Abstract
Injectable In situ gel-forming chitosan/β-glycerol phosphate (CS/β-Gp) solution can be introduced into the body in a minimally invasive manner prior to solidifying within the target tissue. This hydrogel is a good candidate for achieving a prolonged drug delivery system for insulin considering its high molecular weight. In addition to the physicochemical characterization of this hydrogel, in vitro and in vivo applications were studied as a sustained insulin delivery system. In the in vitro release studies, 19–63% of total insulin was released from the CS/β-Gp hydrogel within 150 h at different β-Gp and insulin concentrations. The best formulation was selected for in vivo experimentation to control the plasma glucose of diabetic mice models. The hypoglycemic effect of this formulation following subcutaneous injection in diabetic mice lasted 5 d, significantly longer than that of free insulin solution which lasted several hours.
Introduction
Recently, controlled release systems capable of being implanted near the diseased organ have been investigated to maintain a high therapeutic concentration of drugs in the target area (Kim et al., Citation2010). In addition to the high probability of infection and surgical difficulties encountered with these implantable systems that restricts their application, the presence of a polymeric membrane could hinder appropriate release of large molecular weight drugs such as insulin (Short, Citation2008). These complications associated with implantable systems have led to investigations based on injectable in situ forming hydrogels as promising systems for controlled release of large molecular weight drugs (Ganji & Vasheghani-Farahani, Citation2008; Yan et al., Citation2010). The advantage of injectable hydrogels over other systems is that they are in liquid form below or at room temperature and undergo in situ gelation in the body and easily fill irregular cavities present in an injured tissue (Hoemann et al., Citation2005; Kempe et al., Citation2008). Moreover, they enhance patient compliance in avoiding surgical procedures as well as frequent injections of drug, and prolonged local release of therapeutic agents is achieved by a simple injection (Zheng et al., Citation2010).
Chitosan is an abundant, naturally derived aminopolysaccharide obtained from the partial deacetylation of chitin (Richardson et al., Citation2008), the main component of the cell walls of fungi and exoskeletons of crustaceans and insects (Chenite et al., Citation2001). Chitosan consists of β-(1,4)-linked d-glucosamine units with variable degrees of N-acetylation (Casettari et al., 2012). It has been used for tissue regeneration (Ma et al., Citation2010; Cooper et al., Citation2011; Park et al., Citation2013) and drug delivery applications (Rokhade et al., Citation2007; Abruzzo et al., Citation2012; Ferrari et al., Citation2013) because of its tissue compatibility, biodegradability and low toxicity (Ao et al., Citation2011; Cooper et al., Citation2011; Tsai et al., Citation2011).
Chitosan/β-glycerol phosphate (CS/β-Gp) is an injectable in situ gel-forming formulation that can be placed near a target organ in a minimally invasive manner and forms a solid-like gel after exposure to body temperature (Kim et al., Citation2010). The biocompatibility of CS/β-Gp hydrogel has been investigated (Molinaro et al., Citation2002; Ji et al., Citation2010; Ngoenkam et al., Citation2010). Zhou et al. (Citation2011) reported good blood compatibility with no significant changes in the hematology of Sprague–Dawley rats for white and red blood cells, platelets and the volume of hemoglobin after intramuscular injection of CS/α,β-Gp. Zhao et al. (Citation2009) found good spreading and morphology of mouse embryonic fibroblast cells cultured on CS/β-Gp hydrogel films.
The biomedical applications of this thermosensitive in situ gel-forming formulation have previously been reported. Ruel-Gariépy et al. (Citation2004) proposed CS/β-Gp thermosensitive hydrogel as a promising formulation for delivery of paclitaxel to prevent recurrence of localized tumors. They studied cancer cell growth after intratumoral subcutaneous injections in implanted EMT-6 tumors on Balb/c mice and found that paclitaxel-loaded CS/β-Gp inhibited the growth of tumors in a less toxic manner than Taxol®.
Kim et al. (Citation2010) investigated the application of a CS/β-Gp system for ellagic acid (EA) delivery for brain cancer treatment. They found that chitosan gels containing EA significantly reduced the growth of human U87 glioblastomas and rat C6 glioma cancer cells in an EA concentration-dependent manner.
Cheng et al. (Citation2011) developed thermosensitive gelatin/CS/Gp (G/CS/Gp) hydrogel for prolonged release of ferulic acid (FA) in nucleus pulpous (NP) regeneration. They found that post-treatment of FA-G/CS/Gp inhibited the apoptosis of NP cells caused by reactive oxygen species. In another work, they showed that the thermosensitive FA-G/C/GP hydrogel can treat NP cells from the damage caused by oxidative stress and can be applied in minimally invasive surgery for NP regeneration (Cheng et al., Citation2013).
Although studies have demonstrated the good potential of this in situ gelling hydrogel for encapsulating drugs and biologic agents, little work has been published on the preparation of sustained insulin delivery using a CS/β-Gp solution. Wu et al. (Citation2006) showed the capacity of quaternized chitosan and poly(ethylene glycol) (PEG) with a small amount of α,β-Gp for insulin delivery. They showed that a HTCC–PEG–Gp formulation can be used as a nasal drug delivery system to improve the absorption of hydrophilic macromolecular drugs.
Kempe et al. (Citation2008) used electron paramagnetic resonance spectroscopy to study the microviscosity and pH inside CS/β-Gp hydrogel, and showed that this thermogelling system is an attractive delivery system for peptides and proteins such as insulin. They also found that insulin is not immobilized locally within the hydrogel and insulin incorporation into the gel has no impact on its stability. Peng et al. (Citation2013) developed a novel long-term sustained and controlled delivery system of insulin based on CS/β-Gp hydrogel. They loaded poly(3-hydroxybutyrate-co-3-hydroxyhexanoate) (PHBHHx) nanoparticles containing insulin phospholipid complex into CS/β-Gp hydrogels. Although, the bioavailability of insulin was significantly enhanced in their work, release behavior was slowed down substantially (19.11% of the initially loaded insulin released in 31 d).
The present study investigates the potential of CS/β-Gp hydrogel as an insulin sustained release system. Chitosan/β-Gp/insulin solutions were prepared at different concentrations of β-Gp and insulin. Various properties of the resultant hydrogels including pH, gelation time, turbidity, rheology and biocompatibility were studied. The in vitro release profile and structural stability of the insulin were monitored and in vivo insulin release after subcutaneous injection of the CS/β-Gp formulation was studied in both normal and diabetic mice.
Materials and methods
Materials
Chitosan with average molecular weight and high degree of deacetylation (DDA = 95%) was purchased from Primex Ehf (Siglufjörður, Iceland). Hydrated β-glycerophosphate disodium salt–pentahydrate (MW =306.12), streptozotocin (STZ), 8-anilino-1-naphthalenesulfonic acid (ANS) and diethyl ether (MW = 74.12) were obtained from Sigma-Aldrich (St. Louis, MO). HCl (37% wt), tri-sodium citrate 5,5-hydrate (MW = 357.16), orthophosphoric acid (85% wt), disodium hydrogen phosphate 2.H2O (MW = 177.99), sodium dihydrogen phosphate 2.H2O (MW = 156.01) and Coomassie brilliant blue G-250 (MW = 854.03) were provided by Merck (Darmstadt, Germany). Human recombinant insulin (28.82 IU/mg) was purchased from Exir Parmaceutical Co. (Boroujerd, Iran).
Preparation of insulin-loaded CS/β-Gp thermosensitive solutions
Chitosan (200 mg) was dissolved in 8 ml aqueous hydrochloric acid (0.1 M) to create a transparent solution. The chitosan solution was sterilized by autoclaving at 120 °C for 10 min. Different concentrations of β-GP were dissolved in distilled water and filtered through a 0.22-μm filter. The chitosan solution was placed in an ice-water bath at ∼4 °C and insulin dissolved in a phosphate buffer (pH 7.4) was added to the solution under stirring. The β-GP solution was added to the solution dropwise over 10 min under stirring. The final 10 ml solution contained 2% (w/v) chitosan and 5–14% (w/v) β-GP and maintained at 4 °C for further studies.
Turbidity
The turbidity of the chitosan and CS/β-GP solutions was monitored using a Turb 430 IR/T turbidity meter (Xylem Inc., Munich, Germany). The chitosan and CS/β-GP solutions were poured into quartz cells and their turbidity was determined at 37 °C according to ISO 7027 using standard Formazin suspensions at 0.02, 10 and 1000 FTU (Formazin turbidity unit).
Rheologic characteristics
Rheologic characterization of the CS/β-GP solutions was performed using an ARES rheometer (New Castle, DE). In this experiment, the effect of β-Gp concentration on the thermosensitivity of the CS/β-Gp formulation was examined. Samples containing 8 and 14% (w/v) β-Gp were placed into the rheometer and loss modulus (viscous) and storage modulus (elastic) changes at 37 °C were determined as a function of time. Frequency was fixed at 1 Hz and strain at 1% to assure the linear area of viscoelastic properties. The gelation point was considered to be the point at which the loss and storage moduli values were the same.
pH measurement
Neutral pH value is appropriate for drug performance and there is a relationship between the addition of β-Gp and an increase in the pH of CS/β-GP solutions, so the effect of β-Gp on pH was determined pH-220L, ISTEK, Inc., Seoul, Korea. CS/β-GP solutions (5 ml) were kept at 4 °C for at least 12 h to eliminate the trapped bubbles. They were then transferred to 10 ml beakers and their pH values were measured three times at room temperature.
Gelation time
The inverted-tube test method was utilized to determine the gelation time of the CS/β-GP solutions (Wu et al., Citation2007). In this method, a test tube containing the formulation is tilted and is defined as a solution phase if it flows and as a gel phase if there is no flow. Tubes of 1 cm in diameter containing 0.5 ml of the liquid formulations were refrigerated for at least 12 h to eliminate air bubbles. The gelation time of the cold solutions (kept at 4 °C) was determined at a constant temperature of 37 °C for concentrations of β-Gp in the range of 5–14% (w/v).
To reduce the gelation time without increasing β-Gp content, the samples were maintained at room temperature for 10 min (warm solutions); their gelation time was then determined by the inverted-tube test method. To investigate the effect of insulin on gelation time, different concentrations (0–0.3 mg/ml) of insulin were mixed with a solution containing 8% (w/v) β-Gp and gelation time was determined.
In vitro insulin release
To investigate the insulin release profile, samples of 2% (w/v) chitosan, and 8 or 14% (w/v) β-Gp containing insulin at concentrations of 0.03 and 0.1% (w/v) were used. The release experiment was performed using 0.5 ml of solution in 2 ml microtubes. The tubes were incubated at 37 °C for 2 h to complete the gelation process. After gel forming, 700 μl of phosphate buffer at pH 7.4 was added to each tube and 400 μl of phosphate buffer was collected at predetermined times and replenished with 400 μl of fresh buffer. The released insulin concentration was measured using the Bradford method with UV spectrophotometer (Cary WinUV, Varian, Inc., Palo Alto, CA).
In vitro structural stability of insulin
The overall structural stability of released insulin from CS/β-GP hydrogel was compared to that of the lyophilized pure insulin using fluorescence spectroscopy. ANS was used as a hydrophobic dye to monitor changes in the surface hydrophobicity of insulin. A fresh ANS stock solution was prepared using double-distilled water. An aliquot of 1 μl of the ANS solution was added to 1 ml of buffer to create a final concentration of 10 μm. A blank spectrum without protein was recorded before adding the insulin solution to the ANS solution. Fluorescence was measured (Spectrofluorophotometer; Shimadzu Corp., Tokyo, Japan) immediately after the addition of insulin to the ANS solution. The emission spectra were recorded from 400 to 550 nm with an excitation at 370 nm.
Subcutaneous injection in normal mice
Male mice were provided by the animal center of Department of Physiology of Tarbiat Modares University (Tehran, Iran). They weighed 30–35 g and fasted overnight before the experiment. Four mice were tested in each group and anesthetized using diethyl ether. Samples 200 µl in volume with 8% (w/v) β-Gp and different concentrations of insulin were administrated subcutaneously using a 23 gauge needle to the back of the neck. Blood glucose level was measured up to 8-h post-injection using a Glucocard 01-mini glucometer and the glucose oxidase method.
Subcutaneous injection in diabetic mice
Ten male mice weighing 30–35 g were used. They were made diabetic using STZ dissolved in a sodium citrate buffer at pH = 4.5. STZ solution (100 µl) containing 60 mg STZ/kg was administrated by intraperitoneal injection daily for 5 d. One week after the last injection, mice having a blood glucose level >300 mg/dl were considered to be diabetic. Two groups, containing three diabetic mice were considered: 200 µl CS/β-Gp/insulin liquid formulation with 0.01 mg/ml insulin was injected to one group and the other was designated as a control for STZ effect. Blood glucose was measured at regular intervals and the data from the two groups were compared. Benferroni analysis was used to determine statistical significance.
Toxicity test
System toxicity was determined by measuring changes in the activity of the enzymes aspartate transaminase (AST), creatine phosphokinase (CPK), alkaline phosphatase (ALP) and amylase after subcutaneous injection. Blood samples were taken 7 d after administration and plasma samples gathered by centrifugation. Enzyme activity was determined using enzyme-linked immunosorbent assay kits.
Statistical analysis
Statistical data analysis was performed using Student's t-test with a confidence interval of p < 0.05 when two groups were analyzed. When more than two groups were analyzed, one-way ANOVA was used with a 95% confidence interval (p < 0.05) as the minimal level of significance. The results are expressed as means of at least three tests ± standard deviation (SD). A level of p ≤ 0.05 was set as significant, p ≤ 0.01 for very significant and p ≤ 0.001 for highly significant.
Results and discussion
Thermosensitivity of CS/β-Gp solutions
Chitosan is a pH-dependent cationic biopolymer that is typically not soluble in water, but could be solubilized in acidic aqueous solutions via the protonation of its amine groups. Once dissolved, chitosan remains in solution up to pH < 6.2 (, sample CS1). Neutralization of chitosan solutions with a strong base up to pH > 6.2 instantly leads to the formation of a hydrated gel-like precipitate. The positive charges of the chitosan amine groups are neutralized at this point and their repulsion eliminated, which results in the formation of chitosan–chitosan hydrogen bonds via its hydroxyl (–OH), amine (–NH2), amide (–NH–C) and carbonyl (–C = O) groups (Casettari et al., Citation2012).
Table 1. Characteristics of thermosensitive CS/β-Gp solutions.
Rapid gel formation occurred at room temperature (, sample CS2). Interestingly, the acidic chitosan solution can be neutralized up to a physiologic pH ∼7.2 using β-Gp salt without formation of immediate gel-like precipitate (, samples CS3–CS9 at 25 °C). The acidic chitosan solution did not show thermosensitivity itself (, sample CS1), but subsequent heating of the CS/β-Gp solution resulted in the hydrogel formation (, samples CS3–CS9 at 37 °C).
Turbidity changes in chitosan solution with and without β-Gp salt at 37 °C as a function of time were investigated (). The turbidity of chitosan solution did not significantly change over time; this demonstrates that chitosan does not form a hydrogel without the addition of β-Gp. The turbidity of CS/β-Gp solution increased slowly at first (showed the start of gelation), dramatically increased to >1000 FTU after 9 min, and continued to increase slowly up to 1 h.
Figure 1. Turbidity changes by time for chitosan solutions incubated at 37 °C in the absence of β-Gp (diamonds) and in the presence of β-Gp (squares).
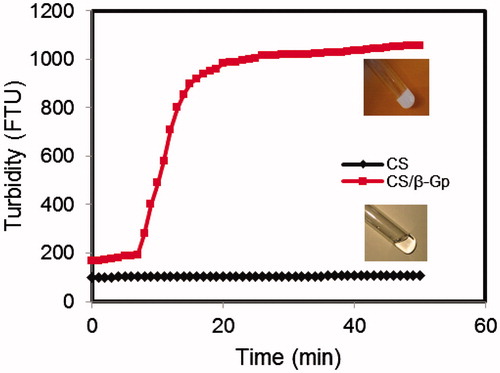
The gelation mechanism of CS/β-Gp solution has previously been reported (Cho et al., Citation2005; Li et al., Citation2010). Briefly, electrostatic attraction between oppositely charged ammonium groups of chitosan and negatively charged phosphate moieties of β-Gp salt allows for attractive hydrophobic and hydrogen bonding between chitosan chains, which are the main molecular forces responsible for the sol/gel transition in CS/β-Gp solutions. shows the rheologic behavior of sample CS5 (8% w/v β-Gp) during sol-to-gel transition at 37 °C. At first, G′ and G′′ showed small, nearly constant values; the loss modulus (G′′ = 11.5 Pa) was greater than the storage modulus (G′ = 8.4 Pa) at 37 °C at time zero. This shows that viscous behavior was dominant before the gelation occurred. At 37 °C, the values of the storage modulus increased by time to values greater than those for the loss modulus; at the gelation point, it increased very rapidly.
Figure 2. Storage (G′) and loss (G′′) moduli changes for CS/β-Gp solution (CS 2% w/v) over time at 37 °C: (a) β-Gp 8% (w/v) and (b) β-Gp 14% (w/v).
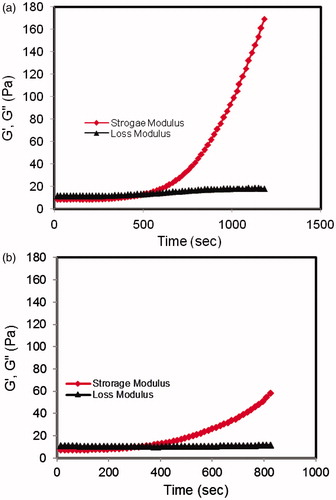
The gelation occurred at the point where these two moduli reached equal values. After the gelation point, the storage modulus increased to a much higher value than that of the loss modulus. It exhibited a solid-like behavior in which elastic behavior was dominant. The same behavior was recorded for sample CS9 (14% w/v β-Gp) in .
The influence of β-Gp salt concentration on the thermosensitivity of chitosan solution was also investigated (). Evidently, by increasing the β-Gp concentration the gelation time decreased. For example, the gelation time for the chitosan solution with 14% (w/v) β-Gp was determined to be ∼4 min, while gel formation for the 5% (w/v) β-Gp solution was >16 min (, samples CS9 and CS3, gelation times for cold solution). By increasing the concentration of β-Gp, more amine groups are neutralized and more interactions between the chitosan chains and intra- and inter-chain hydrogen bonds between OH–NH and O–HN in opposing chains are created. As a result, the time required for the completion of gelation at 37 °C decreased. The results of the rheologic studies confirmed this (). It is clear that the time at which the storage modulus reached the loss modulus was lower for the sample with a high β-Gp concentration ().
Effect of the initial CS/β-Gp solution temperature on its thermosensitivity was also studied (, gelation times for warm solution). Increasing the initial solution temperature (from 4 to 25 °C) decreased the required time for gelation. For example, cold chitosan solution containing 14% (w/v) β-Gp formed a gel in 4 ± 1.3 min while the gelation time decreased to 2 ± 1.0 min for the warm solution (, sample CS9). Statistical analysis indicates that the temperature significantly affected the gelation time (p < 0.05). The temperature increase caused effective forces in the gelation process to be intensified. It also increased entropy of the solvent and changed the structure of the highly ordered water molecules around the chitosan chains, simplifying gelation at high temperatures. The results of this study are in good agreement with those obtained by other researchers (Kim et al., Citation2010). They investigated the Fourier Transform Infrared Spectroscopy (FTIR) spectra of CS/β-Gp at 5 and 37 °C and found that increasing temperature allowed phosphate groups of β-Gp to accept proton and proton transfer occurring during gelation. At 37 °C, diproton phosphate groups (–H2PO4) were present in the solution, but they were not detected in solution at 5 °C. The increase in temperature increased the entropy of the chitosan chains, causing them to release their protons and the phosphate groups of β-Gp to accept the released protons. As a result, a decrease in protonation occurred in the chitosan molecules that decreased their solubility. Elimination of hydrating water molecules surrounding the chitosan chains increased the interactions between chitosan molecules and allowed gelation to occur.
To investigate the effect of drug loading on the gelation time of CS/β-Gp solutions, different amounts of insulin were added to the solution at room temperature and the gelation time of each sample was determined (data not shown). As the insulin concentration increased, gelation time decreased. The presence of amino and hydroxyl groups allowed the insulin to form hydrogen bonds with the chitosan chains. Increasing the hydrogen bonds decreased the gelation time. Wu et al. (Citation2007) developed an injectable thermosensitive hydrogel based on quaternized chitosan for nasal delivery of insulin and found similar results for the initial insulin loading effect on the gelation time.
Thermoreversibility of CS/β-Gp solutions
Upon the completion of gel formation at 37 °C, the hydrogels were maintained at 4 °C overnight to investigate the thermoreversibility of the hydrogels. No thermoreversibility was observed in the samples () although other researchers have observed thermoreversible behavior in some CS/β-Gp solutions (Chenite et al., Citation2000). These results could be attributed to the high DDA of chitosan and high β-Gp concentration in the samples of the present study. Under these conditions, the hydrogen bonds between the chitosan chains and chitosan–water molecules predominate because of the neutralizing effects of β-Gp salt. Since hydrogen bonds are not temperature dependent, cooling the hydrogel did not affect the gel structure. Chenite et al. (Citation2000) studied low concentrations of β-Gp salt and found that gelation mainly occurred via hydrophobic interactions. Since hydrophobic forces are known to be temperature dependent (Ngoenkam et al., Citation2010), cooling the hydrogel caused this weak hydrogel to turn into a liquid solution. It could be concluded that thermoreversibility of the gelation of chitosan hydrogel may be related to the competition between the different molecular forces involved in gel formation.
In vitro insulin release
Insulin was trapped in the CS/β-Gp hydrogels () and its cumulative release behavior was investigated in vitro as shown in . The initial burst release was relatively low: 12.26, 10.23, 5.98 and 4.24% of the drug was released within 8 h from samples CS-In1, 2, 3 and 4, respectively ( and ). A similar release profile was observed by other researchers (Wu et al., Citation2006, Citation2007; Kempe et al., Citation2008).
Figure 3. Insulin release profiles from hydrogels prepared with different concentrations of β-Gp [CS 2% (w/v), insulin 0.3 mg/ml]. Data are mean ± SD (n = 3).
![Figure 3. Insulin release profiles from hydrogels prepared with different concentrations of β-Gp [CS 2% (w/v), insulin 0.3 mg/ml]. Data are mean ± SD (n = 3).](/cms/asset/790ca7c7-2511-40a9-8381-6a860d8b0524/idrd_a_932861_f0003_c.jpg)
Figure 4. Extrinsic fluorescence at λex = 370 nm and λem = 400–550 nm of (a) ANS, (b) pure insulin and (c) insulin in Formulation 1.
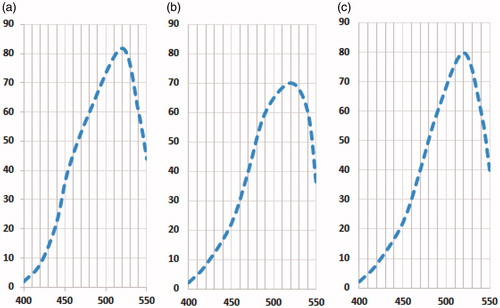
Table 2. Characteristics and kinetic profile of insulin-loaded CS/β-Gp hydrogels.
This indicated that insulin was partially adsorbed onto the surface or distributed among the tunnels of the hydrogel during gelation and then diffused rapidly when the hydrogel came into contact with the release medium.
After an initial burst release, the insulin trapped in the CS/β-Gp hydrogels was released slowly. The release rates of all samples actually decreased by time, which could be attributed to drug recession in the chitosan matrix and a decrease in the concentration gradient of the drug (dC/dx). Evidently, the gel characteristics affected the efficiency of insulin release ( and ). Both the rate and total drug release decreased as the β-Gp salt and insulin concentrations in the formulations increased. Increasing the β-Gp salt concentration resulted in extensive hydrogen bonding via the OH–NH and O–HN in the chitosan chains; increasing the insulin concentration resulted in an increase in the number of intermolecular hydrogen bonds in the CS/β-Gp gel. These form a tight network and effectively hinder drug release from the gel. Diffusing insulin from a rigid network is obviously slower than that from a floppy network.
In vitro insulin structural stability
It has been reported by several researchers that CS/β-Gp retains biologic activity of the incorporated therapeutics and growth factors (Chenite et al., Citation2000). CS/Gp formulation loaded with paclitaxel indicated higher tumor inhibition capability compared to free Taxol® after being injected intratumorally (Ruel-Gariépy et. al., Citation2004). In vivo experiments showed that insulin incorporated in HTCC–PEG–Gp created a better hypoglycemic effect in rats compared to free insulin in phosphate-buffered saline solution (Wu et al., Citation2007). In this study, the stability of insulin released from CS/β-Gp hydrogel (, sample CS5, β-Gp 8% w/v) was studied in vitro using extrinsic fluorescence spectroscopy. Hydrophobic dyes such as ANS are commonly used to the monitor conformational changes to the structure of proteins. The 3D structural changes in protein may alter the intensity and/or λem of ANS. It has a high affinity for binding to the hydrophobic sites of moderately unfolded proteins, enhancing fluorescence intensity (Fu et al., Citation2005).
In this study, insulin released from the gels was incubated with ANS. No changes in Emax or fluorescence intensity were observed (). The results showed that insulin retained its native structure. No change in ANS fluorescence intensity or maximum emission was observed after addition of insulin to the ANS solution (). This indicates that the formulations used in this study had no significant effect on the folded insulin structure. Furthermore, since the insulin showed little interaction with ANS, no significant structural changes were induced by solubilization in an acidic CS/β-Gp solution.
Hypoglycemic effect of CS/β-Gp gel in normal and diabetic mice
The stability of CS/β-Gp during the sustained and controlled release of insulin was evaluated by following its hypoglycemic effect over a period in diabetic Balb/c mice after subcutaneous injection. The in vitro results showed that increasing the β-Gp concentrations led insulin release rate to decrease. It also caused a significant increase in osmotic pressure; since β-Gp diffused out of the gel and high amounts of β-Gp were dispersed in the body. An ideal system is that with the least amount of β-Gp, thus, the CS/β-Gp formulation with 8% (w/v) β-Gp was chosen for the in vivo studies. shows the plasma glucose levels for Balb/c mice after subcutaneous administration of CS/β-Gp with different concentrations of insulin. A dramatic decrease in blood glucose was observed 1-h post-injection, and the decreased levels continued until 5-h post-injection and slowly increased thereafter. By increasing insulin concentration in the system, blood glucose level decreases suggesting more insulin release; which is consistent with the results obtained from in vitro work. The CS/β-Gp blank solution was also injected as a control. The mice subcutaneously injected with the control formulation showed normal blood glucose levels throughout the study; a small change in blood glucose level arose from fasting during the experiment because CS/β-Gp is not active enough to adjust blood glucose values (Peng et al., Citation2013; Wu et al., Citation2007). In addition to drug diffusion, hydrogel degradation could play an important role in drug release behavior. Degradability of chitosan in the presence of enzymes is reported in previous studies (Rao & Sharma, Citation1997; Kim et al., Citation2010). Partial biodegradation of chitosan hydrogel was reported in ∼10–14 d post-implantation into the back of mice (Obara et al., Citation2003). It is well identified that chitosans with lower DDAs are more easily biodegraded due to the presence of blocks of glucosamine moieties containing acetyl groups that serve as a substrate for lysozyme. It was also reported that in vivo biodegradation of chitosan with 85% DDA lasted ∼6 month (Berrada et. al., 2005). In the present study, we used chitosan with 95% DDA with any substantial erosion of hydrogel structure during the first 7 d, so no effect of degradation on release kinetics was observed.
Figure 5. Blood glucose profiles after subcutaneous dorsal injection of CS/β-Gp/insulin with different insulin concentrations into (a) normal mice [CS 2% (w/v), β-Gp 8% (w/v)] (b) diabetic mice [CS 2% (w/v), β-Gp 8% (w/v), insulin 0.01 mg/ml]. Data are mean ± SD (n = 4).
![Figure 5. Blood glucose profiles after subcutaneous dorsal injection of CS/β-Gp/insulin with different insulin concentrations into (a) normal mice [CS 2% (w/v), β-Gp 8% (w/v)] (b) diabetic mice [CS 2% (w/v), β-Gp 8% (w/v), insulin 0.01 mg/ml]. Data are mean ± SD (n = 4).](/cms/asset/42c0a662-c34f-4971-9532-05ed05daa771/idrd_a_932861_f0005_c.jpg)
shows the blood glucose concentration as a percentage of initial level versus time after subcutaneous injection of insulin-loaded CS/β-Gp hydrogel into diabetic Balb/c mice. CS/β-Gp without insulin was also injected into a control group.
A high decrease in blood glucose level was observed 8-h post-injection of the insulin-loaded CS/β-Gp formulation. The minimum blood glucose level was observed 2-h post-injection (21.73 ± 7.79% of initial blood glucose level), although normal blood glucose level was reached at this time (96.33 ± 34.53 mg/dl). After administration of CS/β-Gp hydrogel, the blood glucose levels dropped because of the overnight fasting of STZ mice before the experiment. Although, insulin burst release in the first 8-h post-injection due to the sudden release of surface-adsorbed insulin could accelerate this phenomenon. It was reported by Wu et al. (Citation2007) that the lowest blood glucose level was observed 4- and 5-h post-administration of insulin-loaded HTCC–PEG–Gp containing 3.6 and 4.5 wt% of PEG, respectively.
Benferroni statistical analysis indicates that the system significantly lowered blood glucose levels for 120-h post-administration (p < 0.05). The percentage of blood glucose relative to the initial level was <70% for ∼128 h; this is significantly longer than that of the free insulin solution, which lasted several hours (Peng et al., Citation2013). Since 70% of the initial blood glucose level is the threshold of a therapeutic effect, obtained result indicates that CS/β-Gp hydrogel played a substantial role in prolonging the hypoglycemic effect of insulin. Moreover, Peng et al. (Citation2013) observed that the apparent volume of the CS/β-Gp hydrogels did not change within 31d in vitro. This hydrogel has potential applications as an insulin sustained release system after subcutaneous administration because of its stability and prolonged hypoglycemic effect.
Toxicity of the system
Since a therapeutic carrier must assure that no detrimental effect threatens the subject after its administration, the toxicity of CS/β-Gp was evaluated. This was done by analyzing the activity of ALP, amylase, AST and CPK enzymes 1-week post-subcutaneous injection of the formulation.
shows enzyme activity for the three groups [CS/β-Gp, CS/β-Gp/insulin and control group (n = 4)]. No significant differences were observed in the groups through 7 d of study, showing that the CS/β-Gp/insulin thermosensitive system had no toxic effects on enzyme activity. The low toxicity of CS/β-Gp hydrogel was also reported by several researchers (Zhou et al., Citation2011; Liu et al., Citation2014).
Conclusion
In this research, CS/β-Gp formulations were prepared and their thermosensitivity was investigated by evaluating changes in turbidity and rheologic properties. A dramatic increase in turbidity and elastic modulus was observed at the gelation point. In vitro insulin release was evaluated by changing both the insulin and β-Gp concentrations. It was found that the increase in insulin loading decreased the amount of insulin released in the medium. Insulin incorporation into the chitosan/β-Gp gel had no impact on insulin stability. Following a single subcutaneous injection of CS/β-Gp containing 8% (w/v) β-Gp and 0.01 mg/ml insulin into diabetic mice, the hypoglycemic effect lasted ∼5 d. CS/β-Gp/insulin had no significant effect on the activity of AST, CPK, ALP and amylase enzymes for 1-week post-injection. These results indicate that CS/β-Gp thermosensitive hydrogel is a promising insulin delivery system that avoids blood glucose fluctuations caused by daily insulin injections.
Declaration of interest
The authors report no conflicts of interest. The authors alone are responsible for the content and writing of this article.
References
- Abruzzo A, Bigucci F, Cerchiara T, et al. (2012). Mucoadhesive chitosan/gelatin films for buccal delivery of propranolol hydrochloride. Carbohyd Polym 87:581–8
- Ao Q, Fung CK, Tsui AY, et al. (2011). The regeneration of transected sciatic nerves of adult rats using chitosan nerve conduits seeded with bone marrow stromal cell-derived Schwann cells. Biomaterials 32:787–96
- Berrada M, Serreqi A, Dabbarh F, et al. (2005). A novel non-toxic campthotecin formulation for cancer chemotherapy. Biomaterials 26: 2115–20
- Casettari L, Vllasaliu D, Castagnino E, et al. (2012). PEGylated chitosan derivatives: synthesis, characterizations and pharmaceutical applications. Prog Polym Sci 37:659–85
- Cheng YH, Yang SH, Lin FH. (2011). Thermosensitive chitosan-gelatin-glycerol phosphate hydrogel as a controlled release system of ferulic acid for nucleus pulposus regeneration. Biomaterials 32:6953–61
- Cheng YH, Yang SH, Liu CC, et al. (2013). Thermosensitive hydrogel made of ferulic acid-gelatin and chitosan glycerophosphate. Carbohyd Polym 92:1512–19
- Chenite A, Buschmann M, Wang D, et al. (2001). Rheological characterization of thermogelling chitosan/glycerol-phopshate solutions. Carbohyd Polym 46:39–47
- Chenite A, Chaput C, Wang D, et al. (2000). Novel injectable neutral solutions of chitosan form biodegradable gels in situ. Biomaterials 21:2155–61
- Cho J, Heuzey MC, Begin A, Carreau PJ. (2005). Physical gelation of chitosan in the presence of beta-glycerophosphate: the effect of temperature. Biomacromolecules 6:3267–75
- Cooper A, Bhattarai N, Zhang M. (2011). Fabrication and cellular compatibility of aligned chitosan–PCL fibers for nerve tissue regeneration. Carbohyd Polym 85:149–56
- Ferrari PC, Souza FM, Giorgetti L, et al. (2013). Development and in vitro evaluation of coated pellets containing chitosan to potential colonic drug delivery. Carbohyd Polym 91:244–52
- Fu X, Zhang X, Chang Z. (2005). 4,4′-Dianilino-1,1′-binaphthyl-5,5′-sulfonate, a novel molecule having chaperone-like activity. Biochem Biophys Res Commun 329:1087–93
- Ganji F, Vasheghani-Farahani E. (2008). Hydrogels in controlled drug delivery systems. Iranian Polym J 18:1–26
- Hoemann CD, Hurtig M, Rossomacha E, et al. (2005). Chitosan-glycerol phosphate/blood implants improve hyaline cartilage repair in ovine microfracture defects. J Bone Joint Surg Am 87:2671–86
- Ji QX, Deng J, Xing XM, et al. (2010). Biocompatibility of a chitosan-based injectable thermosensitive hydrogel and its effects on dog periodontal tissue regeneration. Carbohyd Polym 82:1153–60
- Kempe S, Metz H, Bastrop M, et al. (2008). Characterization of thermosensitive chitosan-based hydrogels by rheology and electron paramagnetic resonance spectroscopy. Eur J Pharm Biopharm 68:26–33
- Kim S, Nishimoto SK, Bumgardner JD, et al. (2010). A chitosan/β-glycerophosphate thermo-sensitive gel for the delivery of ellagic acid for the treatment of brain cancer. Biomaterials 31:4157–66
- Li X, Kong X, Wang X, et al. (2010). Gel–sol–gel thermo-gelation behavior study of chitosan–inorganic phosphate solutions. Eur J Pharm Biopharm 75:388–92
- Liu X, Chen Y, Huang Q, et al. (2014). A novel thermo-sensitive hydrogel based on thiolated chitosan/hydroxyapatite/beta-glycerophosphate. Carbohyd Polym 110:62–9
- Ma G, Yang D, Li Q, et al. (2010). Injectable hydrogels based on chitosan derivative/polyethylene glycol dimethacrylate/N,N-dimethylacrylamide as bone tissue engineering matrix. Carbohyd Polym 79:620–7
- Molinaro G, Leroux JC, Damas J, Adam A. (2002). Biocompatibility of thermosensitive chitosan-based hydrogels: an in vivo experimental approach to injectable biomaterials. Biomaterials 23:2717–22
- Ngoenkam J, Faikrua A, Yasothornsrikul S, Viyoch J. (2010). Potential of an injectable chitosan/starch/β-glycerol phosphate hydrogel for sustaining normal chondrocyte function. Int J Pharm 391:115–24
- Obara K, Ishihara M, Ishizuka T, et al. (2003). Photocrosslinkable chitosan hydrogel containing fibroblast growth factor-2 stimulates wound healing in healing-impaired db/db mice. Biomaterials 24:3437–44
- Park H, Choi B, Nguyen J, et al. (2013). Anionic carbohydrate-containing chitosan scaffolds for bone regeneration. Carbohyd Polym 97:587–96
- Peng Q, Sun X, Gong T, et al. (2013). Injectable and biodegradable thermosensitive hydrogels loaded with PHBHHx nanoparticles for the sustained and controlled release of insulin. Acta Biomaterialia 9:5063–9
- Rao SB, Sharma CP. (1997). Use of chitosan as a biomaterial: studies on its safety and hemostatic potential. J Biomed Mater Res 34:21–8
- Richardson SM, Hughes N, Hunt JA, et al. (2008). Human mesenchymal stem cell differentiation to NP-like cells in chitosan–glycerophosphate hydrogels. Biomaterials 29:85–93
- Rokhade AP, Shelke NB, Patil SA, Aminabhavi TM. (2007). Novel interpenetrating polymer network microspheres of chitosan and methylcellulose for controlled release of theophylline. Carbohyd Polym 69:678–87
- Ruel-Gariépy E, Shive M, Bichara A, et al. (2004). A thermosensitive chitosan-based hydrogel for the local delivery of paclitaxel. Eur J Pharm Biopharm 57:53–63
- Short BG. (2008). Safety evaluation of ocular drug delivery formulations: techniques and practical considerations. Toxicol Pathol 36:49–62
- Tsai ML, Chang HW, Yu HC, et al. (2011). Effect of chitosan characteristics and solution conditions on gelation temperatures of chitosan/2-glycerophosphate/nanosilver hydrogels. Carbohyd Polym 84:1337–43
- Wu J, Su ZG, Ma GH. (2006). A thermo- and pH-sensitive hydrogel composed of quaternized chitosan/glycerophosphate. Int J Pharm 315:1–11
- Wu J, Wei W, Wang LY, et al. (2007). A thermosensitive hydrogel based on quaternized chitosan and poly(ethylene glycol) for nasal drug delivery system. Biomaterials 28:2220–32
- Yan J, Yang L, Wang G, et al. (2010). Biocompatibility evaluation of chitosan-based injectable hydrogels for the culturing mice mesenchymal stem cells in vitro. J Biomater Appl 24:625–37
- Zhao QS, Ji QX, Xing K, et al. (2009). Preparation and characteristics of novel porous hydrogel films based on chitosan and glycerophosphate. Carbohyd Polym 76:410–16
- Zheng L, Ao Q, Han H, et al. (2010). Evaluation of the chitosan/glycerol-β-phosphate disodium salt hydrogel application in peripheral nerve regeneration. Biomed Mater 5:35003–14
- Zhou HY, Zhou DJ, Zhang WF, et al. (2011). Biocompatibility and characteristics of chitosan/cellulose acetate microspheres for drug delivery. Front Mater Sci 5:367–78