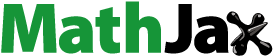
Abstract
Hydrogels are the three-dimensional network structures obtained from a class of synthetic or natural polymers which can absorb and retain a significant amount of water. Hydrogels are one of the most studied classes of polymer-based controlled drug release. These have attracted considerable attention in biochemical and biomedical fields because of their characteristics, such as swelling in aqueous medium, biocompatibility, pH and temperature sensitivity or sensitivity towards other stimuli, which can be utilized for their controlled zero-order release. The hydrogels are expected to explore new generation of self-regulated delivery system having a wide array of desirable properties. This review highlights the exciting opportunities and challenges in the area of hydrogels. Here, we review different literatures on stimuli-sensitive hydrogels, such as role of temperature, electric potential, pH and ionic strength to control the release of drug from hydrogels.
Introduction
The development of drug delivery system is based on the physicochemical and pharmacokinetic properties of the drug. Conventional delivery systems suffer from the limitations of minimal synchronization between the time required to achieve therapeutically effective plasma drug concentration and the actual drug release profile exhibited by the dosage form (Bruguerolle, Citation1998). With the ongoing research in advanced drug delivery formulations to provide stable and economical drug delivery systems, our focus is on the hydrogel systems which are known to reduce the problems of conventional dosage forms, molecules as small as non-steroidal anti-inflammatory drugs (NSAIDs) or as large as proteins and peptides (Graham & Mc-Neil, Citation1984; Bajpai & Saggu, Citation2007).
Initially, hydrogels was described as a colloidal gel of inorganic salts, which were highly different from the hydrogels, we are dealing with nowadays. At that time, the term “hydrogel” was used to describe a three-dimensional (3D) network of hydrophilic natural polymers and gums, in which the network is formed by chemical or physical crosslinking. These ordinary hydrogels were used only for swelling–deswelling process depending on the availability of water in the environment (Lee et al., Citation2013). The hydrogel of new generation was first developed by Witchterle & Lim (Citation1960). Applications of smart hydrogels have been divided into four areas, such as drug delivery, biosensor, bioseparation and tissue engineering. As the time passes, research on hydrogels for drug delivery has been focused on developing advanced drug delivery systems, such as self-regulated insulin delivery systems. With the advancement in the technology, hydrogels having numerous applications in tissue engineering have been developed. These systems are called smart hydrogels as they respond immediately to the environmental changes (Qiu & Park, Citation2001). A large number of studies based on the applications of smart hydrogels in drug delivery, nanotechnology and tissue engineering have been carried out in the recent decades.
These smart hydrogels are currently being developed as new drug delivery systems for various bioactive molecules due of their unique properties, which resembles to that of the living tissues. These unique properties are due to their high water content, soft and rubbery consistency and low interfacial tension with water and biological fluids (Dagani, Citation1997). These systems are so designed to ensure zero-order constant drug release over a prolonged period of time (Lowman & Peppas, Citation1991; Stastny et al., Citation2002). The characteristics like swelling behavior in aqueous medium, pH sensitivity, temperature sensitivity and zero-order kinetics play important role in the development of hydrogel-based drug delivery system.
They can be divided into two categories based on the chemical or physical nature of the crosslinked interactions. Crosslinking due to chemical interactions leads to permanent junction in the polymer network while physical interactions are either due to polymer chain entanglements or due to the physical interactions such as ionic interactions, hydrogen bonding or hydrophobic interactions (Jen et al., Citation1996). These structures imbibe water or biological fluids in large amount at least 10–20 times their molecular weight thus becomes swollen (Kim et al., Citation1992). Hydrogels that exhibit both liquid- and solid-like behavior have a variety of functional properties such as swelling, mechanical, permeation, surface and optical properties (Rossi et al., Citation1991).
In addition, stimuli-sensitive hydrogels have the unique property of undergoing abrupt volume changes from their collapsed and swollen states in response to environmental stimuli, which have both sensor and effector functions. Therefore, various stimuli-sensitive hydrogels that have been studied theoretically and experimentally which can respond to pH, temperature, electric field, ionic strength, solvent composition, pressure and other stimuli (Tanaka et al., Citation1980). The factors responsible for the drug release from the hydrogels are diffusion coefficient, porosity and tortuosity (Rowley et al., Citation1999; Liu et al., Citation2000; Aroca et al., Citation2007).
Various advantages of hydrogels as drug delivery system come from their good transport properties, ability to protect drugs, proteins and peptides from the environment, biodegradability and can be modified according to the route of administration. The high water content of these materials contributes to their better biocompatibility (Hejazi & Amiji, Citation2003). Sensor properties of hydrogels are responsible for their sol–gel phase transition behavior depending upon the environmental conditions. Because of having such unique properties, they are also referred to as “intelligent” or “smart” polymeric systems as they dictate not only the delivery of drugs, but also when and at which time interval it is being released from the system (Soppimath et al., Citation2002). The stimuli of the hydrogels are physical (temperature, electric fields, light, pressure and magnetic fields), chemical (pH and ions) or biological/biochemical ones, responsible for various responses (Qiu & Park, Citation2001). Hydrogels have been used in a variety of applications like wound healing, tissue engineering, gene delivery, ocular drug delivery, transdermal delivery and subcutaneous drug delivery in the form of implants. Different hydrogel preparation techniques along with their merits and demerits and various polymer for hydrogels are shown in and .
Table 1. Different hydrogel preparation techniques along with their merits and demerits.
Table 2. Various polymer for hydrogels along with their applications.
Types of hydrogels
Hydrogels are classified based on the nature of the stimuli responsible for the swelling behavior. Various stimuli-responsive hydrogels also have the ability for controlled and targeted delivery of drug and other bioactive molecules. Stimuli responsible for swelling of hydrogels are shown in . These hydrogels are discussed below.
pH-sensitive or ion-sensitive hydrogels
The hydrogels release the drug by changing the pH of the external environment. pH-sensitive polymers, e.g. polyvinyl amine containing either acidic or basic groups (Brannon & Peppas, Citation1991; Katchalsky & Michaeli, Citation1955). Poly acrylic acid (PAA; an anionic polymer) dissolves/swell more at high pH due to ionization whereas Poly(N,N′-diethylamino ethyl methacrylate (PDEAMA; a cationic polymer) swells more at low pH. The swelling of the polyelectrolyte is mainly due to the electrostatic repulsion between charges present in the polymer chain (Firestone & Siegel, Citation1991). Ionization on polyelectrolyte’s is more difficult due to the electrostatic interactions exerted by the other adjacent ionized groups present on the polymer chain. This can be minimized by using co-monomers like methyl methacrylate, 2-hydroxyethyl methacrylate and maleic anhydride (Kou et al., Citation1988; Brannon-Peppas & Peppas, Citation1990; Falamarzian & Varshosaz, Citation1998). A new kind of pH-sensitive hydrogel based on poly(lactic acid) (PLA), methoxyl poly(ethylene glycol) (MPEG) and itaconic acid (IA) system prepared by heat-initiated free radical polymerization was found as a safe candidate for drug delivery systems (Wang et al. Citation2012).
Takemoto et al. (Citation2010) performed the work on polyion complex in which first the preparation of poly(N-vinylacetamide)-co-poly(N-vinylformamide) (poly(NVA-co-NVF)) hydrogel was carried out, followed by hydrolysis reaction to produce a cationic polyvinyl amine (PVAm) layer on the surface of the hydrogel and then polymerization of polyacrylic acid (PAA) to the surface cationized hydrogel resulted in the formation of polyionic complex (PIC) hydrogel. Therefore, it can be said that the main determining factor for drug release depends on the thickness of the PIC layer having a continuous polymer network, and possesses different functions for the controlled release of drug molecules (Takemoto et al., Citation2010). Various drugs delivered through pH-responsive hydrogels are summarized in .
Table 3. Various drugs delivered through pH-responsive hydrogels for drug delivery.
Temperature-sensitive hydrogels
The mechanical characteristics of the hydrogel and of the drugs from the hydrogels are altered with the change in the temperature of external environment (Bromberg & Ron, Citation1998). The common characteristics of thermo-sensitive polymer are the presence of different hydrophobic groups (methyl, ethyl and propyl). Poly(N-iso-propylacrylamide) (PNIPAAm) and poly(N,N-diethylacrylamide (PDEAAm) are widely used polymers for temperature-sensitive hydrogel because of their lower critical solution and controlled drug delivery which is based on sol–gel phase conversion at the body temperature (Soppimath et al., Citation2002).
Negative thermo-sensitive hydrogels contract when heated to above their lower critical solution temperature (Bae et al., Citation1991). These hydrogels obtain on–off drug release profile from matrices with on at a lower temperature and off at a higher temperature (Katono et al., Citation1991). Positive thermo-sensitive hydrogels show swelling at higher temperature while shrinking at lower temperature (Schild, Citation1992). The lower critical solution temperature (LCST) or lower consolute temperature is the critical temperature below which the components of a mixture are miscible in all compositions. At temperatures below LCST, the system is completely miscible in all proportions, whereas above LCST partial liquid miscibility occurs.
If any hydrophobic group is present in the polymer chain then LCST becomes lower while if any hydrophilic group is present in the polymer, then LCST becomes higher (Feil et al., Citation1992).
The LCST can be changed by adjusting the ratio of hydrophilic and hydrophobic segments of the polymer or by forming co-polymers of hydrophobic (e.g. NIPAAm) and hydrophilic (e.g. acrylic acid) monomers (Hirotsu, Citation1993; Irie, Citation1993; Nazar et al., Citation2011). Recently, the potential use of arginine-based surfactants and ethyl(hydroxyethyl)cellulose produced low toxicity thermoresponsive hydrogel for pharmaceutical and biomedical applications. Thermo-sensitive hydrogels for nasal drug delivery was developed using N-trimethyl chitosan chloride as polymer. Poly(ethylene glycol) and glycerophosphate showed promising role, particularly in rheological and mucoadhesive behavior, and a sol–gel transition was achieved at 32.5 °C within 7 min (Langer, Citation1998). Various drugs delivered through temperature-responsive hydrogel are summarized in .
Table 4. Various drugs delivered through temperature-responsive hydrogels for drug delivery.
Glucose-sensitive hydrogels
These hydrogels are sugar sensitive and shows variable response depending upon the level of glucose. Glucose-sensitive hydrogels are useful for development of self-regulated delivery systems which can deliver the necessary amount of insulin in response to blood glucose concentration (Ishihara & Matsui, Citation1986; Albin et al., Citation1987). This can be achieved by incorporating glucose oxidase (GOD)-loaded hydrogels, lectin-loaded hydrogels and hydrogels with phenyl boronic acid moieties (Brownlee & Cerami, Citation1979).
Horbett and coworkers (Lowman & Peppas, Citation1991; Bajpai & Saggu, Citation2007) were the first to investigate system consisting of immobilized GOD in a pH-responsive polymeric hydrogel, enclosing a saturated insulin solution. In GOD-loaded hydrogels, GOD is combined with pH-sensitive hydrogels. An increase in the blood glucose level causes the glucose to diffuse into the membrane where glucose is converted into the gluconic acid, which lowers the pH resulting in swelling of hydrogels and the release of insulin. This system is gaining interest due to its release pattern which is similar to that of the endogenous release of insulin (Seminoff et al., Citation1989). Insulin release from glucose-sensitive hydrogels are shown in .
In lectin-loaded hydrogels, the unique carbohydrate-binding properties of lectins are very useful for the fabrication of glucose-sensitive systems. Such systems are developed by synthesizing the glycosylated insulin derivative to form complex with concanvalin A (lectin possessing four binding sites). The glycosylated insulin derivative is then released from its complex with Con A in the presence of free glucose, based on the competitive and complementary binding properties of glycosylated insulin and glucose to Con A. Another approach includes the use of phenylboronic acid moiety which forms complex with polyol compounds like glucose in aqueous solutions (Kim et al., Citation1990; Kitano et al., Citation1991; Takemoto et al., Citation2010). One of the types of phenylboronic acid moiety can be prepared by copolymerization of N-vinyl-2-pyrrolidone (NVP) and 3-(acrylamido) phenylboronic acid (PBA; Kitano et al., Citation1992). Due to the reversible complex formation between phenylboronic acid of poly (NVP-co-PBA) and poly(vinyl alcohol) the competitive binding of phenylboronic acid with glucose and PVA could be utilized to construct a glucose-sensitive system. The formation and dissociation of the poly(NVP-co-PBA)/PVA complex could be investigated by observing the change in the viscosity. It was observed that poly(NVP-co-PBA) formed a complex with PVA in the absence of glucose, however the complex dissociated in the presence of glucose. Thus, such systems can sensitize the glucose level and release the insulin. In other studies, self-assembled multilayered films were fabricated to modulate insulin release from the positively charged poly [2-(dimethylamino) ethyl methacrylate] (PDMAEMA) star polymer and negatively charged insulin and GOD (Yin et al., Citation2011). Glucose-responsive microhydrogels based on methacrylate derivatives of dextran and concanavalin A have been prepared for self-regulated insulin delivery without destroying the tertiary structure of insulin (Kataoka & Matsumoto, Citation1998).
Kataoka & Matsumoto (Citation1998) worked on a matrix of glucose-responsive gel using PNIPAAm and fraction of a phenylboronic acid group and it could cause a remarkable glucose-dependant change in swelling of hydrogel. It was observed that on–off regulation of insulin release from the gel was achieved through a drastic change in the solute transport property which was a result of the formation and disruption of the surface barrier layer of the gel (Matsumoto et al., Citation2012).
Matsumoto et al. (Citation2012) while working with 2,2′-azobisisobutyronitrile (AIBN) as an initiator in the presence of N,N′-methylene-bis (acrylamide) (MBAAm) as a crosslinking agent in DMSO reported that surface-controlled release of insulin can be achieved as gel can form a skin layer which provide a rationale for glucose-sensitive release under glucose homeostasis conditions (Podual et al., Citation2000a,Citationb). Various polymers used for glucose-sensitive drug delivery are summarized in .
Table 5. Various glucose sensitive drug delivery systems along with their applications.
Alginate-calcium ion hydrogels
Sodium alginate forms ionic hydrogel upon contact with divalent cations, e.g. calcium due to the physical crosslinking (chelation) between the carboxylate anions of guluronate units in alginate and the calcium ions. Kikuchi et al. (Citation1997) proposed that alginate dissolution is due to the calcium ion release from the hydrogels and integrated this concept with the pulsatile release of macromolecular dextran. Further different parameters like alginate concentration, alginate molecular weight or alginate gel bead diameter influences the onset time for the release of dextran (Okor et al., Citation1991; Iskakov et al., Citation2002).
Isakov and group worked on fluorescein isothiocyanate (FITC) dextran (MW 9400, 71 200, 145 000) pulsatile release from alginate-calcium ion beds coated with poly(carboxy-n-propylacrylamide-co-dimethyl acrylamide) of varying thickness (25–125 µm), and found that FITC-dextran release is strongly dependant on copolymer coat thickness and molecular weight, which effect the onset release time which got modulated from 2 to 60 h for dextran (MW 145 000) having 125-mm thick copolymer coated beads (Kuo & Ma, Citation2001).
Later on, Kuo and group worked on ionically crosslinked alginate hydrogels and control the alginate gelation rate. Both (higher or lower) gelation rate have their impact as faster gelation rate is preferable for better cell encapsulation, while lower gelation rate results in uniform structural and mechanical integrity. Further, it was found that the gelation rate of hydrogel can be controlled by various factors such as calcium content, polymer concentration and gelation temperature (Chen et al., Citation1997).
Chen et al. (Citation2011b) developed a novel natural semi-interpenetrating polymer network (semi-IPN) composed of chitosan crosslinked with glutaraldehyde and silk fibroin. They investigated the pH and ion sensitivity of this network on the system. The FTIR spectra of the semi-IPN showed that the chitosan and silk fibroin had a strong H-bond interaction and formed an interpolymer complex. This chitosan/silk fibroin semi-IPN showed good ion sensitivity. The semi-IPN exhibited good swelling when put alternatively into different pH buffer solutions, which is due to the formation and dissociation of H-bonding within the network. Due to the reversible behavior of semi-IPN, it can also be used as an “artificial muscle” because of its swelling–shrinking nature at different pH (Park & Hoffman, Citation1993). Park and co-workers determined the swelling behavior of non-ionic PNIPAAm) hydrogel in various salts solutions. This non-ionic poly(NIPAAm) gel exhibited a sharp volume phase transition at a critical concentration of sodium chloride in aqueous solution. The critical concentration depends on the temperature changes, however, other salts tested did not cause such an abrupt volume change, which may be due to the reason that NIPAAm exhibits a LCST phenomenon in aqueous solution. This gel collapses and shrinks above the LCST and re-swells and expands below that critical temperature (Balasubramaniam et al., Citation2003). Thus, it was observed that hydrogels exhibit a different degree of swelling in response to different types of salts along with their concentrations. Balasubramaniam and group developed ion-activated in situ gelling systems for sustained ophthalmic delivery of ciprofloxacin HCl and concluded that the formulated systems provided sustained release of the drug over a period of 8 h in vitro (Sultana et al., Citation2006). Sultana and co-workers developed an ion-activated, gelrite based in situ ophthalmic gels of pefloxacin mesylate and compared with conventional eye drops and concluded that this system was capable of effective and controlled management of conjunctivitis (Liu et al., Citation2010). Liu et al. in 2010 investigated in situ gelling Gelrite/Alginate formulations as vehicles for ophthalmic delivery and found that the optimum concentration of gelrite solution for in situ gel-forming delivery systems was 0.3% (w/w) and that for alginate solution was 1.4% (w/w; Vodithala et al., Citation2010). The (mixture of 0.2% Gelrite and 0.6% alginate) solution showed a significant enhancement in gel strength at physiological condition. Vodithala et al. (Citation2010) developed ion-activated ocular in situ gels of ketorolac tromethamine using gelrite as a polymer and concluded that the developed formulations showed sustained release of drugs for up to 6 h. The formulations were found to be non-irritating with no ocular damage (Khoylou & Naimian, Citation2009).
Preparation of hydrogels
The most common method of preparation of hydrogel-based drug product has been showed in . The various crosslinked networks of synthetic polymers such as polyethylene oxide (PEO) (Razzak et al., Citation2001), polyvinyl pyrrolidone (PVP; Palumbo et al., Citation2006), polylactic acid (Onuki et al., Citation2008), polyacrylic acid (PAA; Yang et al., Citation2009), polymethacrylate (Singh & Vashishth, Citation2008) polyethylene glycol (PEG; Peppas & Khare, Citation1993) or natural biopolymers, such as hyaluronic acid, pectin, chondroitin sulfate, alginic acid, carrageenan have been prepared using different techniques like solution polymerization or crosslinking (Fei et al., Citation2000), irradiation crosslinking (Hennink & Nostrum, Citation2002; Liu et al., Citation2002), physical crosslinking (Yoshii & Kume, Citation2003), suspension polymerization and grafting polymerization (Witchterle & Lim, Citation1960).
Solution polymerization/crosslinking
In solution polymerization, ionic or neutral monomers are mixed with multifunctional crosslinking agent. Polymerization can be initiated thermally by UV light or by redox initiators. Solvents are incorporated to serve as heat sink and to minimize the temperature control problems. Unreacted monomers, initiators and crosslinking agent are removed by the use of distilled water (Morishta et al., Citation2002). This method can be used for preparing either pH-sensitive or temperature-sensitive hydrogels using methacrylic acid (Zhang & Wu, Citation2002) or N-isopropylacrylamide (Lugao & Malmonge, Citation2001), respectively, as the monomer.
Crosslinking by irradiation
Radiation crosslinking technique is widely used, since it does not involve the use of chemical additives and therefore retains the biocompatibility of the biopolymer (Peppas et al., Citation1986). The technique mainly relies on producing free radicals in the polymer following the exposure to the high-energy source, such as gamma ray, X-ray or electron beam. The radiolysis of water molecules using radiations results in the formation of hydroxyl radicals, which attacks the polymer chain to form macroradicals. Recombination of macroradicals on different chains results in the formation of covalent bonds that ultimately leads to crosslinking (Shu & Zhu, Citation2002). This whole procedure is carried out in the presence of inert atmosphere or nitrogen as macroradicals can interact with oxygen.
Physical crosslinking
Limitations of covalent crosslinking can be overcome by reversible ionic crosslinking (Torre et al., Citation2003). Ionic polymers can be crosslinked by the addition of di- or tri-valent counter ions. This method relies on the principle of gelling a polyelectrolyte solution (e.g. Na+ alginate) with a multivalent ion of opposite charge (e.g. Ca2+ 2Cl−), e.g. chitosan polycationic polymer can form complex with poly(acrylic acid). This polyelectrolyte complex undergoes slow erosion, which produce a more biodegradable material than covalently crosslinked hydrogels (Noble et al., Citation1999; Berger et al., Citation2004). In contrast to covalent coupling, there is no additional requirement of catalyst (Spinelli et al., Citation2008).
Grafting polymerization
Grafting involves the polymerization of a monomer on the backbone of a preformed polymer and these polymer chains are activated by the action of various chemical reagents and high-energy irradiations. The production of functional monomers on these activated macroradicals leads to branching and then to crosslinking (). Starch grafted with acrylic acid by using N-vinyl-2-pyrrolidone is an example of chemical grafting (Said et al., Citation2004) whereas the preparation of hydrogel of CMC by grafting CMC with acrylic acid in the presence of electron beam in aqueous solution is an example for the formation of hydrogels using high-energy radiation. The electron beam in radiation treatment is used to initiate the free radical polymerization of acrylic acid on the backbone of CMC. The water radiolysis product can also be helpful to abstract proton form macromolecular backbones. Irradiation of both CMC and monomer leads to produce free radicals, which combine to produce hydrogels (McMullan, Citation2005).
Characterization of hydrogels
Morphological characterization
Scanning electron microscopy
Hydrogels can be characterized for their morphology by the use of scanning electron microscopy (SEM). It provides the two-dimensional images which are six-order magnified images of a sample under vacuum (Zworykin et al., Citation1942; Omidian et al., Citation2005). SEM is also used to determine the surface topology, composition and electrical conductivity of the dried samples. SEM has significance as it can determine the pore size and pore structure of building block which affects swelling and further allows controlled release of the bioactive material from the hydrogels.
Atomic force microscopy
It is a very high-resolution type of scanning probe microscopy having an advantage over scanning electron microscopy as it provides 3D surface profile (Janshoff et al., Citation2002). The mechanism involves the approaching of the tip of the probe to the surface of the material results in a decrease in amplitude of the oscillation due to increased interaction between the surface and the tip. This oscillation can be compared with an external reference which provides information on the surface characteristics of the material (Harasaki et al., Citation2001; Geisse, Citation2009). Atomic force microscopy can work well with an ambient air and even in a liquid environment which makes it possible to study biological macromolecules and even living organisms (Alarcon et al., Citation2005).
Chemical/physical characterization
The functional groups play a significant role in the water holding capacity of the hydrogel. By modification of functional groups, the properties of hydrogels can be improved. The characterization based on these groups can be carried out using techniques like UV–visible spectroscopy, infrared (IR) spectroscopy, mass spectrometry and nuclear magnetic resonance (NMR; Mansur et al., Citation2008). Mansur and co-workers carried out Fourier transform infrared spectroscopy (FTIR) for characterization of Polyvinyl alcohol–glutaraldehyde crosslinked hydrogels and found vibrational band between 2840 and 3000 cm−1 which refer to the stretching C–H from alkyl groups and the peaks between 1750–1735 cm−1 are due to the stretching of C=O and C–O from acetate group. The considerable reduction of the intensity of the O–H peaks from the hydrogel indicates the formation of acetal bridges (Kumar et al., Citation2007).
Swelling characterization
The polymer chains in a hydrogel interact with the solvent molecule (usually water) and tend to expand to the fully solvated state, while the crosslinked structure applies a retractive force to pull the chains inside (Wang et al., Citation2010). Equilibrium is achieved when these expanding and retracting forces counter balance each other. Swelling studies can be carried out by immersing completely dried hydrogels in swelling medium and latter the hydrogel is removed from the solution and weighed after an excessive solution on the surface is blotted (Bhattarai et al., Citation2010). The swelling characteristics are crucial to the use of hydrogels in biomedical and pharmaceutical applications since the equilibrium-swelling ratio influences the solute diffusion coefficient, surface mobility, optical and mechanical properties of the hydrogel (Tang et al., Citation2007). The swelling properties are determined by many factors, including the type and composition of monomers, crosslinking density and other environmental factors (temperature, pH and ionic strength; Nicodemus & Bryant, Citation2008).
The swelling ratio is calculated by the following equation:
where Q is the swelling ratio, Ms and Md are the mass of hydrogel in the swollen state and in the dried state, respectively.
The water content of the hydrogels can be determined as the water loss of fully swollen polymer at different time intervals as a function of the time of exposure at 37 °C (Serafim et al., Citation2013).
The water content is determined as a function of time by this equation:
where Ww is the weight of water and Wg is the weight of gel.
Rheological characterization
The rheological properties are very much dependant on properties of hydrogels like association, entanglement and crosslinking agent present in hydrogels (Kheirandish et al., Citation2009). Viscous polymer solutions follow the law G′ ∼ ω2 and G″ ∼ ω where G′ and G″ represents shear storage modulus and shear loss modulus, respectively, while ω represents the angular velocity. Elasticity dominates in case when G′ > G″ which represents the Maxwell-type behavior having single relaxation time and this behavior increases with the increase in concentration (Weng et al., Citation2007). Weng et al. carried out rheological measurements for in situ crosslinking hydrogels formulated from oxidized dextran and N-carboxyethyl chitosan and characterized the network structure in hydrogel (Zhang & Ma, Citation1999).
Porosity and density characterization
The solvent replacement is the main method for determination of porosity, which involves the immersion of dried hydrogels overnight in absolute ethanol and weighed after blotting of excess ethanol on the surface (Shi et al., Citation2002; Polnok et al., Citation2004).
The porosity can be calculated from the following equation:
where M1 and M2 are the mass of the hydrogel before and after immersion in absolute ethanol, respectively, ρ is the density of absolute ethanol and V is the volume of the hydrogel.
The solvent displacement method is used to carry out the density determination of dried hydrogels, which actually show the apparent densities of the hydrogels. This method involves the weighing of dried hydrogels and then these hydrogels are immersed in a predetermined volume of hexane and the increase in the hexane volume was measured as the volume of the polymer (Chang, Citation1988).
The density can be calculated using the following equation:
where Mh is mass of hydrogels and V is the volume of solvent displaced by hydrogels.
Determination of void fraction
Void fraction can be defined as the measure of empty void spaces in the hydrogel. It is a fraction of the volume of voids over the total volume of hydrogels (Chen et al., Citation2000). The void fraction inside hydrogels can be determined by immersing the hydrogels in swelling media up to equilibrium and this volume can be determined as the dimensional volume. Total volume of pores can be determined by subtracting the weight of dried hydrogel from the weight of swollen hydrogel (Gorgieva & Kokol, Citation2012).
The void fraction can be calculated using the following formula:
where Vd is dimensional volume of the hydrogel and Vt is the total volume of pores.
In vitro weight loss profile
In vitro weight loss profile of the hydrogels describes the change in weight as a function of time. Dried hydrogels in the desired swelling medium are used for sampling which firstly includes washing with distilled water, and then drying in vacuum oven until constant weight is obtained (Chiu et al., Citation2013). The percentage weight loss can be calculated using this formula:
where Wt(i) is the initial weight of hydrogel at time zero and Wt(t) is the weight of the hydrogel at time t.
Mechanical characterization
The mechanical strength mainly depends on the composition and structure of the hydrogels. These hydrogels possess weak mechanical strength in fully swollen state due to the high water content (Nicodemus & Bryant, Citation2008). The mechanical properties of the hydrogels are affected by the degree of swelling, co-monomer composition, polymerization conditions and crosslinking density (Mauck et al., Citation2006). Bench compactor can be used for testing the mechanical properties of the hydrogels (Jaiswal & Koul, Citation2011). Mechanical characterization can also be used to determine the multi-components of the hydrogels (Kazakia et al., Citation2006).
Most common methods used to determine the mechanical properties of hydrogels include tensile testing or strip extensiometry. In strip extensiometry technique, tensile force is applied to the strips of material held between two grips. In ring extensiometry, tensile force can be applied to a ring instead of a single strip. Thus, the shear-strain chart can be obtained between the applied force and elongation of the material. This chart can be used to find out different mechanical properties of the hydrogels, including Young’s modulus, yield strength and ultimate tensile strength. Extensiometry can also be used to examine the viscoelastic nature of a hydrogel material by elongating the material strip to a particular length and examining the stress relaxation response over time at a constant strain. Compression is another technique used to examine the mechanical properties of hydrogel. It involves placing the material between two plates and compressing it. Pressure is applied to the hydrogel surface to calculate the mechanical properties of the hydrogel using a theoretical model. The main advantage of compression test over extensiometry is that it does not limit the hydrogel geometry to strips or rings (Svensson et al., Citation2005).
Mechanical properties of the hydrogels could be affected by the type of crosslinking agent and density (Drury & Mooney, Citation2003). Zhang and co-workers worked on the development of collagen–chondroitin sulfate–hyaluronic acid hybrid hydrogel (CCH) scaffolds. Their results showed that the compressive modulus of the crosslinked hydrogels increased from 44.93 to 53.67 kPa with the increase in concentration of genipin (crosslinking agent) up to 1 mM. Results of compression showed that the compressive modulus of non-crosslinked CCH hydrogels was slightly greater than that of collagen hydrogels, which may be due to the compressive behavior of the negatively charged glycosaminoglycans (GAGs). It is based on the theory that water molecules attracted more towards the negative charges of the GAGs, which leads to the increase in compressive capabilities of the tissue (Almarza & Athanasiou, Citation2004; Chao et al., Citation2007). In addition, the concentration of genipin greatly affects the compressive modulus and shows a slow increase in compressive modulus from 44.93 to 53.67 kPa with the increase of genipin concentration. They also found that pure collagen was sufficient to provide mechanical strength to hydrogel. Thus, biological and mechanical properties of collagen hydrogel could be increased by blending hydrogel with suitable polymers [i.e. poly(glycolic acid), poly(lactic-co-glycolic acid) and chitosan], and by adding a suitable chemical crosslinking agent, which simultaneously affect the swelling properties of the hydrogels (Zhang et al., Citation2011). In the market, a large number of hydrogel-based products are being used ().
Table 6. Currently available hydrogels based products in the market.
Applications
Hydrogels are being used in a variety of applications ranging from drug delivery to tissue engineering applications. They have gained importance due to their ability to be delivered through different routes like transdermal, subcutaneous, implantable, ocular, rectal, vaginal, pulmonary, dermal and nasal routes (Junginger, Citation1991; Nagai & Machida, Citation1993; Park & Park, Citation1996).
Wound healing
Hydrogels act as a moist wound dressing material and have ability to absorb and retain the wound exudates along with the foreign bodies (bacteria etc.) within its network structure. In addition, hydrogels have been found to promote fibroblast proliferation by reducing the fluid loss from the wound surface and protect the wound from external impurities which have great impact for rapid wound healing and maintains the microclimate for biosynthetic reactions which is necessary for normal cellular activities (Lopez & Bodmeier, Citation1997).
Hydrogels carry out fibroblast proliferation and keratinocyte migration which are necessary for the epithelialization of the wound. Hydrogel sheets are generally applied over the wound surface with backing of fabric or polymeric film and are secured at the wound surface by the use of adhesives or bandages (Ueno et al., Citation2001). Dextran-based hydrogels are prepared to promote neovascularization and skin regeneration in a third-degree burn wounds (Sun et al., Citation2011). The covalently bound heparin (heparin sulfate proteoglycan) is a multivalent biomaterial which can be used for controlled release of basic fibroblast growth factor (bFGF; ).
Tissue engineering
Tissue engineering (TE) is a multidisciplinary approach for the development of biological substitutes. The principles of TE have been used extensively to restore functions of the traumatized/malfunctioning tissues or organs by combining the patient’s cells with a scaffold for generating new tissues (Hoffman, Citation2002; Hench & Jones, Citation2005). The pore size of the scaffolds should be optimized, as it is necessary for the cell migration into the core of the scaffolds, angiogenesis and supply of nutrients to the cells and to take away the metabolic products away from the cells (Pal et al., Citation2008). By tissue engineering, ideally, patient-derived cells can be cultured in vitro and combined with hydrogel matrix to form a 3D scaffold, fabricated by simple molding technique or more advanced bioprinting procedures ().
Figure 6. Schematic flow chart of basic principle of tissue engineering using hydrogels as extracellular matrix.
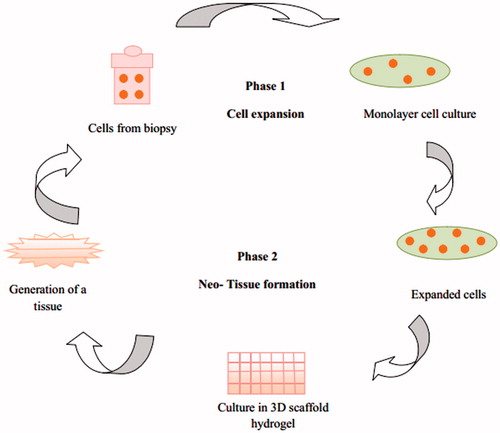
Microfabrication techniques are potentially powerful tools in tissue engineering since they can be used to replicate structures which are in the order of 0.1–10 mm. Microengineered hydrogels are classified as either “top down” or “bottom up”. Top down approach utilizes microengineering approaches to control the microscale features of relatively large pieces of hydrogels. This approach includes designing and micromolding of the shapes of the scaffolds (Borenstein et al., Citation2002; Fidkowski et al., Citation2005). In the bottom-up approach, fabrication by the assembly of smaller building blocks is carried out. This approach mimics much of the native biology that is often made from repeating functional units.
For example, the sinusoid is the repeating functional unit found in the liver. Bottom-up approaches can be used to generate functional units that can be assembled in a modular approach to generate larger scaffolds (McGuigan & Sefton, Citation2006).
Tissue printing is an emerging approach that can also be used to form tissues from smaller building blocks. Using tissue printing, it is possible to generate microvasculature and desired architecture in tissues (Mironov et al., Citation2003). Although a number of challenges (ejector clogging and poor tissue mechanics) have limited the current applications of this technology. It is anticipated that through further research the tissue printing will become a powerful “bottom-up” approach to engineer complex 3D tissues.
Colon specific drug delivery
Colon specific hydrogels of polysaccharides have been specifically designed because of the presence of high concentration of polysaccharidase enzymes in the colon region of GI (gastrointestinal) tract. Drugs loaded in such hydrogels show tissue specificity and change in the pH or enzymatic actions causes the release of the drugs (Calejo et al., Citation2012). Stomach-specific antibiotic delivery for the treatment of Helicobacter pylori infection in peptic ulcer is a good example of such system (Patel & Amiji, Citation1996).
Gene delivery
Most plasmid DNA delivery systems based on synthetic polymers can lead to in vitro degradation of biodegradable polymer for more than 30 days (). Gene delivery generally mediated by viral and non-viral methods. But, viral vectors are quite limited as they can produce immunogenic reactions or mutagenesis of transfected cells therefore, after the relevance of non viral approaches scientist put interest to involve the use of polymers viz. poly-l-lysine (PLL), PGA, PLA and PLGA for gene delivery (Choi & Park, Citation2005). The use of PEG–PLGA–PEG hydrogel for the delivery of plasmid-beta 1 gene increased the wound healing process in a diabetic mouse model (Lee et al., Citation2003).
Further, researchers reported the use of recombinant silk-elastin like polymer hydrogels (SELP) for the delivery of pRL-CMV for the treatment of human breast cancers (Mageed et al., Citation2004). These results suggest that an increase in the transfection efficiency when SELP hydrogels were used. Inclusion of basic fibroblast growth factor (BFGF), insulin growth factor II (IGF-II) and collagen within the microcapsules showed proliferation and differentiation of encapsulated C2C12 myoblasts cell lines. When tested against tumor induced by B16-Fo/neu tumor cells in mice, the alginate polylysine alginate (APA) microcapsules showed 80% reduction in tumor volume at day 21 (Li et al., Citation2006). Lentiviral gene therapy vectors can be entrapped within fibrin hydrogels alone or in complex form with hydroxylapatite (HA) nanoparticles, which provides an opportunity to enhance the bioactivity of fibrin hydrogels for a wide range of applications in regenerative medicine (Kidd et al. Citation2011). Gene delivery of hydrogels incorporating biodegradable polymer is shown in .
Ocular drug delivery
In conventional ocular drug delivery, there are various constraints like effective drainage by tear, blinking and low permeability of the cornea, short-term retention of the drugs and in suspensions and ointments, the unpleasant irritation feeling made scientists to take interest on the hydrogels for ocular drug delivery. The in situ gelling system is prepared using alginate with high gluronic acid content for the ophthalmic delivery of pilocarpine. This system significantly extended the duration of action of pilocarpine to 10 h compared to pilocarpine nitrate as solution which was only 3 h of duration of action (Cohen et al., Citation1997). Nowadays, silicone hydrogel therapeutic lenses are considered a place for their use in recurrent corneal erosions, irregular corneas, keratoconus as bandage lenses (Stapleton et al., Citation2006).
Transdermal delivery
The possible benefits of hydrogels in transdermal drug delivery include (a) drugs can be delivered for a long duration at a constant rate, (b) can be easily interrupted on demand by simply removing the devices and (c) can by-pass hepatic first-pass metabolism and also due to higher water content, swollen hydrogels can provide a better environment for the skin in comparison to conventional ointments and patches. The hydrogels obtained from the copolymerization of bovine serum albumin (BSA) and PEG succeeded as transdermal hydrogels due to their high water content (over 96%) which allow the release of both hydrophilic and hydrophobic drugs. Their use as novel drug formulation in the field of wound dressing was proposed as the potential application of the BSA–PEG hydrogels (Gayet & Fortier, Citation1996). The experimentation was carried out to design a practically controlled-release transdermal system for selegiline using thermo-sensitive hydrogels using copolymers of alginate and pluronic F127 which lead to the reduction in the intersubject variability of skin permeation (Chen et al., Citation2011a).
Subcutaneous delivery
Hydrogels show their applications in implantable therapeutics. Subcutaneous exogenous inserted materials may evoke undesirable responses, such as inflammation, carcinogenicity and immunogenicity. Hydrogels are considered as biocompatible materials due to their high water content. They also provide several promising properties such as (i) minimal mechanical irritation upon in vivo implantation, due to their soft, elastic properties; (ii) prevention of protein adsorption and cell adhesion due to low interfacial tension between water and hydrogels; (iii) broad acceptability for individual drugs with different hydrophilicity and molecular sizes; and (iv) unique possibility (crosslinking density and swelling) to manipulate the release of incorporated drugs (Sinha & Khosla, Citation1998). Several hydrogel formulations for the subcutaneous delivery of anticancer drugs have also been proposed, e.g. PHEMA in crosslinked form when applied with cystabine (Ara-C) and methotrexate showed good biocompatibility (Beyssac et al., Citation1996; Teijon et al., Citation1997). Poly(AAm-co-monomethyl or monopropylitaconate) developed by Blanco’s group was employed for the controlled release of Ara-C and 5-fluorouracil (Blanco et al., Citation1998). The subcutaneous reservoir hydrogel implant were prepared using copolymer of 2-hydroxyethyl methacrylate (HEMA) and are of great interest due to biocompatibility, reversible swelling in aqueous environment, resistance to biodegradation and microbial growth (Kuzma et al., Citation2011).
Biomedical applications of stimuli-responsive polymers in novel drug delivery
Stimuli-responsive polymers are those that respond sharply to small changes in physical or chemical conditions. These polymers are also known as “environmentally sensitive”, “smart” or “intelligent” polymers. They have numerous biomedical applications, especially in the field of drug delivery, cell culture surfaces and in diagnosis. These stimuli-responsive polymers may also be combined with a variety of bioactive molecules, such as nucleic acids, proteins and peptides, small organic molecules and carbohydrates, e.g. poly(ethylene glycol) (PEG) may be conjugated to with the poly(lactic-co-glycolic acid) [PLGA] to provide “stealth” properties. The example of most common smart polymer is poly(N-isopropyl acrylamide), or PNIPAAm which is thermally responsive smart polymer. Some of these polymers may respond to more than one stimulus, such as a copolymer of NIPAAm and acrylic acid (AAc), which is responsive to both temperature and pH. Similarly, copolymers of pH-sensitive methacrylic monomers such as methacrylic acid (MAAc) and hydrophobic methacrylate monomers, such as methyl methacrylate (MMA) are used to prepare enteric-coated tablets. They released the drug in the intestine where the pH rises to physiologic pH levels. These polymeric coatings are useful for protecting “fragile” drugs from stomach acid and gastric enzymes (Hoffman, Citation2013). Vernon and group developed tri-block copolymers, mixed them with drugs and injected sub-cutaneously or intra-muscularly to form phase separated, degradable, drug depot masses at body conditions. These tri-block copolymers were composed of alternating ABA or BAB blocks such as PLGA–PEG–PLGA or PEG–PLGA–PEG blocks and were thermally gelling, hydrolytically degradable in nature. These tri-block polymers released the drug slowly by dissolution and diffusion of the drug (Vernon et al., Citation2000). These smart polymers have also been designed to escape intracellular uptake by the macrophage. Ethyl acrylic acid (EAAc) and propyl acrylic acid (PAAc) form pH-sensitive polymers and copolymers which become sharply hydrophobic as the pH is lowered through their pKs, which is within the pH range of early endosomes. When Ethyl acrylic acid (EAAc) and propyl acrylic acid (PAAc) that form pH-sensitive polymers are conjugated or complexed with drugs and endocytosed into target cells, they can disrupt the lipid bilayer of the endosome as pH drops within the endosome, enhancing the escape of the polymer–drug carrier into the cytosol (Yin et al., Citation2006).
These stimuli-responsive polymers are steadily increasing attention of many scientists in the biomedical applications, especially in the fields of controlled and self-regulated drug delivery due to their close resembles with the normal physiological process of the disease state, thus ensuring optimum drug release as per physiological need (Bawa et al., Citation2009). These polymers have many advantages in drug delivery because they experience rapid changes in their structure triggered by small changes in the outer environment. One of the polymer named poly(NIPAAm-co-AAm) copolymers was used by Li and group to develop Gold (Au) nanocages for the controlled release using high-intensity focused ultrasound (HIFU). They modified the surface of gold nanocages using thermally responsive copolymer, poly(NIPAAm-co-AAm) (NIPAAm: N-isopropylacrylamide; AAm: acrylamide) by means of gold-thiolate linkage. These copolymers lead to conformational changes in response to temperature changes at a transition point known as LCST. This conformational change with temperature change control the drug release by altering the duration in which the polymer chains are kept in the high-temperature state. When a significant amount of acoustic energy is applied to the focus using HIFU, the temperature in the focal volume of the sample increases rapidly. As the temperature rises beyond the LCST of the copolymer, the polymer chains change from a stretched conformation to a collapsed state. This leads to the opening of the pores of the gold nanocages resulting in the drug release. However, when HIFU is turned off, the temperature drops to its original state and the polymer chains relax back to their extended conformation, blocking their pores and thus terminating the drug release. Thus, they successfully developed a platform for HIFU-induced, localized and controlled drug release from the Au nanocages covered with thermally responsive polymers (Li et al., Citation2011).
In the recent years, stimuli-responsive nanogels also known as polymeric nanoparticles are reported by many researchers that are capable of responding to external stimuli by changing their physico-chemical properties, such as volume, water content, refractive index, permeability and hydrophilicity–hydrophobicity. As compared to other polymeric nanoparticles, these stimuli-responsive nanogels provide advantages of high stability, prolonged circulation time in the blood stream, high encapsulation efficacy, controlled as well as site-specific drug release modulated by environment stimuli. These applications of stimulus-responsive nanogels make them an active participant, rather than a passive carrier for drug delivery (Zha et al., Citation2011).
With the advancement in drug delivery application, self-folding polymeric containers are introduced recently to encapsulate a variety of therapeutic cargos such as small molecules, peptides, proteins, bacteria, fungi and mammalian cells. These self-folding polymeric containers contain a thin film or interconnected planar templates curve, roll-up or fold into 3D structures such as cylindrical tubes, spirals, corrugated sheets or polyhedra. These self-folding polymeric containers are important for drug delivery applications since they provide a means to realize 3D, biocompatible, well-tailored composition, size, shape, wall thickness, porosity, surface patterns and chemistry (Fernandes & Gracias, Citation2012).
Recently, Malachowski et al. (Citation2014) proposed a thermo-responsive multi-fingered drug eluting devices. Their aim was to design such tissue gripping drug delivery devices that offer an effective strategy for sustained release of drugs with immediate applicability in the gastrointestinal tract. These were prepared using rigid poly(propylene fumarate) segments and stimuli-responsive poly(N-isopropylacrylamide-co-acrylic acid) hinges and are referred to as theragrippers. The stimuli-responsive polymer allows them to close above 32 °C thus provide a spontaneously gripping onto tissue when introduced from a cold state into the body. They studied the release potential of theragrippers loaded with fluorescent dyes and commercial drugs such as mesalamine and doxorubicin and found an enhanced release as compared to a control batch (Malachowski et al. Citation2014).
Yang et al. (Citation2014) developed a new type of triple-stimuli-responsive (ultrasound/pH/GSH) biodegradable nanocapsules for intravenous drug delivery, which is filled with perfluorohexane, and the DOX-loaded PMAA with disulfide crosslinking. These soft biodegradable nanocapsules with uniform size of 300 nm can easily enter the tumor tissues by ensuring diagnostic and image-guided therapeutic applications using Ultrasound contrast agents (UCAs), thus provide applications in echogenic intravenous drug delivery (Yang et al., Citation2014). If such stimuli-responsive polymers could show significantly enhanced delivery of drugs such as siRNA, they might be utilized in clinical testing in the near future.
Limitations of hydrogels as drug delivery systems
Low mechanical strength is the main problem with the use of hydrogel based drug delivery systems. In some cases, leaching of the drug may result (Patil et al., Citation2011).
They are difficult to handle (Peppas & Colombo, Citation1997).
Drug is very difficult to load in hydrogel-based drug delivery systems (Amsden, Citation1998).
Sterilization is the main problem with the use of these delivery systems (Arndt et al., Citation2004).
Swelling of temperature-sensitive hydrogels affected greatly by changes in the temperature of swelling medium (Mason et al., Citation2001).
Polymer complex can be broken or the network can be swollen as a result of changes in external environment (Bromberg & Ron, Citation1998).
Monomers and crosslinkers used in the synthesis of the hydrogels are not biocompatible, i.e. they may be toxic, carcinogenic or teratogenic (Chiu et al., Citation1999).
One of the major limitation of pH-sensitive polymers is their non-biodegradability. Hydrogels made of non-biodegradable polymers have to be removed from body after use. Non-biodegradability is a serious issue in case of implantable drug delivery agents or implantable biosensors (Beckert & Al, Citation1970).
Glucose-sensitive hydrogel systems do not retain their original states fast enough after responding to the change in glucose concentration (Qiu & Park, Citation2001).
Hydrogel suffers from problem of reproducibility (Qiu & Park, Citation2001).
Electro-sensitive hydrogel-based drug delivery systems cannot work properly under physiological conditions and cannot modulate the drug release in a manner in which it is required (Qiu & Park, Citation2001).
Reaction of hydrogels in response to stimulus (light) is very slow. In many cases, the conversion of light into thermal energy must precede the restructuring of polymer chains upon temperature change, which leads to leaching of content during swelling–deswelling cycles (Qiu & Park, Citation2001).
Clinical trials based on hydrogel-based drug delivery
A short-term clinical trial for the prevention of Peristomal Infection after Percutaneous Endoscopic Gastrectomy (PEG) was done by Blumenstein and group which was based on the development of glycerin-based wound dressing hydrogel. Glycerin hydrogel (GHG) wound dressing system has been proposed with more effective antimicrobial properties. The main purpose of this study was to check the superiority of GHG regarding the incidence of peristomal wound infections during a 30-day post-procedure follow-up. Sixty-eight patients with cancer undergoing PEG were selected from one university and two general hospitals between January 2007 and December 2008. Patients were randomized into the following groups: group 1 includes 34 patients who received GHG and group 2 includes 34 patients who received a traditional wound dressing. In group 1, dressing were changed at day 1 and weeks 1, 2 and 4. In group 2, dressings were changed daily during the first week and at weeks 2 and 4. Infectious site (PEG site) was assessed using two different infection scores. A statistically significant reduction in the mean infection score was observed in patients of group one (first week: 1.64 ± 1.6 versus 3.12 ± 2.69, p < 0.008; second week: 1.37 ± 1.11 versus 2.53 ± 2.37, p < 0.02). After 1 week, 14.7% wound reactions occurred in group 1 as compared to 47.05% wound reactions in group 2, i.e. traditional group (p < 0.005). It was observed that GHG wound dressing significantly reduced the peristomal wound infections and proved itself as convenient, cost-effective alternative for wound management following PEG (Blumenstein et al., Citation2012) and favored approximately five times less frequent dressing change. Another clinical trial was done on a patient-operated microwave system based on hydrogel contact lenses. Clinical effects of a patient-operated system of microwave disinfection of soft contact lenses were checked in a prospective pilot trial involving 103 patients who were taken from five optometric practices. Fifty-six subjects used the test system for a period of 1 month and 13 subjects used the test system for a total period of 3 months. Slit-lamp test was used to examine the clinical signs in both test and control subjects. After 1 month, the incidence of all signs reported in the microwave group was not significantly found greater than in the control group (p = 0.267), and results were the same after 3 months (p = 0.214). There was a significantly greater incidence of edema in the 1-month test group and of staining in the control group. UV spectroscopic examination of worn lenses from test subjects exhibiting significant signs did not show a higher level of deposition than on lenses worn by control (p = 0.397) subjects (Crabbe & Thompson, Citation2001). A clinical trial of recombinant human granulocyte-macrophage colony-stimulating factor (rhGM-CSF) hydrogel for the treatment of deep second-degree burns was carried out. The efficacy and safety of a rhGM-CSF hydrogel to promote deep-second-degree burn wound healing was evaluated. In this multicenter, randomized, double-blind and placebo-controlled clinical trial, a total of 90 patients with deep second-degree burns were randomly divided into two groups. Complete healing time and percentage of wound healing at different time points was observed and side effects of the developed system were recorded. A statistically significant difference was observed at each time point (p < 0.01). However, no side effects were observed. These results showed that rhGM-CSF hydrogel significantly improved and accelerate deep second-degree burn wound healing, thus considered safe for use (Zhang et al., Citation2009). A number of patents have been compiled on stimuli-responsive hydrogels for drug delivery applications in the last few decades ().
Table 7. Recent patent on stimuli-responsive hydrogels for drug delivery applications.
Future scope
The design and synthesis of “smart” hydrophilic polymers and hydrogels has significant potential in biomedical and nanotechnology applications in future. The future success of these materials relies on the development of novel materials that can address specific biological and medical challenges. This development will occur through synthesis of new polymers or by modifying natural polymers. The desired tissue used as model to engineer their desired mechanical, chemical and biological properties into the hydrogel (Lee & Mooney, Citation2001) for tissue engineering. All over the world, the universities and scientific organizations are currently looking into ways of growing functioning heart cells on the heart to replace the tissue that dies when a heart attack occurs by using scaffolds consisted of materials, such as carbon nanofibers and gold nanowires to grow new heart tissue without the need for surgery (Zhang et al., Citation2011).
Glucose-sensitive hydrogels have the ability to mimic the normal endogenous insulin secretion that minimizes the diabetic complications like cardiovascular disorders; retinopathy and nephropathy as well as improve patient compliance as it can release the bioactive compound in a controlled manner and when it is required by the body. Therefore, this delivery system may play promising role in future (Obaidat & Park, Citation1996). Silicone hydrogel lenses have made even further progress towards a lens of first choice. Five silicone hydrogel lens types are now available which are recently launched at the American Academy of Optometry (AAO). Moreover, number of silicone hydrogel daily wearers over the year, increasing daily confirming that the majority of patients can be refitted from conventional hydrogel lens wear to silicone hydrogel daily wear with excellent results (Dumbleton et al., Citation2010). Researchers at Stanford have created a new conducting polymer hydrogel that features high electrochemical activity and can be easily deposited onto surfaces using an ink-jet printer or simply sprayed on. Therefore, these electrically conductive hydrogel may open new opportunities for medical sensors and implants (Pan et al., Citation2012). Hydrogels attached with adhesion molecules like polylysine (polylysine-modified hydrogels) which are brain mimetic materials for neural tissue engineering has a bright future as no any delivery system or natural phenomenon have the ability to regenerate the neurons (Rao et al., Citation2011). The above examples represent some of the approaches which can improve the efficiency of delivery system and can also involve regenerating the tissues as well as the neurons. The main advantageous feature of the hydrogel is that they can deliver the drugs with controlled rate and can be applied through all of the routes which bring attention to work upon it.
Conclusion
Revolutionary advancement has been done in the last few decades for the development of drug delivery system. With the advancement of novel drug delivery systems, hydrogel drug delivery system has further corroborated the link between therapeutic need and drug delivery. The main critical parameters required to be monitored are hydrophobicity/hydrophilicity of the polymer which have great impact on release rate and dissolution rate. The stimuli responses like pH, temperature and glucose levels are monitored to attain the controlled and site specific delivery which ultimately ensures achievement of better patient compliance. The most significant weakness of all these stimuli-responsive system is that their response time is too slow. Thus, the fast acting hydrogels are necessary and easier way to achieve this goal to make thinner and smaller hydrogels. Further advancement involves the synthesis of new polymers and crosslinking agents ensuring significant controlled release. The main success of hydrogel can be attained by high level of in vitro–in vivo correlation between their performance and ability to maintain the structural integrity in biological systems. If the achievements of the past can be extrapolated into future, then there will be high possibility to achieve newer trends in the field of novel drug delivery.
Acknowledgements
The authors are grateful to the ISF College of pharmacy for providing all kinds of support and motivation to carry out this work.
Declaration of interest
The authors report no conflicts of interest.
References
- Alarcon CLH, Pennadam S, Alexander C. (2005). Stimuli responsive polymers for biomedical applications. Chem Soc Rev 34:276–85
- Albin GW, Horbett TA, Miller SR, Ricker NL. (1987). Theoretical and experimental studies of glucose sensitive membranes. J Control Release 6:267–91
- Almarza A, Athanasiou K. (2004). Design characteristics for the tissue engineering of cartilaginous tissues. Ann Biomed Eng 32:2–17
- Amsden B. (1998). Solute diffusion within hydrogels. Mechanisms and models. Macromolecules 31:8382–95
- Arndt KF, Schmidt T, Richter A, Kuckling D. (2004). High response smart gels: synthesis and application. Macromol Symp 207:257–68
- Aroca AS, Ribelles JL, Pradas MM, et al. (2007). Characterization of macroporouspolymethyl methacrylate coated with plasma polymerized poly 2-hydroxyethyl acrylate. Eur Polym J 43:4552–64
- Bae YH, Okano T, Kim SW. (1991). On–off’ thermo control of solute transport. I. Temperature dependence of swelling of N-isopropyl acrylamide networks modified with hydrophobic components in water. Pharm Res 8:531–7
- Bajpai SK, Saggu SS. (2007). Insulin release behaviour of poly (methacrylamide-co-N-vinyl-2-pyrrolidone–co-itaconic acid) hydrogel, an interesting probe. J Macromol Sci A Pure Appl Chem 44:153–7
- Balasubramaniam J, Kant S, Pandit JK. (2003). In vitro and in vivo evaluation of the Gelrite® gellan gum-based ocular delivery system for indomethacin. Acta Pharm 53:251–61
- Bawa P, Pillay V, Choonara YE, du Toit LC. (2009). Stimuli-responsive polymers and their applications in drug deliver. Biomed Mater 4:022001
- Beckert WH, Al E. (1970). Mitogenic activity of the jack bean with rabbit peripheral blood lymphocytes. Int Arch Allergy Appl Immunol 30:337–41
- Berger J, Reist M, Mayer JM, et al. (2004). Structure and interactions in covalently and ionically crosslinked chitosan hydrogels for biomedical applications. Eur J Pharm 57:19–34
- Betancourt T, Pardo J, Soo K, Peppas NA. (2010). Characterization of pH-responsive hydrogels of poly(itaconic acid-g-ethylene glycol) prepared by UV-initiated free radical polymerization as biomaterials for oral delivery of bioactive agents. J Biomed Mater Res A 93:175–88
- Beyssac E, Bregni C, Aiache JM, et al. (1996). Hydrogel implants for methotrexate obtained by ionizing radiation. Drug Dev Ind Pharm 2:439–44
- Bhattarai N, Gunn J, Zhang M. (2010). Chitosan-based hydrogels for controlled, localized drug delivery. Adv Drug Deliver Rev 62:83–99
- Blanco MD, Gomez CO, Teijoan JM. (1998). Ara-C release from poly(acrylamide-co-monomethylitaconate) hydrogels: in vitro and in vivo studies. Polym Gels Netw 6:57–69
- Blumenstein I, Borger D, Loitsch S, et al. (2012). A glycerin hydrogel-based wound dressing prevents peristomal infections after percutaneous endoscopic gastrostomy (PEG). Nutr Clin Pract 27:422–5
- Borenstein JT, Terai H, King KR, et al. (2002). Microfabrication technology for vascularized tissue engineering. Biomed Microdev 4:167–75
- Brannon L, Peppas NA. (1991). Equilibrium swelling behavior of pH-sensitive hydrogels. Chem Eng Sci 46:715–22
- Brannon-Peppas L, Peppas NA. (1990). Dynamic and equilibrium swelling behaviour of pH-sensitive hydrogels containing 2-hydroxyethyl methacrylate. Biomaterials 11:635–44
- Bromberg LE, Ron ES. (1998). Temperature-responsive gels and thermogelling polymer matrices for protein and peptide delivery. Adv Drug Deliv Rev 31:197–221
- Brownlee M, Cerami A. (1979). Glucose-controlled insulin delivery system: semisynthetic insulin bound to lectin. Science 206:1190–1
- Bruguerolle B. (1998). Chronopharmacokinetics current status. Clin Pharmacokinet 35:83–94
- Cahalan PT, Coury AJ, Jevne AH, Kallok MJ. (1988). Hydrogel adhesive. US Patent US4768523A
- Calejo MT, Kjoniksen AL, Pinazo A, et al. (2012). Thermoresponsive hydrogels with low toxicity from mixtures of ethyl (hydroxyethyl) cellulose and arginine-based surfactants. Int J Pharm 436:454–62
- Chang CS. (1988). Measuring density and porosity of grain kernels using a gas pycnometer. Cereal Chem 65:13–5
- Chao P, Grayson W, Vunjak-Novakovic G. (2007). Engineering cartilage and bone using human mesenchymal stem cells. J Orthop Res 12:398–404
- Chen CC, Fang CL, Suwayeh AS, et al. (2011a). Transdermal delivery of selegiline from alginate-Pluronic composite thermogels. Int J Pharm 415:119–28
- Chen G, Ushida T, Tateishi T. (2002). Development of biodegradable porous scaffolds for tissue engineering. J Mater Sci Eng C 17:63–9
- Chen J, Blevins WE, Park H, Park K. (2000). Gastric retention properties of superporous hydrogel composites. J Control Release 55:12–5
- Chen X, Guo Z, Xin J, Li J. (2011b). Modulated insulin release from glucose-sensitive multilayer films. J Control Release 152:152–4
- Chen X, Li W, Zhong W, et al. (1997). Ion sensitivity of hydrogels based on complex-forming chitosan/silk fibroin interpenetrating polymer network. J Appl Polym Sci 65:2257–62
- Chiu HC, Hsiue GH, Lee YP, Huang LW. (1999). Synthesis and characterization of pH-sensitive dextran hydrogels as a potential colon-specific drug delivery system. J Biomater Sci Polym 10:591–608
- Chiu YC, Kocagoz S, Larson JC, Brey EM. (2013). Evaluation of physical and mechanical properties of porous poly (ethylene glycol)-co-(L-lactic acid) hydrogels during degradation. PLoS One 9:607–28
- Choi JS, Park JS. (2005). Design elements of polymeric gene carrier. In: Mahato R, ed. Biomaterials for delivery and targeting of proteins and nucleic acids. Boca Raton: CRC Press, 643–62
- Chudzik SJ. (2012). Hydrogel-based joint repair system and method. US Patent US8242179 B2
- Chung HJ, Lee Y, Park TG. (2008). Thermo-sensitive and biodegradable hydrogels based on stereocomplexed pluronic multi-block copolymers for controlled protein delivery. J Control Release 127:22–30
- Cohen S, Lobel E, Trevgoda A, Peled T. (1997). A novel in situ-forming ophthalmic drug delivery system from alginates undergoing gelation in the eye. J Control Release 44:201–8
- Crabbe A, Thompson P. (2001). Clinical trial of a patient-operated microwave care system for hydrogel contact lenses. Optom Vis Sci 78:605–9
- Creque HM, Langer R, Folkman J. (1980). One month of sustained release of insulin from a polymer implant. Diabetes 29:37–40
- Dagani R. (1997). Intelligent gels. Chem Eng News 75:26–36
- Dong LC, Allan S, Hoffman A. (1991). Novel approach for preparation of pH-sensitive hydrogels for enteric drug delivery. J Control Release 15:141–52
- Drury JL, Mooney DJ. (2003). Hydrogels for tissue engineering: scaffold design variables and applications. Biomaterials 24:4337–51
- Dufresne MH, Garrec DL, Sant V, et al. (2004). Preparation and characterization of water-soluble pH sensitive nanocarriers for drug delivery. Int J Pharm 277:81–90
- Dumbleton K, Woods C, Jones L, et al. (2010). Comfort and vision with silicone hydrogel lenses: effect of compliance. Optom Vis Sci 87:131–9
- Falamarzian M, Varshosaz J. (1998). The effect of structural changes on swelling kinetics of polybasic/hydrophobic pH sensitive hydrogels. Drug Dev Ind Pharm 24:667–9
- Fernandes R, Gracias DH. (2012). Self-folding polymeric containers for encapsulation and delivery of drugs. Adv Drug Deliv Rev 64:1579–89
- Fei B, Wach RA, Mitomo H, et al. (2000). Hydrogel of biodegradable cellulose derivatives. I. Radiation-induced crosslinking of CMC. J Appl Polym Sci 78:278–83
- Feil H, Bae YH, Kim SW. (1992). Mutual influence of pH and temperature on the swelling of ionizable and thermosensitive hydrogels. Macromolecules 25:5528–30
- Fidkowski C, Mofrad MRK, Borenstein J, et al. (2005). Endothelialized microvasculature based on a biodegradable elastomer. Tissue Eng 11:302–9
- Firestone BA, Siegel RA. (1991). Kinetics and mechanisms of water sorption in hydrophobic, ionizable copolymer gels. J Appl Polym Sci 42:901–14
- Gayet JC, Fortier G. (1996). High water content BSA-PEG hydrogel for controlled release device: evaluation of the drug release properties. J Control Release 38:177–84
- Geisse NA. (2009). AFM and Combined Optical Techniques. Mater Today 12:40–5
- Gong J, Osada Y. (2004). Low friction hydrogel having straight chain polymers and method for preparation. US Patent US20040116305 A1
- Gorgieva S, Kokol V. (2012). Preparation, characterization and in-vitro enzymatic degradation of chitosan-gelatine hydrogel scaffolds as potential biomaterials. J Biomed Mater Res A 100:1655–67
- Graham NB, Mc-Neil M. (1984). Hydrogels for controlled drug delivery. Biomaterials 5:27–36
- Gupta AK, Maurya SD, Dhakkar RC, Singh RD. (2010). pH sensitive interpenetrating hydrogel for eradication of Helicobacter pylori. Int J Pharm Sci 3:924–32
- Harasaki A, Schmit J, Wyant JC. (2001). Offset of coherent envelope position due to phase change on reflection. Appl Optics 40:2102–6
- Hejazi R, Amiji M. (2003). Chitosan-based gastrointestinal delivery systems. J Control Release 89:151–65
- Hench LL, Jones JR. (2005). Biomaterials, artificial organs and tissue engineering. 2nd ed. Cambridge, UK: Woodhead Publishing Limited, 227
- Hennink WE, Nostrum CF. (2002). Novel crosslinking methods to design hydrogels. Adv Drug Deliv Rev 54:13–36
- Hinrichs WL, Suhuurmans NM. (1999). Thermosensitive polymers as carrier for DNA delivery. J Control Release 60:249–59
- Hirotsu S. (1993). Coexistence of phases and the nature of first-order phase transition in poly (N-isopropylacrylamide) gels. Adv Polym Sci 110:1–26
- Hoffman AS. (2002). Hydrogels for biomedical applications. Adv Drug Deliv Rev 54:3–12
- Hoffman AS. (2013). Stimuli-responsive polymers: biomedical applications and challenges for clinical translation. Adv Drug Deliv Rev 65:10–6
- Irie M. (1993). Stimuli-responsive poly (N-isopropylacrylamide). Photo and chemical-induced phase transitions. Adv Polym Sci 110:49–65
- Ishihara K, Matsui K. (1986). Glucose-responsive insulin release from polymer capsule. J Polym Sci Polym Lett 24:413–7
- Iskakov RM, Kikuchi A, Okano T. (2002). Time-programmed pulsatile release of dextran from calcium alginate gel beads coated with carboxy-n-propylacrylamide copolymers. J Control Release 80:57–68
- Jaiswal M, Koul V. (2011). Assessment of multicomponent hydrogel scaffolds of poly(acrylic acid-2-hydroxy ethyl methacrylate)/gelatin for tissue engineering applications. J Biomater Appl 1:1–14
- Janshoff A, Neitzert M, Obersofer Y, Fuchs H. (2002). Force spectroscopy of molecular systems, single molecular spectroscopy of polymers and macromolecules. Angew Chem Int Ed 39:3212–37
- Jen AC, Wake MC, Mikos AG. (1996). Review: hydrogels for cell immobilization. Biotechnol Bioeng 50:357–64
- Junginger HE. (1991). Mucoadhesive hydrogels. Pharm Ind 53:1056–65
- Kang SI, Bae YH. (2003). A sulfonamide based glucose-responsive hydrogel with covalently immobilized glucose oxidase and catalase. J Control Release 86:115–21
- Kataoka K, Matsumoto A. (1998). Total synthetic polymer gels responding to external glucose concentration: their preparation and applications to on-off regulation of insulin release. J Am Chem Soc 120:12694
- Katchalsky A, Michaeli I. (1955). Polyelectrolyte gels in salt solution. J Polym Sci 15:69–86
- Katono H, Maruyama A, Sanui K, et al. (1991). Thermo-responsive swelling and drug release switching of interpenetrating polymer networks composed of poly-butyl methacrylate) and poly(acrylic acid). J Control Release 16:215–27
- Kazakia GJ, Nauman EA, Ebenstein DM, et al. (2006). Effects of in vitro bone formation on the mechanical properties of a trabeculated hydroxyapatite bone Substitute. J Biomed Mater Res A 77:688–99
- Kheirandish S, Guybaidullin I, Wohlleben W, Willenbacher N. (2009). Shear and elongational flow behavior of acrylic thickener solutions. Part I: effect of intermolecular aggregation. Rheol Acta 4:397–407
- Khoylou F, Naimian F. (2009). Radiation synthesis of superabsorbent polyethylene oxide/tragacanth hydrogel. Radiat Phys Chem 78:195–8
- Kidd EM, Shin S, Shea DL. (2011). Fibrin hydrogel for lentiviral gene delivery in vitro and in vivo. J Control Release 157:80–5
- Kikuchi A, Kawabuchi M, Sugihara M, et al. (1997). Pulsed dextran release from calcium-alginate gel beads. J Control Release 47:21–9
- Kim JJ, Park K. (2001). Modulated insulin delivery from glucose-sensitive hydrogel dosage forms. J Control Release 77:39–47
- Kim SW, Bae YH, Okano T. (1992). Hydrogels: swelling, drug loading and release. Pharm Res 9:283–90
- Kim SW, Pai CM, Makino K, et al. (1990). Self-regulated glycosylated insulin delivery. J Control Release 11:193–201
- Kitano S, Kataoka K, Koyama Y, et al. (1991). Glucose-responsive complex formation between poly(vinyl alcohol) and poly(N-vinyl-2-pyrrolidone) with pendent phenylboronic acid moieties. Makromol Chem Rapid Commun 12:227–33
- Kitano S, Koyama Y, Kataoka K, et al. (1992). A novel drug delivery system utilizing a glucose responsive polymer complex between poly (vinyl alcohol) and poly(N-vinyl-2-pyrrolidone) with a phenylboronic acid moiety. J Control Release 19:162–170
- Kou JH, Amidon GL, Lee PI. (1988). pH-dependent swelling and solute diffusion characteristics of poly (hydroxyethyl methacrylate-co-methacrylic acid) hydrogels. Pharm Res 5:592–7
- Kumar A, Srivastava A, Galaev IY, Mattiasson B. (2007). Smart polymers: physical forms and bioengineering applications. Prog Polym Sci 32:1205–37
- Kuo CK, Ma PX. (2001). Ionically crosslinked alginate hydrogels as scaffolds for tissue engineering: part 1. Structure, gelation rate and mechanical properties. Biomaterials 22:511–21
- Kuzma P, Young AJM, Mora D, et al. (2011). Subcutaneous hydrogel reservoir system for controlled drug delivery. Macromol Symp 109:15–26
- Langer R. (1998). Drug delivery and targeting. Nature 392:5–10
- Lee KY, Mooney DJ. (2001). Hydrogels for tissue engineering. Chem Rev 101:1869–77
- Lee PY, Li Z, Huang L. (2003). Thermosensitive hydrogel as a Tgf-beta 1 gene delivery vehicle enhances diabetic wound healing. Pharm Res 20:1995–2000
- Lee SC, Kwon K, Park K. (2013). Hydrogels for delivery of bioactive agents: a historical perspective. Adv Drug Deliv Rev 65:17–20
- Li AA, Shen F, Zhang T, et al. (2006). Enhancement of myoblast microencapsulation for gene therapy. J Biomed Mater Res B 77:296–306
- Li W, Cai X, Kim C, et al. (2011). Gold nanocages covered with thermally-responsive polymers for controlled release by high-intensity focused ultrasound. Nanoscale 3:1724–30
- Liu P, Zhai M, Li J, et al. (2002). Radiation preparation and swelling behavior of sodium carboxymethyl cellulose hydrogels. Radiat Phys Chem 63:525–8
- Liu Q, Hedberg EL, Liu Z, et al. (2000). Preparation of macroporous poly 2-hydroxyethylmethacrylate hydrogels by enhanced phase separation. Biomaterials 21:2163–9
- Liu Y, Liu J, Zhang X, et al. (2010). In situ gelling gelrite/alginate formulations as vehicles for ophthalmic drug delivery. AAPS PharmSciTech 11:610–20
- Lopez CR, Bodmeier R. (1997). Mechanical water uptake and permeability of crosslinked chitosan glutamate and alginate films. J Control Release 44:215–25
- Lowman AM, Peppas NA. (1991). Hydrogels. In: Mathiowitz E, ed. Encyclopedia of controlled drug delivery. New York: Wiley, 397–418
- Lugao AB, Malmonge SM. (2001). Use of radiation in the production of hydrogels. Nucl Instrum Methods Phys Res Set B 185:37–42
- Mageed Z, Haider M, Li D, et al. (2004). In vitro and in vivo evaluation of recombinant silk-elastin like hydrogels for cancer gene therapy. J Control Release 94:33–45
- Malachowski K, Breger J, Kwag HR, et al. (2014). Stimuli-responsive theragrippers for chemomechanical controlled release. Angew Chem Int Ed Engl. doi: 10.1002/anie.201311047. [Epub ahead of print]
- Mansur HS, Sadahira CM, Souza AN, Mansur AP. (2008). FTIR spectroscopy characterization of poly (vinyl alcohol) hydrogel with different hydrolysis degree and chemically crosslinked with glutaraldehyde. Mater Sci Eng 28:539–48
- Mason MN, Metters AT, Bowman CN, Anseth KS. (2001). Predicting controlled-release behaviour of degradable PLA-b-PLZ hydrogels. Macromolecules 34:4630–5
- Matsumoto A, Ishii T, Nishida J, et al. (2012). A synthetic approach toward a self-regulated insulin delivery system. Angew Chem Int Ed Engl 51:2124–8
- Mauck RL, Yuan X, Tuan RS. (2006). Chondrogenic differentiation and functional maturation of bovine mesenchymal stem cells in long-term agarose culture. Osteoarthr Cartil 14:179–89
- McGuigan AP, Sefton MV. (2006). Vascularized organoid engineered by modular assembly enables blood perfusion. Proc Natl Acad Sci USA 11:461–6
- McMullan D. (2005). Scanning electron microscopy 1928–1965. Scanning 3:175
- Mironov V, Boland T, Trusk T, et al. (2003). Organ printing: computer-aided jet-based 3D tissue engineering. Trends Biotechnol 10:157–61
- Morishta M, Lowman AM, Takayama K, et al. (2002). Elucidation of the mechanism of incorporation of insulin in controlled release systems based on complexation polymers. J Control Release 81:25–32
- Nagai T, Machida Y. (1993). Buccal delivery systems using hydrogels. Adv Drug Deliver Rev 11:179–91
- Nazar H, Fatouros DG, Merwe SM, et al. (2011). Thermosensitive hydrogels for nasal drug delivery: the formulation and characterisation of systems based on N-trimethyl chitosan chloride. Eur J Pharm Biopharm 77:225–32
- Nicodemus GD, Bryant SJ. (2008). Cell encapsulation in biodegradable hydrogels for tissue engineering applications. Tissue Eng B Rev 14:149–65
- Noble L, Gray AI, Sadiq L, Uchegbu IF. (1999). Chitosan and sodium alginate-based bioadhesive vaginal tablets. Int J Pharm 192:173–82
- Obaidat AA, Park K. (1996). Characterization of glucose dependant gel-sol phase transition of the polymeric glucose-concanvalin A hydrogel system. Pharm Res 13:989–5
- Okor RS, Otimenyin S, Ijeh I. (1991). Coating of certain matrix cores with aqueous-based systems of acrylate methacrylate, a water-insoluble copolymer and drug release profiles. J Control Release 16:349–54
- Omidian H, Rocca JG, Park K. (2005). Advances in super porous hydrogels. J Control Release 102:3–12
- Onuki Y, Nishikawa M, Morishita M, Takayama K. (2008). Development of photocrosslinked polyacrylic acid hydrogel as an adhesive for dermatological patches: involvement of formulation factors in physical properties and pharmacological effects. Int J Pharm 349:47–52
- Pal K, Bag S, Pal S. (2008). Development of porous ultra high molecular weight polyethylene scaffolds for the fabrication of orbital implant. J Porous Mater 15:53–9
- Palumbo FS, Pitarresi G, Mandracchia D, et al. (2006). New graft copolymers of hyaluronic acid and polylactic acid: synthesis and characterization. Carbohydr Polym 66:379–85
- Pan L, Yu G, Zhai D, et al. (2012). Hierarchical nanostructured conducting polymer hydrogel with high electrochemical activity. Cambridge, MA: Harvard University Press, 1–6
- Park H, Park K. (1996). Biocompatibility issues of implantable drug delivery systems. Pharm Res 13:1770–6
- Park TG, Hoffman AS. (1993). Sodium chloride-induced phase transition in non-ionic Poly (N-isopropylacrlyamide) gel. Macromolecules 26:5045–8
- Patel VR, Amiji MM. (1996). Preparation and characterization of freeze dried Chitosan-poly(ethylene oxide) hydrogels for site-specific antibiotic delivery in the stomach. Pharm Res 13:588–93
- Patil SA, Rane BR, Bakliwal SR, Pawar SP. (2011). Pragmatic hydrogels. IJRAP 2:758–66
- Peppas NA, Colombo P. (1997). Analysis of drug release behaviour from swellable polymer carriers using the dimensionality index. J Control Release 45:35–40
- Peppas NA, Khare AR. (1993). Preparation, structure and diffusional behavior of hydrogels in controlled-release. Adv Drug Deliver Rev 11:1–35
- Peppas NA, Mikos AG, Peppas NA. (1986). Preparation methods and structure of hydrogels. In: Peppas NA, Mikos AG, eds. Hydrogels in medicine and pharmacy. Volume I. Boca Raton, FL: CRC Press, 2–23
- Podual K, Doyle FJ, Peppas NA. (2000a). Glucose-sensitivity of glucose oxidase-containing cationic copolymer hydrogels having poly (ethylene glycol) grafts. J Control Release 67:9–17
- Podual K, Doyle FJ, Peppas NA. (2000b). Preparation and dynamic response of cationic copolymer hydrogels containing glucose oxidase. Polymer 41:3975–83
- Polnok A, Verhoef JC, Borchard G, et al. (2004). In vitro evaluation of intestinal absorption of desmopressin using drug-delivery systems based on superporous hydrogels. Int J Pharm 269:303–10
- Qiu Y, Park K. (2001). Environment-sensitive hydrogels for drug delivery. Adv Drug Deliv Rev 53:321–39
- Rao SS, Ha N, Winter JO. (2011). Polylysine-modified PEG-based hydrogels to enhance the neuro-electrode interface. J Biomater Sci Polym 22:611–25
- Razzak MT, Darwis D, Sukirno Z. (2001). Irradiation of polyvinyl alcohol and polyvinyl pyrrolidone blended hydrogel for wound dressing. Radiat Phys Chem 62:107–13
- Rossi DD, Kanjiwara K, Osada Y, Yamauchi A. (1991). Polymer gels-fundamentals and biomedical applications. J Adhesion 37:271–2
- Rowley J, Madlambayan G, Faulkner J, Mooney DJ. (1999). Alginate hydrogels as synthetic extracellular matrix materials. Biomaterials 20:45–53
- Said HM, Alla SG, Naggar WMEL. (2004). Synthesis and characterization of novel gels based on carboxymethyl cellulose/acrylic acid prepared by electron beam irradiation. React Funct Polym 61:397–404
- Schild HG. (1992). Poly (N-isopropylacrylamide): experiment, theory and application. Prog Polym Sci 17:163–249
- Seminoff LA, Olsen GB, Kim SW. (1989). A self-regulating insulin delivery system. I. Characterization of a synthetic glycosylated insulin derivative. Int J Pharm 54:241–9
- Serafim A, Dragusin DM, Zecheru T, et al. (2013). Gelatin hydrogels: effect of ethylene oxide based synthetic crosslinking agents on the physico-chemical properties. Dig J Nanomater Bios 8:101–10
- Shi GX, Cai Q, Wang CY, et al. (2002). Fabrication and biocompatibility of cell scaffolds of poly(L-lactic acid) and poly(L-lactic-co-glycolic acid). Polym Adv Technol 13:227
- Shu XZ, Zhu KJ. (2002). Controlled drug release properties of ionically cross-linked chitosan beads: influence of anion structure. Int J Pharm 233:217–25
- Singh B, Vashishth M. (2008). Development of novel hydrogels by modification of sterculia gum through radiation cross-linking polymerization for use in drug delivery. Nucl Instrum Methods B 266:2009–20
- Sinha VR, Khosla L. (1998). Bioabsorbable polymers for implantable therapeutic systems. Drug Dev Ind Pharm 24:1129–38
- Soppimath KS, Aminabhavi TM, Dave AM, et al. (2002). Stimulus-responsive “smart” hydrogels as novel drug delivery systems. Drug Dev Ind Pharm 28:957–74
- Spinelli LS, Aquino AS, Lucas E, et al. (2008). Adsorption of polymers used in drilling fluids on the inner surfaces of carbon steel pipes. Polym Eng Sci 48:1885–91
- Stapleton F, Stretton S, Papas E, et al. (2006). Silicon hydrogel contact lenses and the ocular surface. Ocul Surf 4:24–43
- Stastny M, Plocova D, Etrych T, et al. (2002). HPMA-hydrogels containing static drugs. Kinetics of the drug release and in-vivo efficacy. J Control Release 81:101–11
- Sultana Y, Aqil M, Ali A. (2006). Ion-Activated, Gelrite based in situ ophthalmic gels of pefloxacin mesylate: comparison with conventional eye drops. Drug Deliv 13:215–9
- Sun G, Zhang X, Shen YI, et al. (2011). Dextran hydrogel scaffolds enhance angiogenic responses and promote complete skin regeneration during wound healing. Annu Rev 108:26976–81
- Svensson A, Nicklasson E, Harrah T, et al. (2005). Bacterial cellulose as a potential scaffold for tissue engineering of cartilage. Biomaterials 26:419–31
- Takemoto Y, Ajiro H, Asoh TA, Akashi M. (2010). Fabrication of surface-modified hydrogels with polyion complex for controlled release. Chem Mater 22:2923
- Tanaka T, Fillmore D, Sun ST, et al. (1980). Phase transitiononic gels. Phys Rev Lett 45:1636–9
- Tang C, Yin L, Yu JC, Pei Y. (2007). Swelling behavior and biocompatibility of carbopol-containing superporous hydrogel composites. J Appl Polym Sci 104:2785–91
- Teijon JM, Trigo RM, Garco O, Blanco MD. (1997). Cytarabine trapping in poly(2-hydroxyethyl methacrylate) hydrogels: drug delivery studies. Biomaterials 18:383–8
- Torre PM, Enobakhre Y, Torrado G, Torrado S. (2003). Release of amoxicillin from polyionic complexes of chitosan and poly (acrylic acid). Study of polymer/polymer and polymer/drug interactions within the network structure. Biomaterials 24:1499
- Ueno H, Mori T, Fujinaga T. (2001). Topical formulation and wound healing applications of chitosan. Adv Drug Deliv Rev 52:105–15
- Vernon B, Kim SW, Bae YH. (2000). Thermoreversible copolymer gels for extracellular matrix. J Biomed Mater Res 51:69–79
- Vodithala S, Khatry S, Shastri N, Sadanandam M. (2010). Formulation and evaluation of ion activated ocular gels of ketorolac tromethamine. Int J Curr Pharm Res 2:33–8
- Wang K, Li WF, Xing JF, et al. (2012). Preliminary assessment of the safety evaluation of novel pH-sensitive hydrogel. Eur J Pharm Biopharm 82:332–9
- Wang Q, Mynar JL, Yoshida M, et al. (2010). High-water-content mouldable hydrogels by mixing clay and a dendritic molecular binder. Nature 21:339–43
- Weng L, Chen X, Chen W. (2007). Rheological characterization of in situ crosslinkable hydrogels formulated from oxidized dextran and N-carboxyethyl chitosan. Biomacromolecules 8:1109–15
- Witchterle O, Lim D. (1960). Hydrophilic gels for biological use. Nature 185:117
- Yan C, Altunbas A, Yucel T, et al. (2010). Injectable solid hydrogel: mechanism of shear-thinning and immediate recovery of injectable hairpin peptide hydrogels. Soft Matter 6:5143–56
- Yang D, Zhang JZ, Fu S, et al. (2009). Evolution process of polymethacrylate hydrogels investigated by rheological and dynamic light scattering techniques. Colloids Surf A 355:197–203
- Yang P, Li D, Jin S, et al. (2014). Stimuli-responsive biodegradable poly(methacrylic acid) based nanocapsules for ultrasound traced and triggered drug delivery system. Biomaterials 35:2079–88
- Yin R, Tong Z, Yang D, Nie J. (2011). Glucose-responsive microhydrogels based on methacrylate modified dextran/concanavalin A for insulin delivery. J Control Release 152:e163–5
- Yin X, Stayton PS, Hoffman AS. (2006). Temperature- and pH-responsiveness of poly(Nisopropylacrylamide-co-propylacrylic acid) copolymers prepared by RAFT polymerization. Biomacromolecules 7:1381–5
- Yong-Hee K, Bae YH, Kim SW. (1994). pH/temperature-sensitive polymers for macromolecular drug loading and release. J Control Release 28:143–52
- Yoshii F, Kume T. (2003). Process for producing gross linked starch derivatives and cross linked starch derivatives produced by the same. US Patent US 6617448 B2
- Zha L, Banik B, Alexis F. (2011). Stimulus responsive nanogels for drug delivery. Soft Matter 7:5908–16
- Zhang K, Wu XY. (2002). Modulated insulin permeation across a glucose-sensitive polymeric composite membrane. J Control Release 80:169–78
- Zhang L, Chen J, Han C. (2009). A multicenter clinical trial of recombinant human GM-CSF hydrogel for the treatment of deep second-degree burns. Wound Repair Regen 17:685–9
- Zhang L, Li K, Xiao W, et al. (2011). Preparation of collagen–chondroitin sulfate–hyaluronic acid hybrid hydrogel scaffolds and cell compatibility in vitro. Carbohydr Polym 84:118–25
- Zhang R, Ma PX. (1999). Poly(hydroxyl acids)/hydroxyapatite porous composites for bone-tissue engineering preparation and morphology. J Biomed Mater Res 44:446
- Zhang Y, Tang Y, Wang Y, Zhang L. (2011). Nanomaterials for cardiac tissue engineering application. Nano-Micro Lett 3:270–7
- Zworykin VA, Hillier J, Snyder RL. (1942). A scanning electron microscope. ASTM Bull 117:15–23