Abstract
The potent anti-proliferative and pro-apoptotic actions of tocotrienols (T3) against cancer, but not normal tissues, have been hampered by their limited systemic bioavailabilty. Recent expansive development of diverse nanoemulsion (NE) vehicles emphasized their vast potential to improve the effective dosing of different clinical and experimental drugs of lipophilic nature, such as T3. The emphasis of the present work is to develop a pharmaceutically scalable, low-energy nano-emulsification approach for optimized incorporation of T3-rich palm oil (Tocomin®), possessing anticancer activity as a potential cutaneous delivery platform for adjunctive therapy of skin carcinomas, either alone or in combination with other chemotherapeutic agents. Different Tocomin®-NEs, obtained with different homogenization strategies, were screened based on physicochemical uniformity (droplet size, charge and polydispersity) and subjected to stress physical stability testing, along with chemical content analysis (≥90% Tocomin® - incorporation efficiency). Adopted hybrid nano-emulsification of Tocomin®, correlated with highest preservation of DPPH-radical scavenging capacity of active T3 in prototype formulation, Tocomin®-NE, which effectively permeated diffusion cell membranes 4-folds higher than propyleneglycol (PG)-admixed Tocomin® control. Against two different cell models of human cutaneous carcinoma, Tocomin®-hybrid NE demonstrated significantly stronger cytotoxic profiles (p ≤ 0.01), visible in both concentration- and time- dependent manners, with at least 5-folds lower IC50 values, compared to those estimated for the closest Tocomin®-control. The proposed hybrid nano-emulsified formulation of Tocomin® provides simple and stable delivery platform, for effective topical application against keratinocyte tumors.
Introduction
Basal cell carcinoma (BCC) and cutaneous squamous cell carcinomas (SCC) – originate in keratinocytes, and collectively referred to as non-melanoma skin cancers (NMSC) – are by far the most common forms of cancer in humans (Rass & Tilgen, Citation2008). Specifically, epidermoid carcinoma, the main malignant SCC tumor type, occurs predominantly in the squamous cells of skin, mouth, esophagus, prostate, lungs and cervix (Chen et al., Citation2008), and henceforth considered the second most prominent form of skin cancer, after basal cell carcinoma. Owing to the high overall metastasis potential of NMSC, patients with regional and visceral metastases often receive palliative combinations of aggressive chemotherapy (Chen et al., Citation2008; Rass & Tilgen, Citation2008). Nonetheless, these cytotoxic combos are associated with marginal survival prolongation and a multitude of toxicities to the patient (Kakumanu et al., Citation2011). Therefore, novel and less invasive topical therapeutic approaches are attracting the most attention, especially in the management and control of advanced skin carcinomas (Rass & Tilgen, Citation2008).
Vitamin E (VE), applies to a family of fat-soluble vitamins known as tocopherols (Tph) and tocotrienols (T3), whereas the natural vitamin E extract of palm oil is commonly referred to as tocotrienol-rich fraction (Sen et al., Citation2006; Ali et al., Citation2010), which can be commercially available under the name “Tocomin®”. Tocomin® is an oily vitamin E mixture composed of different tocotrienols (at least 50% wt), and tocopherols (not more than 15–20% wt). Tocotrienols and tocopherols are quite similar, chemically characterized by a chromane multi-ring core structures, attached to a side phytyl side chain () (Ali et al., Citation2010; Sylvester et al., Citation2010). Vitamin E analogues are commonly recognized for their potent antioxidant activity (Sen et al., Citation2006; Aggarwal & Nesaretnam, Citation2012). Similar to known α- and β-tocopherols, δ- and γ-tocotrienols are capable of scavenging harmful reactive oxygen species. Specifically, their anti-oxidation potential resides mainly with their “chain-breaking” property, which quenches peroxyl and alkoxyl radicals generated during lipid peroxidation (Sen et al., Citation2006; Aggarwal & Nesaretnam, Citation2012).
Figure 1. Chemical structures of various vitamin E compounds and isoforms. Illustrating the primary similarities and differences between tocopherols (Tph) and tocotrienols (T3).
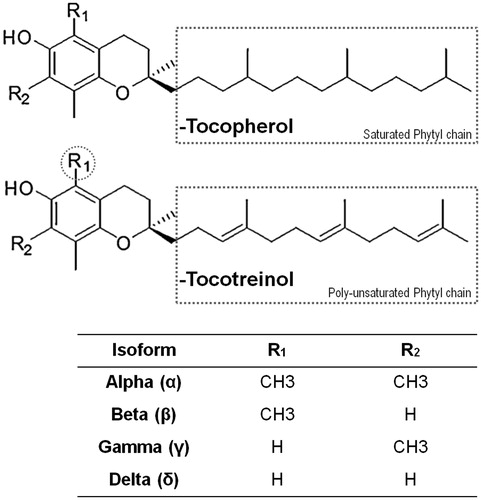
Linked to their anti-oxidative properties, T3s have been broadly studied for their important biological and pharmacological actions, such as neuroprotective, anti-atheroslerotic, anti-cholesterolemic and anti-osteoporotic activities (Sylvester et al., Citation2010; Aggarwal & Nesaretnam, Citation2012). In addition, the anti-proliferative effects elicited by tocotrienol analogues, within Tocomin®, gained them growing attention in cancer therapy (Sylvester et al., Citation2010; Alayoubi et al., Citation2013b). Palm oil fraction rich in tocotrienols have exhibited marked anticancer activity against various types of tumor tissues, of murine and human origins, such as mammary, prostate, hepatic and gastric cancers (Rajendran et al., Citation2011; Patacsil et al., Citation2012; Alayoubi et al., Citation2014). Moreover, certain tocotrienols (γ-tocotrienol, γ-T3) have been investigated in combination treatments to sensitize cancer cells towards co-administered therapeutic drugs, consequently enhancing the overall anticancer efficacy (Kannappan et al., Citation2010; Rajendran et al., Citation2011).
Due to the lipophilic nature of T3s, these natural VE analogues suffered from very poor water miscibility, thus exhibited very limited absorption following systemic administrations, either intraperitoneally or intramuscularly (Sen et al., Citation2006; Abuasal et al., Citation2012). Moreover, following a single bolus dose of 10 mg/kg in rates, γ-T3 failed to achieve more than 10% of oral bioavailability (Abuasal et al., Citation2012). While intravenous delivery was considered a possible alternative route for T3 administration, however, their extensive reticulo-endothelial (RES) clearance, leading to short plasma half life, approx. 2.5–3 h, is currently considered as the main limitation (Abuasal et al., Citation2012). At the same time, while extensively investigated for Tph antioxidant skin therapy (Cichewicz et al., Citation2013), topical and transdermal means of administration have not been sufficiently explored for skin-localized or even deeper-tissue delivery of T3-enriched-oil components (Muller et al., Citation2010). Such dermal applications of T3s would be of great clinical importance for the management of pre/cancerous skin lesions and cutaneous tumors.
Owing to their unique nano-size, and thus high interfacial area, nanoemulsions (NE) exhibit low viscosity and long-term colloidal stability, against sedimentation and creaming (Sarker, Citation2005; McClements et al., Citation2007; Date et al., Citation2010). NE vehicles offer various pharmaceutical advantages for hydrophobic as well as hydrophilic therapeutic cargos, depending on their type, thereby allowing delivery into polar versus non-polar matrices, potentially reducing toxicity. The high surface/volume ratio of the NE is responsible for increased drug incorporation within nano-structured particles, shown to allow more extensive tissue permeability, thus improve overall bioavailability and efficacy of loaded pharmaceuticals (McClements et al., Citation2007; Date et al., Citation2010). Consequently, with the relative ease in fabrication and scale-up, NEs are increasingly becoming a major pharmaceutical platform for improved dermal, transdermal and mucosa transport of various drugs (Sarker, Citation2005; Muller et al. Citation2007; Date et al., Citation2010).
Nanoemulsification of vitamin E compounds has been reported with great success in different reports (Kuo et al., Citation2007; Jordan et al., Citation2012; Saberi et al., Citation2013b) via the utilization of self-nanoemulsifying drug delivery systems, SNEDDS, as the primary surfactant blend (Anton et al., Citation2007; Date et al., Citation2010). Stable NE enriched with vitamin E (such as α- and γ-Tph, and T3) have been developed for parenteral and oral administration, as antioxidant therapy, as well as a nano-delivery vehicle to enhance the systemic bioavailability of many slightly water-soluble anticancer agents, such as paclitaxel, docetaxel, camptothecin, topotecan, etoposide curcumin, quercetine, genistein, etc (McClements et al., Citation2007; Bose et al., Citation2013; Pham et al., Citation2013). For topical administration (Muller et al., Citation2007), NE formulations have been successfully developed for improved cutaneous tissue permeation and residence time of Tph compounds, serving mostly as skin antioxidants (Kuo et al., Citation2007), to ameliorate UV-induced oxidative cell damage (Cichewicz et al., Citation2013). The majority of NE formulations of vitamin E analogues depend on the high-energy emulsification methods, such as high-pressure homogenization and ultrasonic emulsification to create nano-droplets with high kinetic energy (Ng et al., Citation2013; Alayoubi et al., Citation2014). During these processes, several forces, such as hydraulic shear, intense turbulence and collision, sometimes along with cavitation, act together to yield NE with extremely small particle size. Even though high-energy emulsification methods yield nanoemulsions with desired physical properties, and facilitate industrial scalability, such use of high mechanical energy is not generally recommended for temperature-sensitive and labile active ingredients, such as antioxidants, retinoids and macromolecules including proteins, enzymes and nucleic acids (McClements et al., Citation2007; Saberi et al., Citation2013b).
Our current work compares alternative low-energy emulsification methods to develop T3-enriched (aka Tocomin®)-NE, which are physically stable against coalescence in biological media. Using a panel of in vitro physical, biochemical and biological assays, prototype NE formulations are investigated to improve the efficiency of transdermal delivery of T3s, thus enhance the anti-proliferative activity of Tocomin®-NE against two NMSC cell models.
Materials and methods
Materials and cell lines
Commercial GMPO-grade tocotrienol (T3)-rich fraction of red palm oil “Tocomin® 50%” was generously provided by the manufacturer (Carotech Inc., Perak, Malaysia), and is referred to as Tocomin®, for the remainder of the report. Tocomin® is non-GMO-certified to contain ≥50% wt tocotrienols (15.4% dl-α-Tocotrienol, 28.2% dl-γ-Tocotrienol, 6.4% dl-δ-Tocotrienol) and approx. 23% wt dl-α-Tocopherol, plus 25% phytocarotenoids, phytosterols and squalenes. While glycerol, DPPH reagent (2,2-diphenyl-1-picrylhydrazyl), Trypsin/EDTA, penicillin/streptomycin, and fetal bovine serum (FBS) were obtained from Fisher Scientific (Waltham, MA). Tween® 21, Cell titer blue® reagent, and BD-Black 96-well tissue culture plates were purchased from VWR International (Radnor, PA). Seziol® TPGS-Pharma (NF-grade Vitamin E polyethyleneglycol succinate, TPGS) and Solutol® HS-15 (SHS-15) were generously supplied by Cognis and Muchler Inc./BASF (Cincinnati, OH), respectively. Human tongue squamous cell carcinoma (SCC-4) cells, along with human epidermoid carcinoma (A431) were purchased from American Type Culture Collections (Manassas, VA). Milli-Q (MQ) water was utilized for all preparations.
Methods
Preparation of tocotrienol-rich nanoemulsion system (T3-NE)
High-energy sonication method
Tocomin®-NE were prepared by coarse homogenization followed by 60 min ultra-sonication (under cooling & N2 gas). Briefly, to the T3-rich oily phase (composed of Tocomin® 50%), the surfactant blend (solutol HS15:VE-TPGS as 6:4 wt) was added, while warming and gently mixing on a vortex mixer at 800 rpm at 50 °C, for 2 min. The produced homogenous oil–surfactant mixture was hydrated with MQ water – containing 2.25% wt glycerol, with adjusted pH ∼7.8 ≥ 0.03, for tonicity – followed by brief vortex mixing, then homogenization for 5 min at 20 000 rpm using Ultra Turrax-10 homogenizer (IKA Works Inc., Wilmington, NC) to produce the coarse oil-in-water (O/W) emulsion (Saberi et al., Citation2013a). Subsequently, micro-emulsions were ultra-sonicated at 7-watts power, using a Misonix XL2000 probe sonicator (P-1 medium amplitude microprobe, Qsonica, Newtown, CT) for four cycles (each sonication cycle time is divided as 15 min: on + 5 min: off), to obtain the nanosized oil droplets, Tocomin®-Sonic NE (Jordan et al., Citation2012; Pham et al., Citation2013).
Low-energy phase inversion temperature-emulsification method
The phase inversion temperature (PIT) homogenization method relies on inducing a phase transition, during the emulsification process, to emulsions stabilized with non-ionic agents (Izquierdo et al., Citation2004). Simply, O/W microemulsions are transiently transform to W/O types at relatively higher temperatures, then their droplets revert back to an O/W nano-scaled emulsion type, upon rapid cooling (Izquierdo et al., Citation2004; Tadros et al., Citation2004). Specifically, Tocomin®-based microemulsions (composed of O:S:W in 1.5:0.5:8.0 wt ratio), were similarly fabricated using the same surfactant blend (solutol HS15:VE-TPGS as 6:4 wt), adopted for all the different homogenization methods (Anton et al., Citation2007). Then, samples were brought, rapidly and with agitation, from the corresponding hydrophilic-lipophilic balance (HLB), temperature (approx. 72 ± 3 °C) to 25 °C, using water/ice bath, to achieve fast cooling. Such rapid temperature cycling was repeated up to three times, then, resulting NE systems were kept at 25 °C, Tocomin®-PIT NE (Anton et al., Citation2007; Saberi et al., Citation2013a).
Hybrid emulsification method
Briefly, the coarse O/W emulsions of Tocomin®, prepared exactly as described prior, were brought, rapidly and with agitation, from the corresponding HLB temperature (approx. 72 ± 3 °C) to 25 °C, using water/ice bath (Izquierdo et al., Citation2004). Following this single PIT cycle, the Tocomin®-NEs were ultra-sonicated at 7-watts power, using a Misonix XL2000 probe sonicator (P-6 low amplitude microprobe, Qsonica, Newtown, CT) for only two cycles of sonication (each sonication cycle time is divided as 15 min: on + 5 min: off, equivalent to 30 min of total sonication time), to obtain homogenous nanosized oil droplets, Tocomin®-hybrid NE (Jordan et al., Citation2012). A control formulation, α-Tph-NE (utilizing same adopted NE composition of O:S:W in 1.5:0.5:8.0 wt ratio), was also prepared via the same hybrid emulsification technique.
Physicochemical characterization of NEs
Droplet size analysis
The NEs were diluted with de-ionized distilled water before analysis, and the numbered average oil droplet hydrodynamic diameter and the polydispersity index (PDI) were determined using dynamic light scattering technique (Phan et al., Citation2013), via Malvern Zetasizer Nano-ZS (Malvern Instruments Inc., Westborough, MA).
Zeta potential (ζ) measurements
All NEs samples were diluted with de-ionized distilled water and placed in the electrophoretic cell of the Malvern Zetasizer Nano-ZS (Nano ZS, Malvern Instruments Inc., Westborough, MA) and the average surface charge was determined (Phan et al., Citation2013).
Tocomin®-incorporation efficiency determination via HPLC (in terms of γ-T3 recovery)
Being the primary active and the most abundant form of T3, within the Tocomin® phase, γ-T3 content in NE formulations was analyzed – in representation for whole residual T3 of the Tocomin® oil fraction – using a modified reversed-phase-high-performance liquid chromatography (HPLC) method (Ali et al., Citation2010; Alayoubi et al., Citation2013a). Briefly, 50 μL of Tocomin®-NE were dissolved in 5 mL of mobile phase, then injected (25 μL) through ACE PFP C-18 column (4.6 × 250 mm, 4 µm packing vol) using 50% v/v methanol and 50% v/v ethanol/acetonitrile (1:1) blend, as the mobile phase. The flow rate of the mobile phase was maintained at 0.5 mL/min and UV detection was performed at λmax = 295 nm. The γ-T3 concentration in the sample was determined using a calibration curve of high-purity (≥97%) γ-T3 HPLC standard, dissolved in methanol. The incorporation efficiency (IE%) of Tocomin® in the NE samples was calculated based on Tocomin® certificate of analysis, supplied by the manufacturer (Jordan et al., Citation2012; Ng et al., Citation2013), measured initially (D0) and after 60 days (D60) of refrigerated storage conditions (4–8 °C).
Storage stability and stress testing
Physical stability of Tocomin®-NEs
Both particle size and surface charge of all Tocomin®-NEs were monitored over 2 months of storage in refrigerated conditions (4–8 °C) (Ng et al., Citation2013; Phan et al., Citation2013). The stability of the nanoemulsions in culture media (RPMI-1640 culture medium, containing 10% FBS) at 37 °C was also carried out by monitoring the change in particle size over 48 h of incubation to simulate the conditions during the in vitro anti-proliferation study (data not shown) (Jordan et al., Citation2012; Alayoubi et al., Citation2013b).
Mechanical stress testing
Physico-chemically characterized samples (referred to as T0 h) were placed randomly on a microprocessor-controlled thermal-mixer (Fisher Scientific, Waltham, MA), and agitated at 250 rpm, for 24 h at RT, 23 °C. At the end of each test, samples were analyzed for particle size and PDI (referred to as T24 h), plus visually inspected for signs of phase separation (Alayoubi et al., Citation2013b).
Thermal stress testing
The various Tocomin®-NEs were subjected, in quadruplicates, to three freeze–thaw cycles of 24 h each (freezing at a temperature of −20 °C for 20 h, followed by thawing at a temperature of 32 °C), then were examined visually for phase separation and the NE particle size was also determined (Ng et al., Citation2013).
Antioxidant activity by the DPPH assay
The in situ biochemical antioxidant activity of Tocomin® after incorporation within NE was measured via its antioxidant activity in the DPPH• assay (DPPH• is a stable nitrogen-centered free radical) (Thaipong et al., Citation2006; Mostafa et al., Citation2014). Briefly, for radical scavenging measurements, 50 μM of DPPH were dissolved into 5 mL of buffered methanol (prepared from 0.1 M acetate buffer, pH 5.5 and methanol in 4:6 vol/vol ratio). After mixing with 45 μL of test sample (different formulations, diluted buffered methanol), the light absorbance was measured after 30 min at 517 nm, using a Lambda-EZ 210 spectrophotometer (Perkin Elmer, Akorn, OH) (Mostafa et al., Citation2014). Positive control was prepared using control MQ external NE phase with 2.25% glycerol (unprocessed), indicating maximum odd DPPH• electrons (100% free radicals), and all measurements were performed in quadruplicate (Thaipong et al., Citation2006). Comparative hybrid-nanoemulsified control, α-Tph-NE, served as antioxidant tocopherol (Tph) control for test Tocomin® NEs. Based upon our previous work (Jordan et al., Citation2012), coconut oil-based NE, prepared also via hybrid emulsification, was also included (Coco-NE), to serve as a negative (−ve) control for VE-rich NEs (coconut oil phase is comprised mainly of saturated fatty acid triglycerides). Finally, a negative processing control, made of 15 wt% of Tocomin®-in propyleneglycol admixture (Tocomin®-PG) was adopted for further studies (Cichewicz et al., Citation2013).
Simulated skin permeability studies
The permeation and penetration of γ-T3 (as primary active component of Tocomin®) from different NE formulations will be evaluated using multi-Franz diffusion cell apparatus (surface area = 0.64 cm2; receiver liquid volume 5 mL) in a 37 °C-regulated water jacketed system). To simulate for typical IVIVC conditions (Karadzovska & Riviere, Citation2013; Mostafa et al., Citation2014), the receiver compartment will contain PBS (50 mM, pH 7.4) mixed with 0.5% Tween 21, to achieve sink condition), and kept under constant stirring. In order to simulate for the stratum corneum component of skin, artificial cellulose-ester (CE) membranes (0.45 μm), hydrophobically-saturated/coated with isopropyl myristate (IPM), were utilized, after hydration for 24 h with PBS. The donor compartment contained 0.1 mL of test formulations. Using a 500 μL long-needle HPLC syringe (Hamilton Company, Reno, NV), exactly 0.2 mL of each sample is collected, at every hour between 1 and 8 h of incubations, then finally at 12 h time-point. After each collection, the same volume of PBS in the receiver compartment will be compensated. The tests were performed with five cells per each preparation (Karadzovska & Riviere, Citation2013; Mostafa et al., Citation2014).
In vitro cell culture
Human tongue squamous cell carcinoma, SCC-4, and human epidermoid carcinoma, A431, cells, were grown in RPMI-1640, and DMEM culture media, respectively, containing 10% FBS, and penicillin (100 U/mL)/streptomycin (100 μg/mL) in a humidified environment of 37 °C, 5% CO2. Cells were seeded at a concentration of 6 × 103–1.5 × 104 cells/cm2 and sub-cultured at approximately 70–80% confluency. Cells between passages 8–15 were used for experimentation (Kakumanu et al., Citation2011; Jordan et al., Citation2012; Phan et al., Citation2013).
Anti-proliferative assays in skin cancer cell models
Human skin carcinoma cell lines, SCC-4 and A431, were seeded at 10 × 103 cells per well of 96-well plates in six replicates for 24 h. Then, the respective complete culture media were exchanged for serum-free media containing several two-fold serial dilutions of the T3-based negative processing control, made of 15 wt% of Tocomin® in propyleneglycol admixture (Tocomin®-PG) (Cichewicz et al., Citation2013), compared with selected prototype Tocomin®-Hybrid NE formulation at certain co-incubation time points (24, 36, 48, 72 and 96 h), the total cell viability was determined using CellTiter Blue® Kit (Promega, Madison, WI), after washing twice with HBSS and reading sample plate fluorescence (λEx = 480 nm/λEm = 530 nm), using Synergy 2 Biotek fluorescence plate reader (Biotek instruments Co., Winooski, VT), according to manufacturer’s instructions (Jordan et al., Citation2012; Pham et al., Citation2013).
Data analysis
A minimum of triplicates were run for each experiment. Data were reported as mean ± standard deviation. Regression analysis and curve fitting graph (r2 = 0.908–0.992) was done using MagicPlot Pro software, ver. 2.0 (St. Petersburg, Russia). Comparisons between the groups were made using Student’s t-test, and with more than two groups, non-parametric NOVA (Kruskal-Wallis test, with Tukey’s post-hoc analysis) was used to compare results. The p < 0.05 values are considered statistically significant. All statistical analyses were performed using Graphpad Prism Software, ver. 5.0 (La Jolla, CA) (Pham et al., Citation2013).
Results and discussion
Systematic screening of three different methods for fabrication of Tocomin® oil NE, utilizing varied high- and low-energy processes of emulsification – for equal formulation compositions – is described. Based on previous reports of superior emulsification results of VE-enriched NEs, the basic composition ratio of test O/W formulations was kept constant (oil/surfactant blend/water as 25/8/67 wt %) (Jordan et al., Citation2012; Pham et al., Citation2013). Of which, the surfactant blend consisted of 60 wt% polyethoxylated Solutol-HS 15, as the primary non-ionic surfactant (Anton et al., Citation2007), mixed with 40 wt% VE-TPGS, which serves as co-surfactant and stabilizing agent (quite similar in role to poloxamer-188, highlighted in earlier reports of T3-NE) (Alayoubi et al., Citation2013a, Citation2014). This system was used as the starting point for the evaluation of the primary physico-chemical characteristics of NE approaches for Tocomin®. Finally, the intrinsic biochemical and biological activities of prototype Tocomin® -NE were tested for antiproliferative effectiveness, to assess its potential as active topical vehicle to support anticancer treatment, as discussed in the following sections.
Preparation and characterization of different Tocomin®-NE
Structurally, as a mixed heterogeneous phase systems, NEs have the ability to solubilize hydrophobic, as well as hydrophilic drugs in their nanostructure, depending on the O/W or W/O type of NE, respectively (McClements et al., Citation2007; Date et al., Citation2010). In practice, the incorporation of water-insoluble therapeutic agents in NEs, specifically the active Tocomin® fraction of palm oil, can offer improvements in the chemical and/or enzymatic stability of these labile therapeutic agents, leading to improvement in pharmaceutical shelf life, dose administration, local tissue or systemic bioavailability, and/or therapeutic efficacy, especially for dermal applications (McClements et al., Citation2007).
In order to successfully incorporate the viscous oily phase of Tocomin®, NE were first prepared from their coarse emulsion precursors using high energy input via probe ultrasonication (for 30 or 60 min active periods) (Jordan et al., Citation2012) in contrast to the low-energy emulsification using PIT method, with either 2- or 3-repeated temperature cycling (Izquierdo et al., Citation2004), and finally a hybrid method, where 30 min of ultrasonication is followed by only one PIT cycle. Nano-emulsification method data, presented in , demonstrated smaller nanosized droplets (approx. 220–240 nm) for when using 60 min of sonication and 3-cycles of PIT, compared to lower sonication times and PIT cycles (). Similarly, the hybrid emulsification method produced smaller nanodroplets (211.4 ± 8.6 nm, ), which were characterized with a relatively low PDI value (0.29 ± 0.03, ), and the highest ζ-potential (−29.7 ± 1.8 mV), in comparison with the other two methods. In fact, , panels B–C demonstrated the highest PDI and lowest negative ζ-potential values for Tocomin® oil nanoparticles emulsified via PIT method (for both 2- and 3-cycles), indicating significantly less homogenous dispersion of produced NEs (p ≤ 0.05).
Figure 2. Physico-chemical characterization of different homogenization methods for Tocomin® NE formulation. Change in (A) mean droplet size; (B) Polydispersity Index, PDI; (C) and interfacial electrical charge (measured as ζ-potential), of the various Tocomin® NE homogenization processes. (n = 4–5, mean ± SD values denoted with unlike symbols are statistically different, p ≤ 0.05).
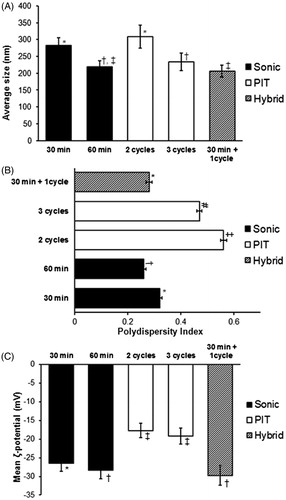
As expected (Das et al., Citation2011; Bose et al., Citation2013), longer ultra-sonication resulted in more efficient breakage of coarse emulsion droplets into the nanometer range and subsequent particle size reduction (, panels A–B). Although doubling the ultrasonic energy time (while maintaining relatively low energy input) significantly improved the nanoscale properties of Tocomin®-NE (Sonic 60 versus Sonic-30, ), the concern about adversely reducing the active T3 content of the oil remains. In fact, such notion is further substantiated by the significantly low IE% of T3 in Sonic-60-NE (90–91%), in comparison with a minimum value of 95–96% obtained in the other two Tocomin®-NEs, all HPLC analyzed at the same time points ().
Table 1. Chemical and physical stability of different Tocomin®-NE preparations.
Based on the presented physical stability data (), a sonication time of 60 min, and 3-cycles of PIT were selected for the high- and low-energy emulsification methods, respectively, and will be adopted for further comparison between produced Tocomin®-NEs, based on extended shelf life and stress stability tests, as well as biochemical analysis.
High-energy emulsification methods (typically 20–30 cycles of microfluidizer/high-shear homogenization, or 30–60 min of ultrasonication) are often utilized (Kuo et al., Citation2007; Bose et al., Citation2013; Alayoubi et al., Citation2014) due to the relatively high viscosity of the T3-rich palm oil fraction. Even though such harsh methods generally yield VE-NEs with desired properties and have industrial scalability, they may not be suitable for thermo-sensitive biological compounds, such as vitamins (e.g. A, C, D and E), poly-unsaturated fatty acids and proteins/peptides, also suffering from susceptibility to rapid oxidation (McClements et al., Citation2007; Muller et al., Citation2007). Furthermore, such high-energy methods require sophisticated instruments and extensive energy input, which considerably increases the cost of NE fabrication. Hence, research efforts have also focused on facile low-energy nano-emulsification techniques (Sarker, Citation2005; Date et al., Citation2010).
The PIT method was first described as an alternative to high shear emulsification methods (Izquierdo et al., Citation2004; Tadros et al., Citation2004). The technique is based on phase inversion process provided by the ability of polyethoxylated non-ionic surfactants to modify their affinity to water or oil according to changes in temperature. The PIT-based methods attracted a lot of attention because of its simplicity, and low-energy consumption, allowing easy scale-up, as well as preventing drug degradation during the process, plus avoiding any organic solvent residues, which can pose a potential human health risk (Sarker, Citation2005; Date et al., Citation2010). With expanded utilization of PIT, data suggested lower physical stability (e.g. agglomeration) of PIT-produced NEs – particularly when incorporating viscous oil/VE components – possibly owing to shedding of surfactant molecules from nanodroplet’s surface, which is manifested in , through reduction in their ζ-potential measured after short-term cold storage (initial ζ-potential = −21.3 ± 1.8 mV versus recorded value of −17.1 ± 1.2 mV, after 30 days) (McClements et al., Citation2007; Saberi et al., Citation2013b).
Consequently, our proposed hybrid nano-emulsification technique of single PIT cycle, followed by reduced (20–30 min) ultrasonication post-processing, may prove to be a much milder, yet as efficient homogenization approach, capable of producing stable Tocomin®-NEs.
Physical and biochemical stability of Tocomin®-NE
Regardless of the emulsification method employed, several factors, such as the viscosity of the oil phase, type of emulsifiers utilized, homogenizing energy input, and repeated homogenization cycles, were found to have a significant effect on both physical stability and size of the NE droplets (McClements et al., Citation2007; Alayoubi et al., Citation2013b; Saberi et al., Citation2013a,Citationb). In our long-term stability study, formulation characteristics (internal phase diameter, polydispersity and zeta potential), along with drug content (expressed as IE%) were assessed after storage of the formulation at 4 °C for up to 60 days (, panel A).
Figure 3. Physical stability of selected Tocomin® NE preparations. Change in mean droplet size, ζ-potential and polydispersity index during (A) cold storage for 60-days, 4–8 °C; (B) mechanical stress test (24 h shaking at 250 rpm, 23 °C); (C) temperature cycling stress test (up to three repeated cycles of freezing–thawing, 24 h each), performed on select Tocomin® NE homogenization processes (n = 4, mean ± SD values denoted with unlike symbols are statistically different, p ≤ 0.05).
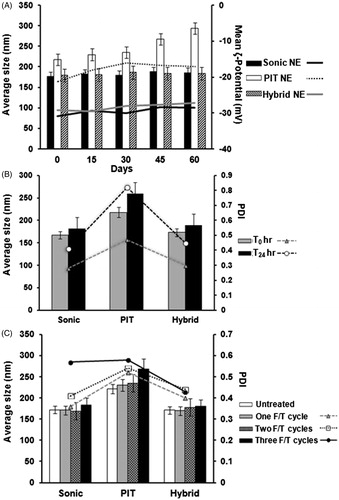
Overall, the physical properties of Tocomin®-NEs remained fairly unchanged after 8 weeks of cold storage. There was <10 nm change (Δ) in particle size (z-ave) and <4 mV change in absolute ζ-potentials – as observed in either Sonic or Hybrid-NE – indicating superior stabilization of the adopted NE composition to maintain adequate Tocomin® incorporation (90–95% at D60), without compromising their integrity or the formulation characteristics (). Nevertheless, Tocomin®-NE produced by 3-cycle PIT showed marked increase in mean particle diameter, associated with noticeably lower ζ-potential values (mean PIT-NE ζ-potential is −17.6 mV, compared to −29.3 mV and −28.5 mV for Sonic- and Hybrid-NE, respectively), starting within the first 15 days of cold storage. The significantly higher PDI values (, p < 0.05), corresponding to Tocomin®-NE produced by 3-cycle PI (mean PDI ∼0.65 versus 0.33 and 0.35 for Sonic- and Hybrid-NE, respectively) corroborate these results. As mentioned earlier, relative tendency for agglomeration can be implicated in such noted instability of PIT-NE of Tocomin®, over time. In general, higher zeta potential values (either positive or negative, 20–30 mV on average) tend to stabilize particle suspensions by inducing repulsion between similarly charged particles, and thereby reduce their potential to agglomerate (Muller et al., Citation2007; Bose et al., Citation2013).
Stress testing data of our chosen proof-of-concept Tocomin®-NEs (, panels B–C), further affirms the far superior consistency of both the Sonic- and Hybrid-NE formulations of Tocomin®, over the PIT-prepared one. Under accelerated stability conditions (RT with agitation, , panel B), hybrid nano-emulsified Tocomin® showed marginal change (Δ) in mean droplet size (<8% change), and its values were very close to those measured for Tocomin® produced via sonication for 60 min. After 24 h test period, PDI values for Sonic-NE and Hybrid-NE increased by almost 37%, to 0.41 and 0.44, respectively. Conversely, PIT-NE sample suffered from 22% increase in particle diameter (Δ ∼ 45 nm), and almost 100% rise in corresponding PDI values, in conjunction with the appearance of a creaming top layer, within an hour post-test, due to accelerated aggregation and agglomeration of PIT-produced Tocomin® nanodroplets. Likewise, Sonic-NE and Hybrid-NE retained their structural uniformity over repeated freeze–thaw cycles, evident through nominal change in their z-ave droplet diameter ( – panel C), while the change in the particle size was more noticeable in case of PIT-NE sample. Interestingly, while the PDI Hybrid-NE sample was quite stable (Ave. PDI = 0.4 to 0.43) over all three freeze–thaw cycles, Sonic-NE sample manifested a substantial shift in measured PDI (from 0.41 to 0.58, i.e. Δ > 40%), after the third/final freeze–thaw cycle.
Measuring the inhibition of DPPH free radical provides a direct estimate of the antioxidant capacity preserved in each NE sample (Muller et al., Citation2010; Mostafa et al., Citation2014), which allows for assessment of the effects of each nano-emulsification technique on the activity of incorporated Tocomin® (). Unprocessed Tocomin® mixed with propylene glycol (Tocomin®-PG) was employed here as a positive control, to represent 100% radical scavenging activity of Tocomin®. Hybrid nano-emulsified α-tocopherol (α-tocopherol-NE) was included to show comparative anti-oxidant capacity of processed α-tocopherol, to that of processed Tocomin®-NEs, since it would serve as a positive VE-NE control in subsequent cancer cell anti-proliferative assays (). Data obtained in current DPPH•-scavenging assay () revealed that α-tocopherol-NE sample had the most antioxidant activity, followed closely by the Hybrid-NE of Tocomin®. In contrast, both Tocomin®-NEs (Sonic and PIT) had noticeably lower antioxidant profile against DPPH•, which can be somewhat explained by the relatively lower γ-T3 retention () in these samples, mostly due to added processing steps/conditions.
Figure 4. DPPH-antioxidant assay of Tocomin® NE preparations. Homogenized NE formulations of Tocomin® were compared to tocopherol-containing hybrid NE, and Tocomin® mixed with propylene glycol, which serves as non-processes Tocomin® control (n = 4, mean ± SD values denoted with unlike symbols are statistically different, p ≤ 0.05).
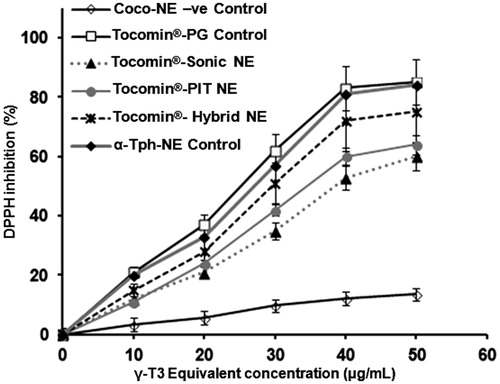
Figure 5. In vitro simulated-skin penetration assay. Franz diffusion cell model data showing (A) time-dependent membrane penetration profile of Tocomin®, over the initial period of 8 h; (B) accumulated amount of Tocomin® after 12 h, calculated based on HPLC-measured γ-T3, for different samples (n = 5, mean ± SD values denoted with unlike symbols are statistically different, p ≤ 0.05).
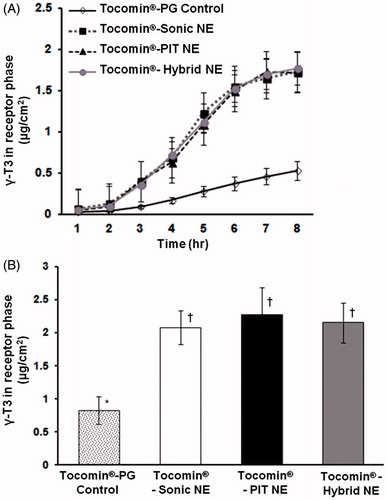
The Tocomin®-hybrid NE was adopted as prototype formulation, based upon its excellent physico-chemical properties: high physical stability (after cold storage, plus physical and thermal cycling stress testing) demonstrating smaller particle size (mean diameter ∼170 nm), PDI (Ave. ≤0.35), as well as maintaining the highest ζ-potential value (−29 ± 2.4 mV), compared to all other Tocomin®-NE preparations ( and ). And chiefly owing to its superior content recovery of γ-T3 (IE ≤ 95.7 ± 0.11%, post-60 days of cold storage, ), combined with demonstrating the highest activity of incorporated T3/Tocomin® against tested Tocomin®-NE controls (), all qualified Tocomin®-hybrid NE as the lead nano-emulsified Tocomin® formulation for further investigation.
In vitro permeation and anti-cancer activity of developed Tocomin®-NE
The permeation ability of the different Tocomin®-NE preparations was evaluated using in vitro simulated-skin permeation studies, carried out using a lipophilically (IPM)-coated artificial semi-permeable CE membranes, to generally mimic the hydrophobic-hydrophilic epidermal-subcutaneous skin layers (). The corresponding non-homogenized Tocomin®-PG formulation (simply admixed with propylene glycol) was also used as control (Cichewicz et al., Citation2013).
Data presented in indicate that all Tocomin® was released from all formulations, penetrating the artificial skin/membrane, into the receptor compartment of the Franz-cells. However, the permeation profiles varied widely between Tocomin®-NEs and the Tocomin®-PG control. For all tested Tocomin® samples, the permeation was estimated to reach a steady state, by T = 3 h, for comparison purposes, with apparent linear increase range until T = 6 h, which slowed markedly by T = 7–8 h. Hence, statistical comparison of the permeation rate in this linear region showed that all Tocomin®-NEs provided virtually the same conspicuous flux (J), significantly higher than the non-encapsulated Tocomin®-NEs Tocomin®-PG control (p ≤ 0.01) (Chen et al., Citation2006). In fact, the calculated J values for all homogenized/nanoscale Tocomin®-NEs (mean J = 0.392 to −0.397 μg/cm2 h) were at least 4-fold higher than those obtained with unprocessed Tocomin® mix with PG (J = 0.095 μg/cm2.h). Moreover, at the end of the study (post-12 h), the accumulative amounts of nano-formulated γ-T3 (in any of the Tocomin®-NEs) were approx. 2.5- to 3-fold higher, when compared with that released from control Tocomin®-PG ().
Whilst the NE and control PG-admixture had high concentrations of Tocomin®, in order to mediate for high permeation rate of Tocomin®, obviously its permeation rate from the PG-control preparation was greatly lower than those from NEs. This can be attributed to the surfactant effect of polyoxyethylene alkyl ethers (Solutol HS-15), which increased the solubility of Tocomin® in water, thus improved its permeation rate (polyethoxylated surfactants typically increase water affinity of NE), yielding water-continuous formulations, which has been shown to result in enhanced skin penetration of incorporated lipophilic compounds (Zhang & Michniak-Kohn, Citation2011; Cichewicz et al., Citation2013). Furthermore, based on the lag time, 2.11–2.12 h, almost equal between all tested Tocomin® preparations, in conjunction with markedly superior permeation profiles of Tocomin®-NEs, compared to corresponding Tocomin®-PG-control (), one can conclude that propylene glycol (PG), due to its limited effect to incorporate and solubilize the lipophilic Tocomin®, had clearly impacted the permeation of Tocomin® (PG, utilized here in the Tocomin® control preparation, is often used as permeation enhancer for sparingly-soluble compounds, for topical applications) (Chen et al., Citation2006; Cichewicz et al., Citation2013).
The noted potential enhancement in Tocomin® skin-penetration by NEs can be attributed to several factors, mainly: increased drug (i.e. T3, mainly γ-T3) solubilization capacity; very small droplet size associated with a high surface area (which would in turn improve interactions and drug transfer into the skin); ability to increase drug release directly at skin layer, compared to other formulations; and ability of their surfactant molecules to act as permeation enhancers, modulating the stratum corneum organization, and potentially decreasing its barrier function (Zhang & Michniak-Kohn, Citation2011; Cichewicz et al., Citation2013).
An expanding body of evidence supports that tocotrienols (γ, δ and α-T-3) posses potent neuroprotective, antioxidant, anticancer and hypo-cholesterolemic properties that are quite distinct from the properties of tocopherols (Tph) (Sen et al., Citation2006; Kannappan et al., Citation2010; Muller et al., Citation2010). Specifically, direct comparison between the two VE-subclasses indicated that Tocotrienols (T-3) were markedly more potent in suppressing growth and inducing cell death than tocopherols and that the relative effectiveness of specific vitamin E isoforms displayed a consistent relationship corresponding to δ-tocotrienol ≥ γ-tocotrienol > α-tocotrienol > δ-tocopherol. γ- and α-tocopherol (Kannappan et al., Citation2010; Muller et al., Citation2010; Sylvester et al., Citation2010). Moreover, when tested at similar dose levels, tocotrienols demonstrated significant specific antiproliferative activity against tumor cells, but not normal cells (Sylvester et al., Citation2010). Specifically, tocotrienols have been shown to exert potent growth inhibition of mammary carcinoma cells, inducing apoptosis in MDA-MB231 and MCF-7 breast cancer cells, mediated via PARP-cleavage and caspase-7 activation (Rajendran et al., Citation2011; Patacsil et al., Citation2012). On the other hand, the anticancer efficacy of per-oral administration of tocotreinol produced inconsistent results, primarily due to the insufficient/saturable tocotrienol absorption/transport mechanisms within the intestine and circulation, which subsequently limit their systemic bioavailability to target tissues (Sen et al., Citation2006; Sylvester et al., Citation2010). Still, based on encouraging experimental data revealing enhanced distribution of poly-unsaturated tocotrienols within the phospholipid layers of cell membranes – suggested as the main underlying cause for their superior antioxidant effects (Muller et al., Citation2010), in vitro – our hybrid NE formulation of Tocomin® for dermal and transdermal application, would mediate direct and intense inhibition against skin cancer cells.
Time-dependent profile of in vitro anti-proliferative activities against both human keratinocyte cancer cells, A431 () and SCC-4 () demonstrated marginal activity for both positive control treatments (α-Tph-NE and Tocomin®-PG), while Tocomin® hybrid NE showed relatively significant cell inhibition (p ≤ 0.05), after 48 h of incubation. Over longer incubation periods (72–96 h), cells treated with Tocomin®-PG were significantly more inhibited than α-Tph-NE or culture media treated ones. At the same time-points, and possibly owing to stable superior nanocarrier properties (Sarker, Citation2005; Kuo et al., Citation2007; Alayoubi et al., Citation2013b), the lowest cell viability was noted with Tocomin® hybrid NE treatment (p ≤ 0.01), reaching approx. 32.5% and 39% in A431, and SCC-4 cell cultures, respectively. Further analysis of the concentration curve-fitted profiles (, panels A–B), obtained in both A431 and SCC-4 cells, indicates not only divergently strong cancer cell inhibition, produced by Tocomin® hybrid NE treatment, starting at γ-T3 equivalent concentration as low as 12.5 μM (p ≤ 0.05–0.001), but confirms also the relatively limited anti-proliferative activity of α-tocopherol NE analogues, at such low doses (Alayoubi et al., Citation2013b). Indeed, the measured IC50 values after Tocomin®-NE treatment – 42.6 ± 3.8 μM and 47.3 ± 3.2 μM, in A431 and SCC-4 cells, respectively – were about 5-to-6-fold lower than those extrapolated for the Tocomin®-PG positive control (calculated as 217.4 μM in A431 cells and as 278.5 μM in SCC-4 cells). Such consistent inhibition of skin carcinoma cells, produced by our prototype Tocomin® hybrid NE over 96 h, substantiate the great potential therapeutic benefits for such topical nanosystem, as compared with liquid administration of tocotrienol-rich oils.
Figure 6. Concentration-dependent anti-proliferative activity of developed Tocomin® NE against skin carcinoma cells. Microplate-based CellTiter Blue® assay of human (A) epidermoid carcinoma, A431; and (B) squamous cell carcinoma, SCC-4, tissue cultures, at γT3-equivalent concentration range of 3–100 μM, and after incubation for 72 h, at 37 °C, 5% CO2 conditions. (n = 5–6, mean ± SD values denoted with unlike symbols are statistically different, p ≤ 0.05–0.01).
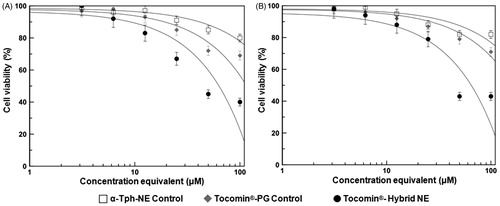
Figure 7. Temporal anti-proliferative activity of developed Tocomin® NE against skin carcinoma cells. Microplate-based CellTiter Blue® assay of human (A) epidermoid carcinoma, A431; and (B) squamous cell carcinoma, SCC-4, tissue cultures, at constant γT3-equivalent concentration dose of 30 μM, measured after different incubation times, 48–96 h, at 37 °C, 5% CO2 conditions (n = 5–6, mean ± SD values denoted with unlike symbols are statistically different, p ≤ 0.05–0.01).
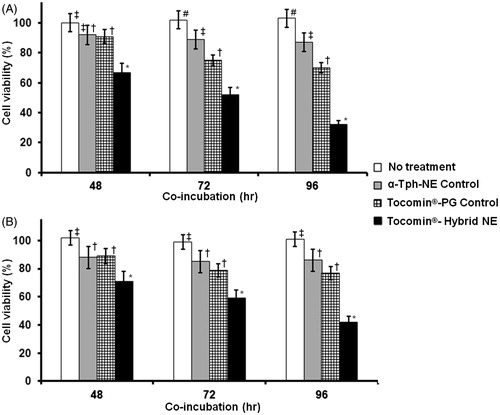
Conclusions
The beneficial aspects of the hybrid-nanoemulsified delivery system, reported in this article for Tocomin®, in comparison to comparable controls have been correlated to better yield in physicochemical parameters, as well as stability in chemical and structural composition. Superior nanoscale properties obtained with our prototype hybrid Tocomin®-NE, in conjunction with improved drug-membrane permeability, have consequently generated dramatic improvements in the anti-proliferative effectiveness of incorporated Tocomin® against tested skin carcinoma cell models. Our reported data suggest the potential of such hybrid nanoemulsion platform of Tocomin® for topical delivery, as a possible adjunctive therapeutic strategy for non-melanoma skin cancers.
Declaration of interest
All authors declare no conflict of interests present in this work.
This work was supported by intramural faculty development award by MWU-CPG grant committee.
References
- Abuasal BS, Lucas C, Peyton B, et al. (2012). Enhancement of intestinal permeability utilizing solid lipid nanoparticles increases gamma-tocotrienol oral bioavailability. Lipids 47:461–9
- Aggarwal B, Nesaretnam K. (2012). Vitamin E tocotrienols: life beyond tocopherols. Genes Nutr 7:1
- Alayoubi A, Alqahtani S, Kaddoumi A, Nazzal S. (2013a). Effect of PEG surface conformation on anticancer activity and blood circulation of nanoemulsions loaded with tocotrienol-rich fraction of palm oil. AAPS J 15:1168–79
- Alayoubi A, Ayoub NM, Malaviya A, et al. (2014). Entrapment into nanoemulsions potentiates the anticancer activity of tocotrienols against the highly malignant (+SA) mouse mammary epithelial cells. J Nanosci Nanotechnol 14:4002–5
- Alayoubi A, Kanthala S, Satyanarayanajois SD, et al. (2013b). Stability and in vitro antiproliferative activity of bioactive “Vitamin E” fortified parenteral lipid emulsions. Colloids Surf B Biointerfaces 103:23–30
- Ali H, El-Sayed K, Sylvester PW, Nazzal S. (2010). Molecular interaction and localization of tocotrienol-rich fraction (TRF) within the matrices of lipid nanoparticles: evidence studies by Differential Scanning Calorimetry (DSC) and Proton Nuclear Magnetic Resonance spectroscopy ((1)H NMR). Colloids Surf B Biointerfaces 77:286–97
- Anton N, Gayet P, Benoit JP, Saulnier P. (2007). Nano-emulsions and nanocapsules by the PIT method: an investigation on the role of the temperature cycling on the emulsion phase inversion. Int J Pharm 344:44–52
- Bose S, Du Y, Takhistov P, Michniak-Kohn B. (2013). Formulation optimization and topical delivery of quercetin from solid lipid based nanosystems. Int J Pharm 441:56–66
- Chen H, Chang X, Du D, et al. (2006). Microemulsion-based hydrogel formulation of ibuprofen for topical delivery. Int J Pharm 315:52–8
- Chen J, Ruczinski I, Jorgensen TJ, et al. (2008). Nonmelanoma skin cancer and risk for subsequent malignancy. J Natl Cancer Inst 100:1215–22
- Cichewicz A, Pacleb C, Connors A, et al. (2013). Cutaneous delivery of alpha-tocopherol and lipoic acid using microemulsions: influence of composition and charge. J Pharm Pharmacol 65:817–26
- Das S, Ng WK, Kanaujia P, et al. (2011). Formulation design, preparation and physicochemical characterizations of solid lipid nanoparticles containing a hydrophobic drug: effects of process variables. Colloids Surf B Biointerfaces 88:483–9
- Date AA, Desai N, Dixit R, Nagarsenker M. (2010). Self-nanoemulsifying drug delivery systems: formulation insights, applications and advances. Nanomedicine (Lond) 5:1595–616
- Izquierdo P, Esquena J, Tadros TF, et al. (2004). Phase behavior and nano-emulsion formation by the phase inversion temperature method. Langmuir 20:6594–8
- Jordan M, Nayel A, Brownlow B, Elbayoumi T. (2012). Development and evaluation of tocopherol-rich argan oil-based nanoemulsions as vehicles possessing anticancer activity. J Biomed Nanotechnol 8:944–56
- Kakumanu S, Tagne JB, Wilson TA, Nicolosi RJ. (2011). A nanoemulsion formulation of dacarbazine reduces tumor size in a xenograft mouse epidermoid carcinoma model compared to dacarbazine suspension. Nanomedicine 7:277–83
- Kannappan R, Yadav VR, Aggarwal BB. (2010). Gamma-Tocotrienol but not gamma-tocopherol blocks STAT3 cell signaling pathway through induction of protein-tyrosine phosphatase SHP-1 and sensitizes tumor cells to chemotherapeutic agents. J Biol Chem 285:33520–8
- Karadzovska D, Riviere JE. (2013). Assessing vehicle effects on skin absorption using artificial membrane assays. Eur J Pharm Sci 50:569–76
- Kuo F, Timothy K, Wilson TA, et al. (2007). A nanoemulsion of an anti-oxidant synergy formulation reduces tumor growth rate in neuroblastoma-bearing nude mice. J Exp Ther Oncol 6:129–35
- McClements DJ, Decker EA, Weiss J. (2007). Emulsion-based delivery systems for lipophilic bioactive components. J Food Sci 72:R109–24
- Mostafa DM, Ammar NM, Abd El-Alim SH, El-anssary AA. (2014). Transdermal microemulsions of Glycyrrhiza glabra L.: characterization, stability and evaluation of antioxidant potential. Drug Deliv 21:130–9
- Muller L, Theile K, Bohm V. (2010). In vitro antioxidant activity of tocopherols and tocotrienols and comparison of vitamin E concentration and lipophilic antioxidant capacity in human plasma. Mol Nutr Food Res 54:731–42
- Muller RH, Petersen RD, Hommoss A, Pardeike J. (2007). Nanostructured lipid carriers (NLC) in cosmetic dermal products. Adv Drug Deliv Rev 59:522–30
- Ng SH, Woi PM, Basri M, Ismail Z. (2013). Characterization of structural stability of palm oil esters-based nanocosmeceuticals loaded with tocotrienol. J Nanobiotechnol 11:1–7
- Patacsil D, Tran AT, Cho YS, et al. (2012). Gamma-tocotrienol induced apoptosis is associated with unfolded protein response in human breast cancer cells. J Nutr Biochem 23:93–100
- Pham J, Brownlow B, Elbayoumi T. (2013). Mitochondria-specific pro-apoptotic activity of genistein lipidic nanocarriers. Mol Pharm 10:3789–800
- Phan V, Walters J, Brownlow B, Elbayoumi T. (2013). Enhanced cytotoxicity of optimized liposomal genistein via specific induction of apoptosis in breast, ovarian and prostate carcinomas. J Drug Target 21:1001–11
- Rajendran P, Li F, Manu KA, et al. (2011). Gamma-Tocotrienol is a novel inhibitor of constitutive and inducible STAT3 signalling pathway in human hepatocellular carcinoma: potential role as an antiproliferative, pro-apoptotic and chemosensitizing agent. Brit J Pharmacol 163:283–98
- Rass K, Tilgen W. (2008). Treatment of melanoma and nonmelanoma skin cancer. Adv Exp Med Biol 624:296–318
- Saberi AH, Fang Y, McClements DJ. (2013a). Effect of glycerol on formation, stability, and properties of vitamin-E enriched nanoemulsions produced using spontaneous emulsification. J Colloid Interface Sci 411:105–13
- Saberi AH, Fang Y, McClements DJ. (2013b). Fabrication of vitamin E-enriched nanoemulsions: factors affecting particle size using spontaneous emulsification. J Colloid Interface Sci 391:95–102
- Sarker DK. (2005). Engineering of nanoemulsions for drug delivery. Curr Drug Deliv 2:297–310
- Sen CK, Khanna S, Roy S. (2006). Tocotrienols: vitamin E beyond tocopherols. Life Sci 78:2088–98
- Sylvester PW, Kaddoumi A, Nazzal S, El Sayed KA. (2010). The value of tocotrienols in the prevention and treatment of cancer. J Am Coll Nutr 29:324S–33S
- Tadros T, Izquierdo P, Esquena J, Solans C. (2004). Formation and stability of nano-emulsions. Adv Colloid Interface Sci 108–109:303–18
- Thaipong K, Boonprako U, Crosby K, et al. (2006). Comparison of ABTS, DPPH, FRAP, and ORAC assays for estimating antioxidant activity from guava fruit extracts. J Food Comp Anal 19:669–75
- Zhang J, Michniak-Kohn B. (2011). Investigation of microemulsion microstructures and their relationship to transdermal permeation of model drugs: ketoprofen, lidocaine, and caffeine. Int J Pharm 421:34–44