded PCL nanoparticles was studied using DoE. The experiments were carried out in two stages. In the first stage, conditions for producing nanoparticles were identified using full factorial design and central composite design (CCD). Under these, the dependency of size and zeta potential on drug–polymer (curcumin-PCL) ratio (D/P ratio), homogenization speed and polyvinyl alcohol (PVA) concentration was first analyzed using full factorial design (). The design consisted of eight trials with zero center points and single block. The independent variable exhibiting the maximum influence on the outcome, i.e. mean particle size was selected and analyzed using CCD for their interaction with homogenization speed to produce nanoparticles. CCD consisted of 13 runs with four cube points, five center points in a cube, four axial points and zero center points in axial. The alpha value was maintained at 1.41 ().
Table 1. Full factorial design showing the effect of process variables on size.
Table 2. Central composite design to study the effect of interaction between homogenization speed and homogenization time on mean particle size.
In the second stage, the effect of the independent variables on percentage drug encapsulation was studied using factorial design (). The levels of these variables were selected based on the results obtained from the above factorial and CCD studies. The levels were identified such that particles of less than 500 nm could be achieved under the conditions employed. Minitab-16 (trial version; Minitab, Coventry, UK) and Design Expert (V.8.0.7.1; Stat-Ease, MN, USA) were used throughout the experiments for creating design matrix and analysis of the outcome.
Table 3. Factorial design to study the effect of process variables on percentage drug encapsulation, size and zeta potential.
Preparation of curcumin-loaded polycaprolactone nanoparticles
CPNs' were prepared by emulsion technique. Curcumin (20 mg) and PCL () were dissolved in 20 ml of dichloro methane (DCM). The drug–polymer mixture was added drop wise to 20 ml of PVA () maintained under a high speed homogenization ( during factorial design and during CCD) (Polytron Mixer, Kinematica, Luzen, Switzerland) and allowed for emulsification for 15 min ( indicates the homogenization time used during CCD). At the end of homogenization, the mixture was kept under shaking overnight in an orbital shaking incubator at 150 rpm and 30 °C to allow complete evaporation of DCM. After overnight incubation, emulsion obtained was first centrifuged at low rpm (2500 rpm for 3 min) to remove free drug and coarse particles. The resulting supernatant was then centrifuged at high speed (22 000 rpm for 60 min). The pellet was suspended in ultrapure water (Milli-Q, Millipore, Bangalore, India) and the particle size was analyzed using quasi-elastic light scattering (Malvern zeta sizer, Malvern Instruments, Malvern, UK). The supernatant obtained after low speed centrifugation was freeze dried (Ilshin Corp., Gyeonggi, South Korea) at −45 °C for 28–30 h. The amount of curcumin in the resulting cake was estimated to calculate entrapment efficiency. Freeze dried cake (CPN) was dissolved in DCM. The solvent was removed by evaporating the mixture at 40 °C. The free curcumin was dissolved in methanol and the absorbance was measured by UV spectrophotometer at 422 nm (Biospec-1601, Shimadzu, Japan). The amount of curcumin was then estimated by comparing with a standard curve.
Drug release
Amount of drug released from the formulation was studied using vial method (Khalil et al., Citation2013). CPNs were prepared using optimized conditions and freeze dried. Weighed quantity of freeze dried CPN (containing approximately 2.5 mg of curcumin) was transferred into a vial and 2 ml of phosphate buffer saline (PBS, pH 7.4) was added. The vials were incubated on an orbital shaker incubator (Scigenics Ltd, Chennai, India) at 37 °C with 120 rpm. Vials were removed at the required time interval and the content was transferred into a centrifuge tubes. The samples were centrifuged at 22 000 rpm for 60 min. At the end of centrifugation, the supernatant was estimated for amount of curcumin released. The pellet was redispersed in a fresh 2 ml PBS and transferred back into the vial and incubated on the orbital shaker incubator for next set of readings.
Animal studies
The anticancer potential of the CPN was studied using EAC model. All the animals were maintained and handled following the guidelines of Committee for Purpose of Control and Supervision of Experiments on Animals (CPCSEA) after getting approval (IAEC/KMC/66/2013) to carry out the research work on animals. All the Swiss albino mice used in the experiments were 8–10 weeks old weighing 23–32 g selected from the group of inbred animals maintained under the controlled environmental conditions. Animals were fed with standard rat food and water ad libitum. On the day of injection (Day 0), each mouse was injected intraperitoneally with viable EAC cells (2.5 × 106 cells) except sham control. On day 1, the animals were randomized into four groups with each group containing eight animals (four animals per cage) viz., group 1 (normal control), group 2 (EAC control without any intervention), group 3 (plain curcumin) and group 4 (CPN). Group 3 and 4 received three doses of drug by intra-peritoneal route (day 1, day 4 and day 7). Plain curcumin (triturated with 0.2% carboxy methyl cellulose) was given at 40 mg/kg body weight (Pal et al., Citation2001). The formulation was injected such that the total amount of the drug in the formulation was 20 mg/kg body weight of animal (Ranjan et al., Citation2013). Tumor development in each animal was monitored by recording the weight of each animal from day 1 till day 14. Changes in body weight of all the groups were recorded. The changes in the body weight of the test animals were compared with the EAC control to understand the effectiveness of the formulation.
Blood was withdrawn by retro orbital plexus puncture from each group of animals and was used for analyzing RBC, WBC and hemoglobin content using automated hematology analyzer. Four animals from each group were sacrificed at the end of 14 d. The peritoneal fluid collected from the sacrificed animals was used for estimating the tumor volume and tumor weight. The remaining animals were monitored for 30 d to calculate mean survival time (MST). Data were analyzed and represented as mean ± SEM using one-way analysis of variance followed by Tukey's post hoc test (GraphPad Prism 5.00, Instat Software, San Diego, CA, trial version). A value of p < 0.05 was considered to be significant (Koganti et al., Citation2013).
PLGA based in-situ implant
After identifying the optimum conditions for preparing CPN, polymer based in-situ implant containing CPN was developed and studied. PLGA (50:50) was used as the polymer for developing in-situ implant. Acetone served as a model solvent. The required amount of PLGA (50:50) to prepare 25% (w/v) polymer solution was dissolved in acetone at room temperature. CPN was dispersed in the polymer solution. This polymer solution containing CPN was slowly injected into 25 ml of phosphate buffer (pH 7.4) taken in a glass vial. This resulted in formation of implant. Samples were analyzed for 72 h. Each time an aliquot of 1-ml dissolution sample was withdrawn periodically and replaced with an equal volume of fresh buffer. The amount of the drug released from this implant under the conditions used (waster bath shaker at 150 rpm, 37 °C) was analyzed by measuring the absorbance at 422 nm (Biospec-1601, Shimadzu, Japan).
Results and discussion
Evaluation of effect of process variables on preparation of curcumin-loaded PCL nanoparticles
Factorial studies showed that homogenization speed as an independent variable exhibited maximum influence on mean particle size (). PVA as an individual factor had less influence on the outcome, i.e. mean particle size. However, the outcome was more dependent on the interaction of PVA with other factors. The positive effect of homogenization speed and drug–polymer ratio indicates that these factors could result in higher particle size. The negative effect of PVA indicates that this variable is required to achieve particle of smaller size. The higher percentage contribution of interaction effect, i.e. PVA with other independent variables further confirms that PVA could be involved in stabilization of particles. Lower level of PVA could result in the formation of aggregates thereby resulting in large sized particles. When the level of PVA is maintained at 0.5% w/v, the particle size would be in the range of >700 nm to <400 nm. But if the level of PVA is maintained at 2% w/v, the particle size distribution is narrower, i.e. >480 nm to <360 nm.
Although homogenization speed as an independent variable had the maximum influence, the outcome was more dependent on the combined effect of homogenization speed and PVA concentration than on the individual effects of these independent variables (). This could be due to the tendency of small sized particles to aggregate or settle down due to increase in surface tension when the particle size is reduced to 300–500 nm. The lower homogenization time could also contribute to the need for higher PVA level. At low PVA concentrations sufficiently longer homogenization time would be required as the amount of free PVA in the system will be less. At high PVA level, the nanoparticles are immediately coated with PVA as soon they are formed.
Figure 1. Contour plot showing the effect of process variables analyzed using factorial design on mean particle size. Effect of various levels of homogenization speed and PVA concentration on mean particle size when the level of drug–polymer ratio is maintained at a low level (a) and high level (b).
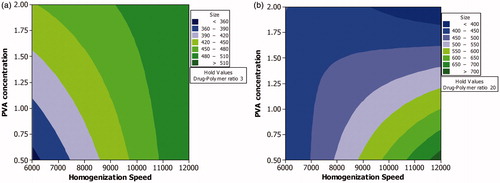
Factorial studies showed that homogenization speed as an individual factor had the maximum effect on particles. The effect of interaction of homogenization speed with homogenization time on particle size was therefore further evaluated using CCD (). The study showed that homogenization time had a significant effect (p-value 0.002) on mean particle size. Decrease with particle size was more pronounced with increasing homogenization time rather than with increasing homogenization speed ().
Figure 2. Surface and overlaid contour plot showing the effect of variables during CCD. (a) Surface plot showing the effect of interaction of homogenization speed and homogenization time on mean particle size. (b) Overlaid contour plot showing level of homogenization speed and homogenization time required to achieve particle size <500 nm.
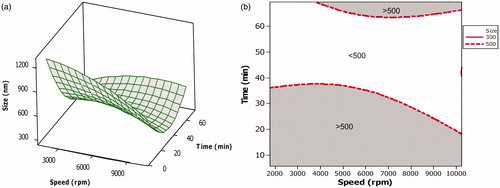
Percentage drug encapsulation
The low and high levels of the independent variables for this study were selected from CCD. Homogenization time was maintained at 30 min. PVA and speed as independent variables had a significant effect on percentage of drug encapsulated with p-values 0.01 and 0.03, respectively. The other variables did not have significant influence on the percentage drug encapsulation. All the independent variables and their interaction with each other had a significant effect on the size (p values of D/P ratio, PVA concentration, homogenization speed, interaction of D/P ratio-PVA, D/P ratio-speed and PVA-speed were < 0.05). Although more than one fourth of the outcome (size) was dependent on the level of individual factor viz., amount of PVA and speed, a higher percentage of the outcome was under the influence of the combined effect of these two individual factors. The negative sign on the effect shows that the interaction of the variables increased the possibility of achieving particles of small size. This once again emphasizes that although speed is the chief factor involved in reducing particle size, PVA is required to stabilize the reduced particle ( and ).
Table 4. The effect of process variables studied under factorial design on percentage drug encapsulation, size and zeta potential.
In case of zeta potential, homogenization speed had a significant effect (p-value 0.02). The effect of interaction of speed with drug–polymer ratio on size was insignificant (p value 0.09). The effect of interaction of speed with PVA and speed-PVA- D/P ratio had a significant effect on the size (p value 0.02) ( and ).
The study showed that a minimum percentage drug encapsulation of 20% could be achieved if the level of all the three independent variables viz., D/P ratio, PVA concentration and homogenization speed are maintained at their highest levels. A decrease in the level of any of these variables will result in a lower percentage of the drug encapsulated. When the speed and drug–polymer ratio are maintained at a high level, PVA needs to be maintained at a high level to achieve particles of small size. At low speed small sized particles are achieved only if the other two variables viz., D/P ratio and PVA concentration are maintained at a low level. At high homogenization speed, if the level of D/P ratio is maintained at a low level, then PVA also needs to be maintained at low level and vice-versa. But a better drug encapsulation is achieved when the level of drug–polymer ratio is maintained high. Therefore, to achieve high drug encapsulation with low particle size, a high concentration of PVA would be required ( and ).
There is a considerable change in surface tension when the particle size falls below 10 µm with concurrent increase in the surface area. A higher amount of surfactant would therefore be required to reduce the high surface tension resulting from increased surface area (Bianco & Marmur, Citation1992). Interaction of homogenization speed and surfactant concentration exhibits a significant effect on drug encapsulation. Complete encapsulation of drug-loaded polymer with surfactant reduces drug leakage into the continuous phase. An optimum amount of surfactant in the formulation is required to achieve complete coating of drug–polymer particles (Kushwaha et al., Citation2013). Apart from surfactant concentration, binding of surfactant to the particle surface is affected by many other factors including homogenization speed and homogenization time. In absence of additional shear force such as homogenization, the coating of surfactant on particles solely depends on the ability of surfactant to get adsorbed on the particles. This depends on the concentration gradient of surfactant in the medium to the particles which acts as the driving force for diffusion. The diffusion from the medium on to the particle surface reduces as the concentration reduces. In presence of high shear stress or low shear stress for long time, the particles would be uniformly coated with the surfactant thus preventing particle aggregation and drug leakage (Helgason et al., Citation2009).
Through steric stabilization, non-ionic surfactants such as PVA are able to stabilize nanoparticles. Particles are wet rapidly when the surfactant reaches critical level. Wetting process is further accelerated when the homogenization time is increased. The present experiment also showed that particle size reduces when homogenization speed and homogenization time are maintained at a high level. This further increases the need for a high amount of PVA. Thus, the level of PVA depends homogenization time and speed. The nanoparticles will therefore be coated completely with PVA only if higher shear stress is maintained for sufficiently long time (Sis & Birinci, Citation2009).
Although zeta potential could be affected by particle size distribution, it is difficult to exactly correlate the effect of homogenization speed, homogenization time and surfactant concentration (independent variables used in the present work) on zeta potential as the effect of all these factors are interdependent. As discussed previously as the total surface area in the system increases with increase in the number of small sized particles, an increase in the total surfactant level due to increase in the amount of surfactant coated particles will be observed. If the surfactant is ionic in nature, then an increase/ decrease in zeta potential could be observed depending on the type of surfactant used. PVA used in the present experiment is a non-ionic surfactant. Although homogenization speed was observed to increase the zeta potential, interaction of speed with PVA was found to reverse the effect on zeta potential ( and ; and ). Further experiments were carried out using curcumin-loaded PCL nanoparticles of size 391.67 ± 19.78 nm (), and zeta potential of 29.03 ± 1.04 mV () prepared using optimized conditions.
Figure 3. Effect of various levels of homogenization speed and PVA concentration on (a) percentage drug encapsulation, (b) mean particle size and (c) zeta potential when the level of curcumin-PCL ratio is maintained at highest level.
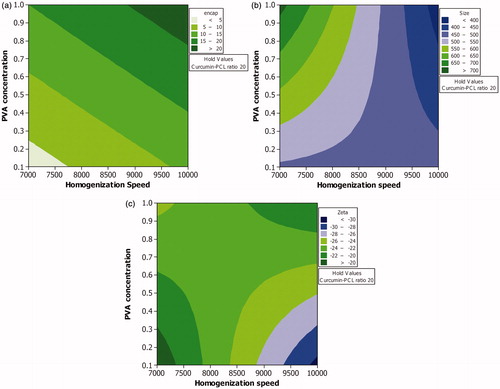
Drug release
Drug release studies showed that after initial burst release, the amount of drug released was low and steady. Initial burst release could be due to the release of curcumin adsorbed to the surface of the CPNs ().
In-vivo anticancer study by EAC tumor model in Swiss albino mice
Efficacy of the CPN in controlling tumor progression was investigated in-vivo using EAC model. Although EAC model may not give complete idea on therapeutic efficacy, it is the simplest, cost effective and less time consuming model to analyzing anticancer efficacy of a formulation. Formulation showing good efficiency can be taken up further studies. Increase in body weight of animals that received intervention (plain curcumin and CPN) was less when compared to the EAC control (). MST in case of EAC control, curcumin and PCL-curcumin treated groups were 15, 18 and 16.5 d, respectively (). There was a significant (p value <0.05) reduction in tumor volume in both the treatment groups (plain curcumin and curcumin-loaded PCL) (). Both the treatment groups were able to control EAC induced decrease in the hemoglobin () and RBC level () and EAC induced increase in WBC level ().
Figure 7. Effect of plain curcumin and curcumin-loaded PCL nanoparticles on (a) body weight and (b) mean survival time.
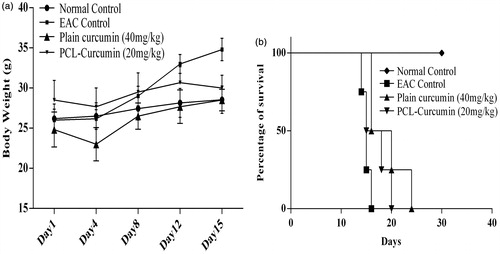
Figure 8. Effect of plain curcumin and curcumin-loaded PCL nanoparticles on hematological parameters (a) tumor volume, (b) hemoglobin level, (c) RBC level and (d) WBC level. *indicates that the treatment with plain curcumin and PCL-curcumin nanoparticles significantly (p-value < 0.05) controlled the proliferation of EAC cells.
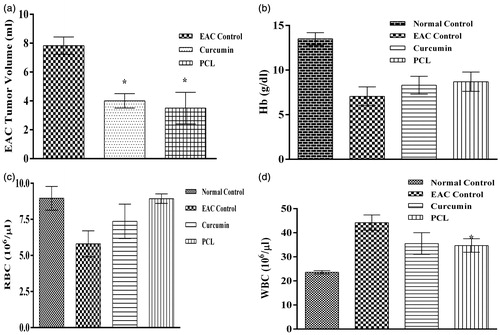
Although the study showed that the survival time in case of CPN treated animals was not significantly higher (p value >0.05) than the plain curcumin-treated animals, CPNs were given at half the dose of plain curcumin. Also, the drug release from the CPN was in a sustained manner. This property would make curcumin more effective in treating solid tumors as it would avoid repeated dosing. CPN could be further made to release the drug only at the target through suitable surface modification by incorporation of suitable ligands or by delivering as in-situ polymer based implant whereby the formulation is directly injected into the target site. Further experiments using higher dose of CPN and different animals models could help in complete understanding of the true benefit of CPN. Also tuning the drug administration based on the drug release profile could help in achieving a better efficacy.
PLGA in-situ injectable implant
Even after the tumor mass is removed from its site of origin, it is better to treat the target site for an extended period of time to prevent relapse. It would be beneficial if repeated administration is avoided and the drug is released in sustained manner from a single dose. This would reduce the frequency of administering the drug thereby improving patient compliance. The drug (nanoparticles in the present case) from an in-situ injectable implant, upon injection close to/ into the target site will be released near/ within the target site continuously for an extended period of time. Previous studies show that implants are indeed more effective in controlling tumor progression (Madan et al., Citation2009). The CPN dispersed PLGA in-situ injectable implant was formulated and in-vitro drug release study was conducted in pH 7.4 buffer. The in-situ implant quickly precipitated after slow injection into the aqueous solution due to the solvent diffusion effect of the organic solvent with the aqueous media. The in-vitro drug release profile from the in-situ injected implant showed that the curcumin was released from the formulation in a slow and a sustained manner (). This is due to the quick precipitation of the nanoparticles dispersed polymer solution in an aqueous medium arising from solvent diffusion and precipitating effect. This results in a highly entangled polymer network trapping the PCL nanoparticles which slowed and sustained the drug release in the aqueous dissolution medium. The initial burst release observed in case of CPNs was absent in case of implant. Although the curcumin that were adsorbed to the surface of CPN would have got released, its diffusion into the medium is limited by the presence of PLGA network around CPN. Therefore, the drug will get accumulated within PLGA mesh like network and will be slowly released into the medium depending on the pore size of the network and degradation of PLGA. Further studies on physical properties and the therapeutic application of this implant using various animal models will help in understanding the therapeutic efficacy of this formulation. But this would be possible only after identifying the suitable dose of CPN.
Conclusion
The role of independent variables viz., drug–polymer ratio, PVA concentration, homogenization speed and homogenization time involved in development of curcumin-loaded PCL nanoparticles was studied and optimized using DoE. The study showed that interactions among the independent variables especially homogenization speed and PVA concentration exhibited higher influence when compared to the effect of the independent variables as such on the outcome. Using these statistical tools curcumin-loaded PCL nanoparticles of size 391.67 ± 19.78 nm, percentage drug encapsulation of 17.39 ± 1.01% and zeta potential of 29.03 ± 1.04 mV was achieved when the process variables were maintained at the selected conditions (drug–polymer ratio: 1:20, surfactant concentration: 0.9% w/v, homogenization speed: 10 000 rpm and homogenization time: 30 min). Curcumin-loaded nanoparticles were able to delay tumor progression in mice. PLGA based in-situ implant containing curcumin-PCL nanoparticles showed drug release in a sustained manner.
Acknowledgements
Our thanks are due to authorities of Manipal University for providing the required facilities to carry out the work.
Declaration of interest
We hereby declare that there is no conflict of interest with any organization regarding the material discussed in this article.
References
- Anand P, Kunnumakkara AB, Newman RA, Aggarwal BB. (2007). Bioavailability of curcumin: problems and promises. Mol Pharmaceut 4:807–18
- Anand P, Nair HB, Sung B, et al. (2009). Design of curcumin-loaded PLGA nanoparticles formulation with enhanced cellular uptake, and increased bioactivity in vitro and superior bioavailability in vivo. Biochem Pharmacol 79:330–8
- Anderson MJ, Whitcomb PJ. (2000). Design of experiments. Kirk-Othmer Encyclopedia Chem Technol 1–22
- Bianco H, Marmur A. (1992). The dependence of the surface tension of surfactant solutions on drop size. J Coll Interface Sci 151:517–22
- Guideline ICHHT. (2009). Pharmaceutical development. Q8 (2R). As revised in August
- Helgason T, Awad TS, Kristbergsson K, et al. (2009). Effect of surfactant surface coverage on formation of solid lipid nanoparticles (SLN). J Coll Interf Sci 334:75–81
- Khalil NM, Nascimento TCFD, Casa DM, et al. (2013). Pharmacokinetics of curcumin-loaded PLGA and PLGA–PEG blend nanoparticles after oral administration in rats. Coll Surf B: Biointerface 101:353–60
- Koganti S, Jagani HV, Palanimuthu VR, et al. (2013). In vitro and in vivo evaluation of the efficacy of nanoformulation of siRNA as an adjuvant to improve the anticancer potential of cisplatin. Exp Mol Pathol 94:137–47
- Kushwaha AK, Vuddanda PR, Karunanidhi P, et al. (2013). Development and evaluation of solid lipid nanoparticles of raloxifene hydrochloride for enhanced bioavailability. BioMed Res Int 9
- Lee SC, Oh JT, Jang MH, Chung SI. (1999). Quantitative analysis of polyvinyl alcohol on the surface of poly (D, L-lactide-co-glycolide) microparticles prepared by solvent evaporation method: effect of particle size and PVA concentration. J Control Rel 59:123–32
- Lionberger RA, Lee SL, Lee L, et al. (2008) Quality by design: concepts for ANDAs. AAPS J 10:268–76
- Madan M, Bajaj A, Lewis S, et al. (2009) In situ forming polymeric drug delivery systems. Ind J Pharmaceut Sci 71:242–51
- Pal S, Choudhuri T, Chattopadhyay S, et al. (2001) Mechanisms of curcumin-induced apoptosis of Ehrlich's ascites carcinoma cells. Biochem Biophys Res Commun 288:658–65
- Ranjan AP, Mukerjee A, Helson L, et al. (2013). Efficacy of liposomal curcumin in a human pancreatic tumor xenograft model: inhibition of tumor growth and angiogenesis. Anticancer Res 33:3603–9
- Raveendran R, Bhuvaneshwar GS, Sharma CP. (2013). In vitro cytotoxicity and cellular uptake of curcumin-loaded Pluronic/Polycaprolactone micelles in colorectal adenocarcinoma cells. J Biomater Appl 27:811–27
- Sis H, Birinci M. (2009). Effect of nonionic and ionic surfactants on zeta potential and dispersion properties of carbon black powders. Coll Surf A: Physicochem Eng Aspects 341:60–7
- Telford JK. (2007). A brief introduction to design of experiments. Johns Hopkins APL Tech Digest 27:224–32
- Yallapu MM, Jaggi M, Chauhan SC. (2012). Curcumin nanoformulations: a future nanomedicine for cancer. Drug Discov Today 17:71–80
- Yallapu MM, Jaggi M, Chauhan SC. (2013). Curcumin nanomedicine: a road to cancer therapeutics. Curr Pharmaceut Design 19:1994–2010