Abstract
Superparamagnetic iron oxide nanoparticles (SPIONs) have been the subject of extensive research due to their potential biomedical applications. In the present investigation, superparamagnetic FA-PEI-Fe3O4 were successfully prepared and evaluated as a targeted MRI contrast agent. FTIR characteristics, TGA, VSM, and MR imaging confirmed the composition and magnetic properties of the synthesized nanoparticles. TEM showed that FA-PEI-Fe3O4 were spherical in shape and well dispersed. The nanoparticles were superparamagnetic at room temperature with a saturation magnetization value of 67.1 emu/g. The nanoparticles showed higher uptake efficiency due to receptor-mediated endocytosis. Moreover, specificity of FA-PEI-Fe3O4 to target tumor cells was demonstrated by the increased nanoparticle uptake and significant contrast enhancement of KB cells over MCF7 cells. The competitive inhibition of FA-PEI-Fe3O4 by free FA further confirmed the specific interaction of this conjugate with FA receptors. In vivo MR imaging studies showed a decreased signal intensity and enhanced tumor contrast post-injection of FA-PEI-Fe3O4. These results indicate that FA-PEI-Fe3O4 can be used as a promising tumor-targeting agent as well as a T2 negative-contrast agent in MR imaging applications.
Introduction
Superparamagnetic iron oxide nanoparticles (SPIONs) have been the subject of extensive research because of their non-toxicity, biocompatibility, low particle dimension, large surface area, and suitable magnetic properties (Landmark et al., Citation2008; Liu et al., Citation2010; Wang et al., Citation2012; Zhao et al., Citation2013; Pala et al., Citation2014; Li et al., Citation2014; Stepanov et al., Citation2014). As a contrast agent, SPIONs can greatly enhance the magnetic resonance imaging (MRI) contrast due to their superparamagnetic property and have gained attention in clinical use including MRI contrast enhancement, targeted drug delivery, cancer diagnosis, tissue repair, cell labeling and separation, immunoassay, and magnetic hyperthermia (Silva et al., Citation2011; Wu et al., Citation2014). SPIONs have a large surface area/volume ratio and tend to aggregate and adsorb plasma proteins, the direct use of SPIONs can result in biofouling of the particles in blood plasma and the formation of aggregates that are quickly eliminated by cells of the reticularendothelial system (RES) (Rogers & Basu, Citation2005). One possible approach to increase the circulation time of nanoparticles in the blood stream is to modify the nanoparticles with polymers and to minimize protein adsorption (Zhang et al., Citation2002).
A wide variety of polymer-modified SPIONs have been reported in published literatures, such as amphiphilic polymer-modified SPIONs (Yang et al., Citation2009), polysaccharide-modified SPIONs (Gupta & Gupta, Citation2005), and dendrimer-modified SPIONs (Shi et al., Citation2008). Most of them use complicated synthetic method to modify the SPIONs or use the cross-linked agent glutaraldehyde. The remaining glutaraldehyde may have an adverse effect on the human body. In this study, we described a simple and facile method to modified SPIONs using self-assembly of a positively charged poly(ethyleneimine) (PEI) polyelectrolyte through electrostatic attraction. The PEI coating not only serves as a protective biological layer to improve particle stability, particularly preventing particle agglomeration but also primary amine groups in its molecule facilitate the conjugation of tumor-targeting molecular or other functional molecules (Cai et al., Citation2013; Li et al., Citation2013). In this study, we chose folic acid (FA) as a targeting ligand to conjugate to the surface of the PEI coated the SPIONs for active targeting to tumors as magnetic resonance imaging (MRI) contrast agent.
Folic acid (FA) is a water-soluble vitamin B which is generally recognized as an effective tumor-targeting agent to conjugate with nanoparticles (Mishra & Jain, Citation2003; Bhattacharya et al., Citation2007; Zhang et al., Citation2010; Lee et al., Citation2013; Li et al., Citation2013). FA receptors exhibit limited expression on healthy cells but are often present in large numbers on cancer cells (Kamen & Capdevila, Citation1986). FA receptors are overexpressed on epithelial cancers of the ovary, mammary gland, colon, lung, prostate, nose, throat, and brain (Ross et al., Citation1994; Weitman et al., Citation1994). FA receptors are also overexpressed on hematopoietic malignancies of myeloid origin, including chronic and acute myelogeneous leukemias. The FA receptors density also appears to increase as the stage/grade of the cancer increases (Parker et al., Citation2005). An additional feature of FA receptors that is particularly attractive for intravenous drug delivery strategies is the fact that FA receptors generally become accessible to the vascular epithelium only after malignant transformation. This is because FA receptors are selectively expressed on the apical membrane surface of normal polarized epithelial cells and are, therefore, protected from FA receptors directed therapeutics delivered in the plasma (Dixit et al., Citation2006). FA has short chains and a small size, and thus facilitates the internalization of nanoparticles. Meanwhile, FA is stable, non-immunogenic, inexpensive, and, in addition, has a very high affinity for its cell surface receptors (Stella et al., Citation2000). Therefore, controlling the attachment of the ligand FA to SPIONs constitutes an important step for FA receptors-mediated targeted delivery of SPIONs.
In addition, magnetic resonance imaging (MRI) contrast agents are required to have high relaxivity (r2 = T2−1) for shortening the T2 effectively. Experiments have shown that the clustering of SPIONs is an effective approach for increasing r2 dramatically (Qiu et al., Citation2010; Min et al., Citation2012; Pöselt et al., Citation2012; Hayashi et al., Citation2013). In the study, we expected clustering of SPIONs coated by PEI polyelectrolyte have high MRI contrast effect due to its high relaxivity.
The synthesized SPIONs were characterized by Fourier transform infrared spectroscopy (FTIR), transmission electron microscope (TEM), thermogravimetric analysis (TGA), and vibrating sample magnetometry (VSM). The in vitro cellular uptake of FA-conjugated SPIONs was tested. The imaging potential of FA-conjugated SPIONs was investigated in vitro and in vivo.
Materials and methods
Materials
Ferric chloride hexahydrate (FeCl3·6H2O > 99%), ferrous chloride tetrahydrate (FeCl2ċ4H2O), poly(ethyleneimine) (PEI, MW 25 000), 1-ethyl-3-[3-(dimethylamino) propyl] carbodiimide (EDC), N-hydroxysuccinimide (NHS), and folic acid (FA) were obtained from Sigma-Aldrich (St. Louis, MO). All other chemical reagents were of analytical grade, obtained from commercial sources and used without further purification.
Synthesis of PEI-coated magnetic nanoparticles
Iron oxide (Fe3O4) nanoparticles were synthesized via a coprecipitation process of Fe (III) and Fe (II) with ammonium hydroxide reported previously (Gao et al., Citation2010) with minor modifications. Briefly, FeCl3·6H2O (11.60 g) and FeCl2·4H2O (4.30 g) were dissolved in 400 mL of deionized water under nitrogen gas protection with vigorous stirring at 85 °C. Subsequently, about 20 mL of ammonium hydroxide solution was added to the solution and stirring was continued till black precipitate was formed. The precipitate was then washed twice with ethanol and several times with distilled water till neutral pH was obtained.
The prepared iron oxide nanoparticles were redispersed in deionized water with vigorous stirring at 85 °C, a portion of PEI solution was added into the suspension, the mixture was stirred for 30 min at 85 °C under a nitrogen gas atmosphere. The magnetic nanoparticles were isolated by ultracentrifugation (Hitachi, CF 16RX) at 12 000 rpm for 10 min at 4 °C and washed three times with deionized water.
Conjugation reaction of FA with PEI-coated magnetic nanoparticles
N-Hydroxysuccinimide ester of FA (NHS-folate) was prepared according to a method previously described (Mornet et al., Citation2003). Briefly, 4 g of FA, 0.7 g of EDC, and 0.6 g of NHS were dissolved in 150 mL of deionized water. The pH of the solution was adjusted to 10 by the addition of 1.5 M NaOH and the mixture was allowed to react for 2 h at ambient temperature.
The PEI-coated magnetic nanoparticles were dispersed in a portion of deionized water then mixed with the above solution under constant stirring. After 24 h of incubation at ambient temperature, the solution was dialyzed against deionized water for 24 h using a dialysis tube (molecular weight cut-off 12 kDa). Then, the as-obtained FA-PEI-magnetic nanoparticles (FA-PEI-Fe3O4) were collected.
Characterization of nanoparticles
Fourier transform infrared spectroscopy (FTIR) spectra were acquired using a Nicolet 560 FTIR spectrometer (Welltech Scientific Inc., Bear Lake, MI) with a resolution of 4 cm−1 in the range 4000–400 cm−1. Samples of FA-PEI-Fe3O4 were air-dried then milled with KBr and the mixture was pressed into a pellet for analysis.
Transmission electron microscopy (TEM) samples were prepared by depositing a drop of the diluted particle suspension on to a carbon-coated copper grid and allowed to air dry. The grid was then measured by a transmission electron microscope (TEM; Tecnai G2 20S-Twin, Arbin Instruments, College Station, TX) at an accelerating voltage of 80 kV. The mean size of three kinds of magnetic nanoparticles was determined by dynamic light scattering (DLS) (Malvern Mastersizer 3000, Malvern Instruments Ltd, Worcestershire, UK) in deionized water. The surface potential of the three kinds of magnetic nanoparticles was measured by a Malvern Zetasizer Nano ZS model ZEN3000 (Malvern Instruments Ltd, Worcestershire, UK) equipped with a standard 633 nm laser.
The content of Fe3O4 in the FA-PEI-Fe3O4 was determined by thermogravimetric analysis (TGA) using a TA instruments Q500 TGA unit. The temperature of the sample gradually increased from 30 to 800 °C at a heating rate of 10 °C/min. The saturation of magnetization was evaluated using a vibrating sample magnetometer (VSM, DMA 1600, VSM, Adelphi, MD) at room temperature (RT). The applied magnetic field varied from 2 × 104 Oe to −2 × 104 Oe in order to obtain the hysteresis loops.
MR imaging
Magnetic resonance imaging (MRI) was used to assess the magnetic properties of FA-PEI-Fe3O4. A portion of FA-PEI-Fe3O4 was redispersed in phosphate buffered saline (PBS) and diluted at various concentrations of 50, 25, 15, 7.5, 5, 2.5, 1.25, and 0 mg mL−1. Then mixed with 1.5% (3:7, v/v) agar solution by vortexing and cooled in an ice bath and stored at 4 °C until analysis.
All MR images were performed with a 1.5 T clinical MRI instrument (Siemens Avanto Medical Systems, Berlin, Germany) at RT. A spin-echo multisection pulse sequence was selected. Various echo times (TE) from 20 ms to 120 ms with a repetition time (TR) of 5300 ms were used. The spatial resolution parameters were set as follows: an acquisition matrix of 432× 512, a field of view of 30.0 × 25.3 cm2, a section thickness of 3 mm, and two averages. The host software (Paravision ver 3.0.2, GE NMR Instruments, Fremont, CA) was used for data acquisition, reconstruction, and visualization/analysis of the images. After acquiring the images, the magnitudes of image intensities were measured within manually drawn regions of interest (ROIs) for each of the samples to obtain signal intensity measurements. R2 was defined as 1/T2 with the units of s−1. The relaxivity coefficient (mM−1 s−1) equals the ratio of R2 to the concentration of FA-PEI-Fe3O4.
Cell culture
KB cells, which are of high FA receptors expression, MCF7 cells, which are of middle-FA receptors expression, L929 cells, which are low-FA receptors expression, were employed. All cells were obtained from the cell bank of Peking Union Medical College. All cell lines were cultured in RPMI Medium 1640 (Gibco, Waltham, MA) containing 10% fetal bovine serum (FBS; Gibco, Waltham, MA), 100 units/mL penicillin, and 100 μg/mL streptomycin at 37 °C in a 5% CO2 and 95% air humidified atmosphere.
In Vitro cellular uptake
For quantitative cellular uptake analysis, KB cells, MCF7 cells, and L929 cells were initially plated and grown to 60% confluence in 75 cm2 flasks. Cells were then washed twice with PBS and cultured in 10 mL of FA-free RPMI-1640 medium containing 10% FBS, 100 units/mL penicillin, and 100 μg/mL streptomycin for 24 h. The growth medium was then aspirated and the cells were washed twice with PBS. Then, the medium was replaced with fresh medium containing FA-PEI-Fe3O4 at a concentration of 100 μg/mL. After incubation for 2 h, the medium was removed and the treated cells were washed three times with PBS, detached with trypsin–EDTA, and resuspended in 1 mL of PBS containing 5% FBS. For a comparative study of free FA competition, a portion of free FA was added to the fresh medium containing FA-PEI-Fe3O4 at a concentration of 100 μg/mL and then incubated with KB cells. After incubation for 2 h at 37 °C, cells were washed three times with PBS to remove free nanoparticles. Cell density and viability were determined through staining with trypan blue and counting cells using a hemocytometer with 0.9 mm3 counting chamber.
Intracellular uptake of the nanoparticles was quantified by inductively coupled plasma emission spectroscopy (ICP), where the iron content of each sample was analyzed. ICP samples were prepared by centrifuging down the cells and dissolving the cell pellet in 37% HCl at 70 °C for 1 h. Intracellular uptake experiments were performed in triplicate with percent error determined through calculating the standard deviation of these measurements.
In vitro MR imaging
T2-weighted signal intensities were measured with a 1.5 T clinical MRI instrument (Siemens Avanto Medical Systems, Berlin, Germany) with the concentration of the nanoparticles ranging from 0 to 200 μg/mL. KB cells were incubated with FA-PEI-Fe3O4 and PEI-Fe3O4 (diluted in FA-free RPMI-1640 medium) for 4 h at RT, meanwhile, MCF7 cells were incubated with FA-PEI-Fe3O4 (diluted in FA-free RPMI-1640 medium) for 4 h at RT. Then, the media were dispensed and the cells were washed three times with PBS containing 2% FBS. The T2-weighed images were acquired using the following parameters: point resolution: 432 × 512, section thickness: 3 mm, TE: 100 ms, TR: 5300 ms, number of acquisitions: 1.
In vivo MR imaging studies
All animal experiments were performed in compliance with the local ethics committee. Female BABL/c athymic nude mice (nu/nu, body weight, around 16 g), 4 weeks of age, were obtained from Beijing HFK Bioscience Co., Ltd (Beijing, China) and kept 5 mice/cage with water and food ad libitum according to Institutional Animal Care and Use Committee approval.
Tumor-bearing nude mice were prepared by subcutaneous injection of the 5 × 106 KB cells into the right later thigh of mice. Before the imaging procedure, mice were anaesthetized by trichloroacetaldehyde hydrate (10%) at the dose of 40 mg/kg body weight and maintained at normal body temperature. FA-PEI-Fe3O4 was diluted in normal saline containing 0.9% w/v NaCl to obtain an estimated injection volume. The mice were scanned before and after the administration of 150 μL FA-PEI-Fe3O4 solution in saline with a dose of 5 mg (Fe)/kg body weight through tail vein for 4 h. MR imaging studies were performed with a 1.5 T clinical MRI instrument (Siemens Avanto Medical Systems, Berlin, Germany) with the following parameters: point resolution: 512 × 512, slice thickness: 0.8 mm, TE: 5 ms, TR: 15 ms, DFOV: 349 × 349 mm2.
Statistical analysis
The statistical analysis of experimental data utilized Student's t-test with p < 0.05 as a significant difference. The experimental results are given in the format of mean ± standard deviations (SD).
Results and discussion
Preparation and characterization of FA-PEI-Fe3O4
Magnetic Fe3O4 nanoparticles were synthesized by coprecipitation of ferrous and ferric ions in aqueous solution by addition of ammonium hydroxide. PEI was then coated onto the surfaces of the magnetic nanoparticles through electrostatic attraction, the FA was subsequently conjugated with PEI-Fe3O4 via carbodiimide chemistry to prepare FA-PEI-Fe3O4.
FTIR spectroscopy was used to confirm that FA was successfully immobilized on the nanoparticles. FTIR spectra of Fe3O4 (), PEI-Fe3O4 (), and FA-PEI-Fe3O4 () are shown in . For the naked Fe3O4 (), the peak at 584 cm−1 can be attributed to the Fe–O groups corresponding to the presence of magnetite (Lin et al., Citation2009; Rastogi et al., Citation2011). clearly demonstrates the successful chemical conjugation since the characteristic peaks at 2928 cm−1, 2830 cm−1, and 1466 cm−1 related to asymmetry stretching vibration, symmetry stretching vibration, and deformation vibration of C–H bond of PEI backbone, respectively. In addition, the peak at 1644 cm−1 due to deformation vibration of primary amine of PEI backbone. Compared with , the absorption band at 1574 cm−1 attributed to the carboxylic acid of FA () which confirms that FA was successfully coated on the surface of PEI-Fe3O4.
shows the transmission electron microscopy (TEM) images of naked Fe3O4 (), PEI-Fe3O4 (), and FA-PEI-Fe3O4 (). The TEM images show the naked Fe3O4 nanoparticles were significant aggregation. The PEI-Fe3O4 nanoparticles were nearly spherical in shape and agglomerated to some extent. FA-PEI-Fe3O4 nanoparticles were nearly spherical in shape with an approximate particle size of 10 nm and well dispersed, and there were little differences in particle size between PEI-Fe3O4 and FA-PEI-Fe3O4. The average particle sizes were 60.8 nm ± 4.4 nm, 26.8 nm ± 6.1 nm, and 28.3 nm ± 6.1 nm for Fe3O4, PEI-Fe3O4, and FA-PEI-Fe3O4 nanoparticles, respectively. We believe that the larger particle size of Fe3O4 nanoparticles can be mainly attributed to particle aggregation which also can be seen from TEM images. The average diameters obtained by DLS were larger than the sizes determined from the TEM images for the corresponding samples. This is mainly because DLS determined the size in the hydrated state of the sample.
The zeta potential of magnetic nanoparticles was examined to further confirm the presence of PEI and FA on nanoparticles surface. The zeta potential of naked Fe3O4 nanoparticles was negative (zeta potential = −24.3 mV). After PEI coating, the zeta potential of Fe3O4 nanoparticles significantly increased to positive surface potential (zeta potential = 17.8 mV). After the conjugation of FA, the zeta potential of Fe3O4 nanoparticles decreased to 4.6 mV due to the conjugation of the Fe3O4 nanoparticles surface amine groups with FA.
describes the TGA profile of naked Fe3O4 (), PEI-Fe3O4 (), and FA-PEI-Fe3O4 (). For the naked Fe3O4, the initial 2–2.5% weight loss in the temperature range of 30–800 °C is due to the loss of residual water in the sample. For the PEI-Fe3O4, the weight loss is about 17.5 wt%. This might be due to the loss of PEI conjugated to the nanoparticles. In contrast, for the FA-PEI-Fe3O4 a significant weight loss was observed above 300 °C, the total weight loss is about 50 wt% at 500 °C. This can be attributed to decomposition of the organic components of the coating. The TGA results have further confirmed that FA was successfully coated on the surface of PEI-Fe3O4 and the content of the Fe3O4 in the FA-PEI-Fe3O4 was estimated to be about 37 wt%.
To determine the magnetic properties of three kinds of magnetic nanoparticles, magnetization measurements were performed using VSM at room temperature (RT). The applied magnetic field varied from 2 × 104 Oe to 2 × 104 Oe in order to obtain the hysteresis loops. According to the magnetization measurement, no hysteresis was observed, which indicates that the FA-PEI-Fe3O4 retain the superparamagnetic property (). The saturation magnetization of the naked Fe3O4, PEI-Fe3O4 and FA-PEI-Fe3O4 was determined to be 68.7 emu/g, 68.2 emu/g, and 67.1 emu/g, respectively. Which indicates that PEI and FA coating did not significantly decreased the saturation magnetization of magnetic nanoparticles. The MS value of the FA-PEI-Fe3O4 was similar to that of FA-PF127-PAAIO (Folic acid-Pluronic F127-polyacrylic acid-bound magnetic iron oxide nanoparticles) reported by Lin et al. (Citation2009) and was higher than those reported by Hayashi et al. (Citation2013). The higher MS of the FA-PEI-Fe3O4 is beneficial for cancer imaging.
MR imaging characteristics of magnetic nanoparticles
MRI was used to assess the magnetic properties of the FA-PEI-Fe3O4 as a novel contrast agent. The T2-weighted MRI of the FA-PEI-Fe3O4 at a 1.5 T clinical MRI instrument presented significant color change with a variation in particle concentration (). It can be seen that FA-PEI-Fe3O4 exhibited significant negative contrast enhancement as the concentration increased from 0 to 50 mg mL−1 and the relaxation rate, R2 = 1/T2, was linearly proportional to the particle concentration (). The relaxivity coefficient was calculated to be 135.78 mM−1 s−1 according to per millimole of iron, which was higher than the literature reported that of the Fe3O4 nanoparticles coated with polymer multilayers (Shi et al., Citation2008). The higher relaxivity coefficient is essential for them to be used as good T2 negative contrast agent for sensitive MR imaging applications.
Cellular uptake of magnetic nanoparticles
To test the targeting efficacy of the FA-PEI-Fe3O4, KB cells, MCF7 cells, and L929 cells were used. As shown in , the MCF7 cells demonstrated more than two times higher uptake with FA-PEI-Fe3O4 than that of the L929 cells after 2 h of incubation. However, the KB cells demonstrated approximately six times uptake with FA-PEI-Fe3O4 than that of the L929 cells. This might be due to the high metabolic activity of KB cells leading to the over expression of the FA receptors on the cell surface and the low metabolic activity of L929 cells. For the PEI-Fe3O4, KB cells show much lower cells uptake than that in FA-PEI-Fe3O4 case. These results corroborate that the FA-PEI-Fe3O4 can facilitate the recognition of FR positive cancer cells and enhance the tumor cells uptake.
Figure 6. Cellular uptake of FA-PEI-Fe3O4 and PEI-Fe3O4 without free FA or with free FA by KB cells, MCF7 cells and L929 cells. (*p < 0.05).
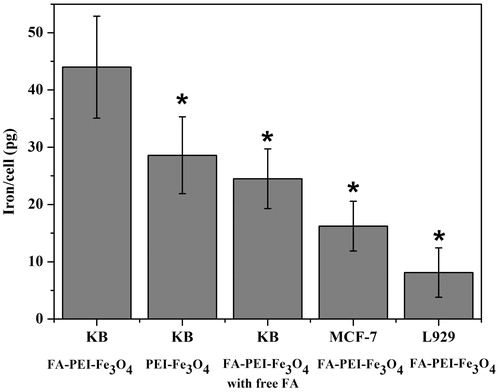
The specific targeting of the FA-PEI-Fe3O4 by FA receptors was further verified by a competitive inhibition assay, in which KB cells were cultured with FA-PEI-Fe3O4 mixed with free FA. It can be seen that a remarkable decrease nanoparticle uptake by KB cells was observed when the free FA was added in culture medium (). The results indicate that with free FA serving as a competitive inhibitor, the uptake of FA-PEI-Fe3O4 was reduced due to decreased availability of the FA receptors on the cell surface. This in turn suggests that FA-PEI-Fe3O4 is specifically targeted at the FA receptors.
In vitro MR imaging studies
To check the MRI efficiency of the FA-PEI-Fe3O4 as a novel contrast agent, the T2-weighted MRI of the FA-PEI-Fe3O4 and PEI-Fe3O4 was measured at a 1.5 T clinical MRI instrument at room temperature. In general, we incubated KB cells and MCF7 cells in the presence of FA-PEI-Fe3O4 and PEI-Fe3O4, respectively, and then compared their T2-weighted MR images. As shown in , the T2-weighted MRI of the MCF-7 cells treated with FA-PEI-Fe3O4 shows little change in MR contrast with increased particle concentration. In contrast, the MRI of the KB cells incubated with FA-PEI-Fe3O4 exhibits significant negative contrast enhancement as the concentration of the nanoparticles increased. This indicates that the KB cells had uptaken more FA-PEI-Fe3O4 compared with the MCF7 cells. However, the MRI of the KB cells treated with PEI-Fe3O4 shows lower negative contrast enhancement compared with that of FA-PEI-Fe3O4 at same concentration. This trend correlates well with the results of cellular uptake experiments MCF7 cell described above. These results indicate that FA-PEI-Fe3O4 play an important role in receptor-mediated endocytosis into the FA receptors over expression cells.
In vivo MR-imaging studies of animal model
In consideration of both in vitro cellular uptake and MR-imaging studies of magnetic nanoparticles indicate that FA-PEI-Fe3O4 can target FA receptors over expression KB tumor cells. We then examined the in vivo targeting magnetic resonance imaging capabilities of FA-PEI-Fe3O4 in mice-bearing subcutaneous KB xenografted tumors.
shows the T2-weighted MR images of the mice bearing subcutaneous KB xenografted tumors, acquired before () and 4 h after () intravenous administration of the FA-PEI-Fe3O4. It can be seen that the phantom images of post-injection of FA-PEI-Fe3O4 () become darkened significantly compared with that pre-injection of FA-PEI-Fe3O4 (). This hypointense region indicates the presence of magnetic nanoparticles within the tumor tissue. From these results, it could be concluded that the FA-PEI-Fe3O4 enhanced the contrast in the tumor tissue which can be used as a T2 negative-contrast agent in MR imaging applications.
Conclusions
In summary, superparamagnetic FA-PEI-Fe3O4 were successfully prepared and evaluated as a targeted MRI contrast agent. FTIR characteristics, TGA, VSM, In vitro, and In vivo MR imaging confirmed the composition and magnetic properties of the synthesized nanoparticles. The saturated magnetization of the composite nanoparticles reached as high as 67.1 emu/g and the nanoparticles showed superparamagnetism. TEM showed that FA-PEI-Fe3O4 were spherical in shape and well dispersed. Moreover, for the FA-PEI-Fe3O4, the receptor-mediated endocytosis can further facilitate the cellular uptake, resulting in higher uptake efficiency. Specificity of FA-PEI-Fe3O4 to target tumor cells was demonstrated by the increased nanoparticle uptake and significant contrast enhancement of KB cells over MCF7 cells. The competitive inhibition of FA-PEI-Fe3O4 by free FA further confirmed the specific interaction of this conjugate with FA receptors. T2-weighted MR images showed that the MR signal in the tumor tissue became darkened significantly 4 h after intravenous administration compared with that pre-injection of FA-PEI-Fe3O4.
Based on these data, we concluded that FA-PEI-Fe3O4 have great potential as an effective tumor-targeting agent and could be evaluated as a promising MRI contrast agent that specifically targets FA receptors overexpressing tumor cells. Further studies are in progress to test the targeting efficacy and stability in vivo.
Declaration of interest
The authors report that they have no conflicts of interest to report. The authors gratefully acknowledge the financial support of this research by the National Key Basic Research Program of China (2013CB932703), the Natural Science Foundation of China (81472851, 81101743, 21425522, 31200751, and 31300827), and Beijing Natural Science Foundation (7152158).
References
- Bhattacharya R, Patra CR, Earl A, et al. (2007). Attaching folic acid on gold nanoparticles using noncovalent interaction via different polyethylene glycol backbones and targeting of cancer cells. Nanomedicine 3:224–38
- Cai HD, An X, Cui J, et al. (2013). Facile hydrothermal synthesis and surface functionalization of polyethyleneimine-coated iron oxide nanoparticles for biomedical applications. ACS Appl Mater Interfaces 5:1722–31
- Dixit V, Van den Bossche J, Sherman DM, et al. (2006). Synthesis and grafting of thioctic acid-PEG-folate conjugates onto Au nanoparticles for selective targeting of folate receptor-positive tumor cells. Bioconjug Chem 17:603–9
- Gao FP, Cai YY, Zhou J, et al. (2010). Pullulan Acetate coated magnetite nanoparticles for hyperthermia: preparation, characterization and in vitro experiments. Nano Res 3:23–31
- Gupta AK, Gupta M. (2005). Cytotoxicity suppression and cellular uptake enhancement of surface modified magnetic nanoparticles. Biomaterials 26:1565–73
- Hayashi K, Nakamura M, Sakamoto W, et al. (2013). Superparamagnetic nanoparticle clusters for cancer theranostics combining magnetic resonance imaging and hyperthermia treatment. Theranostics 6:366–76
- Kamen BA, Capdevila A. (1986). Receptor-mediated folate accumulation is regulated by the cellular folate content. Proc Natl Acad Sci USA 83:5983–7
- Landmark KJ, DiMaggio S, Ward J, et al. (2008). Synthesis, characterization, and in vitro testing of superparamagnetic iron oxide nanoparticles targeted using folic acid-conjugated dendrimers. ACS Nano 2:773–83
- Lee KY, Seow E, Zhang Y, Lim YC. (2013). Targeting CCL21-folic acid-upconversion nanoparticles conjugates to folate receptor-α expressing tumor cells in an endothelial-tumor cell bilayer model. Biomaterials 34:4860–71
- Li JC, He Y, Sun WJ, et al. (2014). Hyaluronic acid-modified hydrothermally synthesized iron oxide nanoparticles for targeted tumor MR imaging. Biomaterials 35:3666–77
- Li JC, Zheng LF, Cai HD, et al. (2013). Polyethyleneimine-mediated synthesis of folic acid-targeted iron oxide nanoparticles for in vivo tumor MR imaging. Biomaterials 34:8382–92
- Lin JJ, Chen JS, Huang SJ, et al. (2009). Folic acid-Pluronic F127 magnetic nanoparticle clusters for combined targeting, diagnosis, and therapy applications. Biomaterials 30:5114–24
- Liu YT, Li K, Pan J, et al. (2010). Folic acid conjugated nanoparticles of mixed lipid monolayer shell and biodegradable polymer core for targeted delivery of Docetaxel. Biomaterials 31:330–8
- Min C, Shao H, Liong M, et al. (2012). Mechanism of magnetic relaxation switching sensing. ACS Nano 6:6821–8
- Mishra PR, Jain NK. (2003). Folate conjugated doxorubicin-loaded membrane vesicles for improved cancer therapy. Drug Deliv 10:277–82
- Mornet S, Portier J, Duguet E. (2003). Ferrofluides stables en milieu neutre et ferrofluides modifiés obtenus par modification de la surface des particules de ces ferrofluides. French Patent 0306279
- Pala K, Serwotka A, Jeleń F, et al. (2014). Tumor-specific hyperthermia with aptamer-tagged superparamagnetic nanoparticles. Int J Nanomed 9:67–76
- Parker N, Turk MJ, Westrick E, et al. (2005). Folate receptor expression in carcinomas and normal tissues determined by a quantitative radioligand binding assay. Anal Biochem 338:284–93
- Pöselt E, Kloust H, Tromsdorf U, et al. (2012). Relaxivity optimization of a PEGylated iron-oxide-based negative magnetic resonance contrast agent for T2-weighted spin-echo imaging. ACS Nano 6:1619–24
- Qiu P, Jensen C, Charity N, et al. (2010). Oil phase evaporation-induced self-assembly of hydrophobic nanoparticles into spherical clusters with controlled surface chemistry in an oil-in-water dispersion and comparison of behaviors of individual and clustered iron oxide nanoparticles. J Am Chem Soc 132:17724–32
- Rastogi R, Gulati N, Kotnala RK, et al. (2011). Evaluation of folate conjugated pegylated thermosensitive magnetic nanocomposites for tumor imaging and therapy. Colloids Surf B Biointerfaces 82:160–7
- Rogers WJ, Basu P. (2005). Factors regulating macrophage endocytosis of nanoparticles: implications for targeted magnetic resonance plaque imaging. Atherosclerosis 178:67–73
- Ross JF, Chaudhuri PK, Ratnam M. (1994). Differential regulation of folate receptor isoforms in normal and malignant tissues in vivo and in established cell lines. Physiologic and clinical implications. Cancer 73:2432–43
- Shi X, Wang SH, Swanson SD, et al. (2008). Dendrimer-functionalized shell-crosslinked iron oxide nanoparticles for in-vivo magnetic resonance imaging of tumors. Adv Mater 20:1671–8
- Silva AC, Oliveira TR, Mamani JB, et al. (2011). Application of hyperthermia induced by superparamagnetic iron oxide nanoparticles in glioma treatment. Int J Nanomed 6:591–603
- Stella B, Arpicco S, Peracchia MT, et al. (2000). Design of folic acid-conjugated nanoparticles for drug targeting. J Pharm Sci 89:1452–64
- Stepanov A, Burilov V, Pinus M, et al. (2014). Water transverse relaxation rates in aqueous dispersions of superparamagnetic iron oxide nanoclusters with diverse hydrophilic coating. Colloids Surf A Physicochem Eng Aspects 443:450–8
- Wang C, Ravi S, Martinez GV, et al. (2012). Dual-purpose magnetic micelles for MRI and gene delivery. J Control Release 163:82–92
- Weitman SD, Frazier KM, Kamen BA. (1994). The folate receptor in central nervous system malignancies of childhood. J Neurooncol 21:107–12
- Wu QH, Meng N, Zhang YR, et al. (2014). The effect of two novel amino acid-coated magnetic nanoparticles on survival in vascular endothelial cells, bone marrow stromal cells, and macrophages. Nanoscale Res Lett 9:461
- Yang X, Pilla S, Grailer JJ, et al. (2009). Tumor-targeting, superparamagnetic polymeric vesicles as highly efficient MRI contrast probes. J Mater Chem 19:5812–17
- Zhang HZ, Li XM, Gao FP, et al. (2010). Preparation of folate-modified pullulan acetate nanoparticles for tumor-targeted drug delivery. Drug Deliv 17:48–57
- Zhang Y, Kohler N, Zhang M. (2002). Surface modification of superparamagnetic magnetite nanoparticles and their intracellular uptake. Biomaterials 23:1553–61
- Zhao M, Li AM, Chang J, et al. (2013). Develop a novel superparamagnetic nano-carrier for drug delivery to brain glioma. Drug Deliv 20:95–101