Abstract
Doxorubicin (DOX) is a potent anticancer drug for the treatment of tumors, but the poor specificity and multi-drug resistance (MDR) on tumor cells have restricted its application. Here, a pH and reduction-responsive peptide–drug conjugate (PDC), pHLIP-SS-DOX, was synthesized to overcome these drawbacks. pH low insertion peptide (pHLIP) is a cell penetrating peptide (CPP) with pH-dependent transmembrane ability. And because of the unique cell membrane insertion pattern, it might reverse the MDR. The cellular uptake study showed that on both drug-sensitive MCF-7 and drug-resistant MCF-7/Adr cells, pHLIP-SS-DOX obviously facilitated the uptake of DOX at pH 6.0 and the uptake level on MCF-7/Adr cells was similar with that on MCF-7 cells, indicating that pHLIP-SS-DOX had the ability to target acidic tumor cells and reverse MDR. In vitro cytotoxicity study mediated by GSH-OEt demonstrated that the cytotoxic effect of pHLIP-SS-DOX was reduction responsive, with obvious cytotoxicity at pH 6.0; while it had poor cytotoxicity at pH 7.4, no matter with or without GSH-OEt pretreatment. This illustrated that pHLIP could deliver DOX into tumor cells with acidic microenvironment specifically and could not deliver drugs into normal cells with neutral microenvironment. In summary, pHLIP-SS-DOX is a promising strategy to target drugs to tumors and provides a possibility to overcome MDR.
Introduction
Doxorubicin (DOX), also known as adriamycin, is a prominent member of the family of anthracycline antibiotics. Since its first isolation from a strain of pigment-producing Streptomyces in 1960s, it has become one of the most widely used anticancer drugs in the clinical treatment of various types of cancer, including breast tumors (Kratz et al., Citation1998; Yoo et al., Citation2000; Girotti & Minotti, Citation2013). DOX exerts its cytotoxic activity by intercalation into DNA, inhibition of the activity of topoisomerase II, resulting in DNA damage and induction of apoptosis (Ai et al., Citation2011; Soudy et al., Citation2013). In spite of the potent anticancer activity, the clinical application of DOX is limited by two main factors, dose-limiting cardiotoxicity and drug resistance (Maksimenko et al., Citation2014).
It is well-known that the tumor microenvironment has some features that are different from healthy tissues, such as a lowered extracellular interstitial pH, a higher intracellular glutathione (GSH) concentration or an increased expression of certain enzymes, such as matrix metalloproteinases (Mura et al., Citation2013) and these features can be used as endogenous stimuli to develop tumor-specific anticancer drug delivery systems. The extracellular pH in solid tumors (6.5–7.2) is lower than that in normal tissues (∼7.4) as a result of the “Warburg effect” (Zhao et al., Citation2012). Since the pH differences between the solid tumor and normal tissues (or blood) are ubiquitous regardless of the tumor heterogeneity, pH-responsive systems have been investigated extensively (Drachuk et al., Citation2012; Pan et al., Citation2012; Wilson et al., Citation2013). At the same time, reduction-responsive drug delivery systems have also received more and more attention for its site-specific drug release characteristics. Reduction-responsive drug carriers generally contain a disulfide bond which is reduced to sulfydryl by reducing agent, such as GSH, but maintains stable under normal conditions. The intracellular GSH concentration (2–10 mM) is substantially higher than that in the extracellular environment (2–10 μM), which is the main basis of reduction-responsive drug delivery systems (Geng et al., Citation2012; Bao et al., Citation2014). Furthermore, the GSH concentration in tumor tissues is several times higher than that in normal tissues (Kuppusamy et al., Citation2002), which is more helpful to break the disulfide bond between the drug and its carrier, resulting in more rapid drug release at the tumor site. These facts make the reduction-responsive drug delivery systems been widely used in the study of tumor targeting.
Cell penetrating peptides (CPPs), also known as protein transduction domains, are relatively short peptide sequences (5–30 amino acids in length) that are able to penetrate the cell membrane at low concentrations and deliver various types of cargoes into cells (Vasconcelos et al., Citation2013). The human immunodeficiency virus type 1 (HIV-1) encoded transactivator of transcription (Tat) peptide (Nasrollahi et al., Citation2012) was the first discovered sequence capable of crossing cell membranes and since then researchers had paid more and more attention to CPPs. There are different classifications of CPPs based on different classification criteria, such as their origins (Bechara & Sagan, Citation2013) or physico-chemical properties (Milletti, Citation2012). Here, we divide them into two big classes: traditional CPPs and pH-sensitive CPPs. Traditional CPPs are pH-insensitive CPPs including arginine-rich CPPs, amphipathic CPPs and so on, they possess the ability to deliver drugs or nanocarriers into cells but their primary obstacle was the lack of selectivity, they can penetrate all kinds of cell membranes without screening (Zhang et al., Citation2011). pH-sensitive CPPs taking advantage of the pH difference between tumor tissues and normal tissues are a group of CPPs sharing several common characteristics as follows: their cell-penetrating capacity is concealed at neutral pH, such as in normal tissues and blood circulations; when pH decrease, such as in tumor tissues their cell penetrating capacity is recovered through various mechanisms. Therefore, pH-sensitive CPPs provide a strategy to overcome the non-specific shortcoming caused by traditional CPPs. The widely used pH-sensitive CPPs include GALA (a peptide composed of repeating sequences of Glu-Ala-Leu-Ala), histidine-containing peptides, pH low insertion peptide (pHLIP) and other pH-sensitive peptides (He et al., Citation2013).
pHLIP, a 36-amino acid peptide derived from the C-helix of bacteriorhodopsin, is a water-soluble peptide which can insert into cell membranes through conformational transitions at acidic pH conditions. Under different pH conditions, pHLIP has three states: at neutral and high pH values without lipid bilayers, pHLIP is soluble in water (state I); at neutral and high pH values with the presence of lipid bilayers, it bounds to the surface of a membrane in a largely unstructured conformation (state II); and in an acidic environment with lipid bilayers, it inserts across the membrane as an α-helix (state III) (Hunt et al., Citation1997; Andreev et al., Citation2007). It has been demonstrated that pHLIP exists as a monomer at concentrations less than 8–10 μM and does not induce membrane fuse or leakage even at high concentrations (Reshetnyak et al., Citation2007). The insertion behavior of pHLIP has the following characteristics: reversible, the elevated pH induces the loss of helical structure and release from the lipid bilayers; unidirectional, the C-terminus is translocated into a cell or vesicle whereas the N-terminus stays outside (Andreev et al., Citation2010). The mechanism of pHLIP insertion is related to the protonation of two aspartic residues in the transmembrane region at acidic conditions (Fendos & Engelman, Citation2012) and the insertion process is accompanied with an energy release of about 1.8 kcal/mol in addition to the binding energy (about 7 kcal/mol), which can be used to deliver the cargoes conjugated to its C-terminus across cell membranes (Reshetnyak et al., Citation2008).
Multi-drug resistance (MDR) is the resistance of cancer cells to chemotherapeutic drugs. The emergence of MDR is mainly because the expression of adenosine triphosphate (ATP)-binding cassette (ABC) transporters on the cytoplasmic side of the resistant cell membranes, resulting in an increased drug efflux (Wu et al., Citation2014). Some researchers have reported that peptide–drug conjugate (PDC) is an effective way for overcoming MDR as the conjugate displays a mode of entry different from the drug itself (Dubikovskaya et al., Citation2008). Since the entry or insertion of pHLIP is not mediated by endocytosis, binding to cell receptors or formation of pores in cell membranes, but the formation of α-helix (Andreev et al., Citation2007) and DOX is translocated into cells directly, we expected that pHLIP might reverse MDR.
Here, we designed a PDC taking advantage of pHLIP through the GSH-responsive linker disulfide bond, called pHLIP-SS-DOX. It combined the benefits of tumor targeting mediated by pHLIP and site-specific drug release based on reduction-responsive disulfide bond. And it was expected to have the ability to reverse MDR because of the unique entry mode. The basic principle of the conjugate is shown in . At physiological pH, pHLIP-SS-DOX interacts weakly with the cell membrane and cannot enter the cells. At low pH, through the formation of a transmembrane helix with its C-terminus inserted in the cytoplasm, pHLIP carries the anticancer drug DOX into tumor cells directly, then the disulfide bond is cleaved under intracellular reductive environments to release DOX. The cellular uptake behavior of pHLIP-SS-DOX was characterized on DOX-sensitive MCF-7 cells and DOX-resistant MCF-7/Adr cells to evaluate its ability to reverse MDR.
Figure 1. Schematic diagram of the delivery of drugs into a cell. At physiological pH, the peptide–drug conjugates interact weakly with a membrane. At low pH, the peptide forms a transmembrane helix with its C-terminus inserted in the cytoplasm, the intracellular reductive environment breaks the disulfide bond to release the drug.
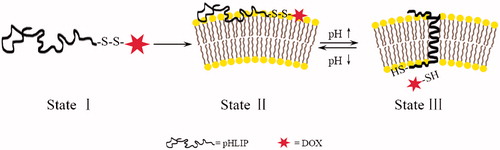
Materials and methods
Materials
The human breast cancer cell line MCF-7 was acquired from the Institute of Materia Medica, Chinese Academy of Medical Sciences and Peking Union Medical College (CAMS, Beijing, China) and the DOX-resistant breast cancer cell line MCF-7/Adr was acquired from the Institute of Hematology and Blood Diseases Hospital, Chinese Academy of Medical Sciences and Peking Union Medical College (CAMS, Beijing, China). 2,2′-Dithiodipyridine (Py-SS-Py), N,N-diisopropyl-ethylamine (DIEA) and O-benzotriazole-N,N,N′,N′-tetramethyluronium hexafluorophosphate (HBTU) were purchased from J&K Scientific Ltd. (Beijing, China). 3-Mercaptopropionic acid (MPA) was obtained from ACROS Organics (Morris Plains, NJ). DOX hydrochloride was purchased from SinaSun biotech. Co. Ltd. (Beijing, China). Di(N-succinimidyl)3,3′-dithiodipropionate (DSP) was obtained from TCI (Tokyo, Japan), pHLIP and FITC-pHLIP (labeled at the N-terminus) were synthesized by China Peptides Co., Ltd. (ShangHai, China). LDH cytotoxicity assay kit was from Bao RJ Technology Co., Ltd. (Beijing, China). Dithiothreitol (DTT) was obtained from Lan-Yi Co., Ltd. (Beijing, China). Sulforhodamine B (SRB), trichloroacetic acid (TCA) and Tris base were from Sigma-Aldrich (St. Louis, MO). Hoechst 33258 was obtained from Beyotime (Haimen, Jiangsu, China). RPMI 1640 cell culture solution, penicillin, streptomycin and phosphate buffered saline (PBS) were supplied by Mcgene Co. (Beijing, China). All other chemicals were of analytical or HPLC grade.
Synthesis of pHLIP-SS-DOX
Synthesis of 2-pyridyl-2-carboxyethyl disulfide (Py-SS-MPA)
Py-SS-MPA was synthesized and purified by modifying a literature method (Xie et al., Citation2005). Briefly, to a solution of Py-SS-Py (3.75 g, 17 mmol) dissolved in ethanol (30 mL), 0.4 mL of acetic acid was added. Then MPA (739 μL, 8.5 mmol) dissolved in ethanol (20 mL) was added dropwise over a period of 20–30 min. The reaction was under nitrogen protection at room temperature for 2 h. Then the solvent was evaporated under reduced pressure to yield a viscous yellow oil. This crude product was then separated from the remnant materials and the accompanying byproducts by a basic Al2O3 column. The column was washed with CH2Cl2/ethanol (3/2) to elute remnant materials and byproducts and was then eluted with CH2Cl2/ethanol (3/2) containing 4% acetic acid to obtain the target product. After removing the solvent under high vacuum, a colorless viscous oil was produced. Then the oil was purified through a process of recrystallization with water to obtain a white precipitate, which was then dried in vacuo overnight. This product was characterized by 1H NMR and ESI/MS.
Synthesis of Py-SS-DOX
Py-SS-DOX was synthesized by modifying a literature method (Chhikara et al., Citation2011). To a solution of Py-SS-MPA (29.1 mg, 0.135 mmol) in DMF, HBTU (102.6 mg, 0.27 mmol) was added to activate the carboxyl of Py-SS-MPA. The activating reaction lasted for 30 min. Then DOX·HCl (93.96 mg, 0.162 mmol) suspended in DMF was added slowly to the reaction mixture. Next, DIPEA was added to the reaction at room temperature. The reaction mixture was stirred under nitrogen atmosphere for 3 h. After completion of the reaction, 50 mL of cold anhydrous diethyl ether was added to produce a red precipitate. The crude product was purified by column chromatography over silica gel using DCM/methanol (20/1) as the eluents to afford product. The product was characterized by 1H NMR, ESI/MS, HPLC and Fourier transform infrared (FTIR) spectrometry.
Synthesis of pHLIP-SS-DOX
pHLIP-SS-DOX was prepared through thiol-disulfide reaction. Briefly, Py-SS-DOX (7 mg, 0.0095 mmol) was dissolved in DMF and then pHLIP was added dropwise. The reaction was stirred under nitrogen atmosphere at room temperature for 48 h. Then the reaction liquid was transferred to a dialysis tube (MWCO 2000) to dialyze exhaustively against 50% ethanol and distilled water to remove excess Py-SS-DOX and byproducts. Finally, the solution in the dialysis tube was lyophilized to obtain the final product and stored at −20 °C for further use. The product was characterized by MALDI-TOF-MS, HPLC and UV spectrometry (R, K. C. et al., Citation2012).
Synthesis of DOX-SH
To a solution of DOX·HCl (60.9 mg, 0.105 mmol) in DMF, DSP (20.2 mg, 0.05 mmol) was added dropwise at room temperature, a catalytic amount of TEA (14.6 μL, 0.105 mmol) was added to the reaction mixture. After the reaction lasted at room temperature overnight, the reaction mixture was transferred to 50 mL of cold anhydrous diethyl ether. The resulting red precipitate was washed twice with 5 mL of anhydrous diethyl ether. The solid red product was collected by filtration. The product was characterized by ESI/MS and HPLC. A solution of DTT was added to the product when DOX-SH was required for the experiment (Santra et al., Citation2011; Shi et al., Citation2012).
Verification the property of pHLIP
Liposome preparation
To investigate the interaction of pHLIP with cell membranes, we prepared blank liposome composed of lecithin and cholesterol to simulate cell membranes. Liposome was prepared by thin lipid film hydration method and the lipid composition was EPC/cholesterol at 4/1 (w/w). Briefly, lipids were dissolved in 30 mL of chloroform in a pear-shaped flask and evaporated at 37 °C on a rotary evaporator for 40 min. The obtained dried lipid film was hydrated with 1 mL of phosphate buffer solution, followed by sonication for 30 min. External buffer was exchanged by eluting through a Sephadex G50 column equilibrated with PBS (pH 7.4).
pHLIP insertion process at low pH
Insertion process was monitored by measuring the tryptophan (Trp) fluorescence (Hunt et al., Citation1997). Insertion process is accompanied by the translocation of Trp fluorophores from the aqueous solution into the hydrophobic environment of a lipid bilayer, such a change of polarity will cause an enhancement of emission fluorescence and a shift of the spectrum to short wavelengths. Measurements were carried out using a Cary Eclipce fluorescence spectrophotometer. Samples were prepared as follows. First, we prepared the solution of pHLIP in PBS with pH 8.0. And then liposomes were added to the solution and incubated at room temperature in the dark at pH 8.0 for 3 h. Last, to generate the pHLIP insertion, pH was reduced to 4.0 to observe the insertion behavior obviously by the addition of HCl solution. All measurements were performed at room temperature and with pHLIP and liposome at concentrations of 20 μg/ml, respectively. The widths of the emission and excitation slits were set to 5 nm. When tryptophan emission was monitored (pHLIP has two Trp residues), the samples were excited at 295 nm and the emission spectra were taken from 310 to 400 nm.
Formation of α-helix at low pH
Formation of α-helix was monitored by CD spectroscopy which can reflect the secondary structure of peptides (Tetin et al., Citation2003). CD spectrum of pHLIP was recorded on a Jasco (Easton, MD) 810 spectrometer. Samples were prepared as described in section “pHLIP insertion process at low pH”. All measurements were performed with pHLIP and liposome at concentrations of 20 μg/ml, respectively. CD intensities were expressed in mean millidegree. Samples were measured in a 0.1 cm path length quartz cuvette and raw data were acquired from 250 to 200 nm at 0.2 nm intervals using a 2 s averaging time, and at least three scans were averaged for each sample (Thevenin et al., Citation2009).
Interaction of pHLIP with cells
Confocal microscopy was used to observe the interaction of pHLIP with cells at different pH conditions. Following the culturing of MCF-7 cells for 24 h on 14 mm2 glass coverslips that were placed in culture dishes, FITC-pHLIP was added to incubate with cells at pH 7.4 or pH 6.0 for another 3 h. At the end of incubation, cells were washed with cold PBS, fixed with 4% paraformaldehyde for 30 min and stained with Hoechst 33258 (excitation/emission wavelength: 352/461 nm) for 30 min at 37 °C. Then the fluorescent images of cells were analyzed using a laser scanning confocal microscope (LSCM, LEICA, TCS SP5, Germany).
In vitro cellular uptake studies of the conjugate
LDH leakage assay
LDH leakage assay was used to test the membrane integrity after incubation with pHLIP conjugate at different pH values. MCF-7 cells were seeded in 96-well plate at a density of 8 × 103 cells/well for 24 h. Then the medium was removed, and serum-free medium adjusted to pH 7.4, 6.0, 5.0 or 4.0 containing pHLIP-SS-DOX at a concentration of 7 μM was added to incubate at 37 °C for 3 h. One hour before the end of incubation, we took out the plate and added the LDH release reagent to the positive control wells, the amount of LDH release agent added to each well was 10% of the original culture fluid volume. After mixed evenly by repeatedly beat of the control wells the plate was continued to incubate at 37 °C for 1 h. After that, the cell culture medium of each well was centrifuged at 400 g for 5 min, and 120 μL of supernatant of each well was transferred to a new plate to incubate with 60 μL newly prepared LDH detection working solution in dark place for 30 min. Fluorescene was measured at 490 nm. Untreated cells were defined as no leakage and 100% leakage was defined as total LDH release by treating LDH release reagent (Zhang et al., Citation2011).
Flow cytometry
Cellular uptake of the conjugates was quantitatively evaluated using flow cytometry. Briefly, MCF-7 and MCF-7/Adr cells (2.5 × 105 cells/mL) in RPMI 1640 medium were placed at 37 °C, 5% CO2, 95% air and 100% RH in glass bottom dishes for 24 h and then treated with serum-free medium diluted solution of DOX, pHLIP-SS-DOX, Py-SS-DOX, DOX-SH (at a DOX concentration of 7 μM) at pH 7.4 or pH 6.0 for 3 h. At the end of the treatment, the medium was removed and cells were rinsed with cold PBS (pH 7.4) twice, then tyrisin was added to detach the cells. After collected by centrifugation, cells were washed twice with cold PBS, resuspended with PBS and analyzed on FACScan flow cytometer (Becton Dickinson, San Jose, CA). The data were analyzed with FACStation software program. The number of cells collected was 10 000, and each assay was carried out in triplicate.
Confocal microscopy analysis
Confocal microscopy was used to observe the cellular internalization and distribution of different conjugates directly. Briefly, following the culturing of MCF-7 and MCF-7/Adr cells for 24 h on 14 mm2 glass coverslips that were placed in culture dishes, various solutions (DOX, pHLIP-SS-DOX, Py-SS-DOX, DOX-SH) at a DOX concentration of 7 μM were added to each dish and incubated for another 3 h at pH 7.4 or pH 6.0. At the end of the incubation, cells were washed with cold PBS, fixed with 4% paraformaldehyde for 30 min and stained with Hoechst 33258 (excitation/emission wavelength: 352/461 nm) for 30 min at 37 °C. Then the fluorescent images of cells were analyzed using a laser scanning confocal microscope (LSCM, LEICA, TCS SP5, Germany).
In vitro cytotoxicity mediated by GSH
The in vitro cytotoxicity mediated by GSH was evaluated by SRB colorimetric assay of MCF-7 cells. The intracellular level of GSH was elevated by pretreatment with GSH ethyl ester (GSH-OEt) (Chuan et al., Citation2014). Briefly, each well on a 96-well plate was plated with 5000 MCF-7 cells and incubated for 24 h. Then cell culture medium containing 10 mM GSH-OEt was added to preincubate for 2 h with cells. Cells without GSH-OEt pretreatment were used as control. Subsequently, the medium containing GSH-OEt was removed and the cells were washed by PBS three times. The cells were then exposed to pHLIP-SS-DOX solutions (at a DOX concentration of 431 and 86 μM) diluted with RPMI 1640 medium for 48 h at 37 °C at pH 7.4 or pH 6.0. The cells were fixed with 200 μL of 10% cold trichloroacetic acid at 4 °C for 1 h, then washed with distilled water and dried in the air. Subsequently, the fixed cells were stained with 100 μL of 0.4% SRB for 30 min and washed with 1% acetic acid five times. Finally, 200 μL of 10 mM Tris base was added into each well to dissolve the cellular bound SRB with shaking for 30 min, and the absorbance at 540 nm was quantified using a 96-well plate reader (Thermo Scientific, Multiskan, FC).
Statistical analysis
Data were expressed as mean ± SD of multiple determinations along with Student’s t-test. The differences were considered significant if p < 0.05.
Results and discussion
Synthesis of pHLIP-SS-DOX
pHLIP-SS-DOX was successfully synthesized through a three-step reaction as shown in Scheme 1. First, Py-SS-MPA was synthesized via a thiol-disulfide exchange reaction. The excess mole of Py-SS-Py not only makes the reaction more completely but also inhibits the produce of by-products MPA-SS-MPA together with the addition of acetic acid. 1HNMR spectrum (400 MHz, DMSO-d6, ) demonstrated the successful synthesis of Py-SS-MPA and the results were in accordance with literature: δ, 2.64 (2H, t), 3.01 (2H, t), 7.26 (1H, dd), 7.77 (1H, d), 7.84 (1H, td), 8.45 (1H, t) (Chen et al., Citation2012). ESI/MS also validated the successful synthesis of Py-SS-MPA, as the experimental MW of Py-SS-MPA was observed to be approximately 214 (Figure S1) and the theoretical calculated MW was 215, which was in accordance with the fact that compound with a carboxyl group always appeared the peak of M-1. The product yield was 44.68%.
Figure 2. Characteristics of Py-SS-DOX. 1H NMR spectra (A) and HPLC spectra (B) of DOX·HCl, Py-SS-COOH, Py-SS-DOX. ESI/MS spectrum (C) and FTIR spectrum (D) of Py-SS-DOX.
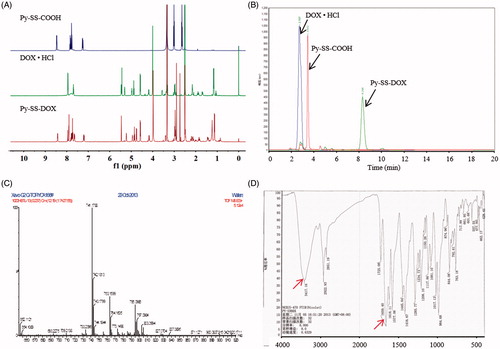
Scheme 1. Synthetic routes. Reagents and conditions: (i) Ethanol/AcOH, rt, 2–3 h; (ii) DMF, HBTU, DIEA, rt, 12 h; (iii) DMF, rt, 48 h.
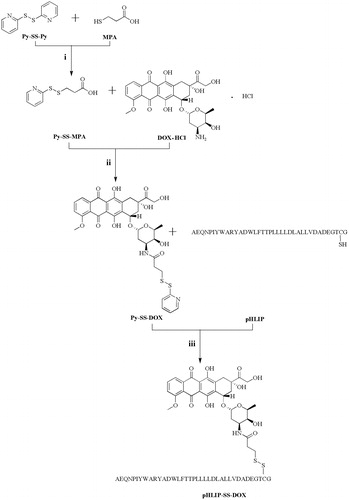
Subsequently, disulfide bond was introduced to DOX via an amide reaction from Py-SS-MPA, resulting in the formation of Py-SS-DOX. It was the couple reaction of the carboxyl group of Py-SS-MPA and the amine group of DOX. We choose to modify the amine group of DOX because the conjugation via the amine group is easier (Santra et al., Citation2011). HBTU is a coupling reagent commonly used as a carboxylic acid activating reagent (Mali et al., Citation2014). It has the following advantages: simple reaction conditions, short reaction time and high yield. 1H NMR spectra (400 MHz, DMSO-d6, ) of Py-SS-MPA, DOX·HCl and Py-SS-DOX showed that the characteristic peaks of starting materials Py-SS-MPA and DOX·HCl could be found in the spectrum of the product Py-SS-DOX, for this result there are two possibilities, successful conjugation or mixture of materials. The HPLC spectra () indicated that there was no peak of Py-SS-MPA or DOX·HCl in the spectrum of the product, reflecting that it was not the mixture of materials. 1H NMR and HPLC together demonstrated that we successfully synthesized Py-SS-DOX. The ESI/MS spectrum () further indicated the successful synthesis of the product Py-SS-DOX, as there was a peak at m/z 741.1099, which was consistant with the theoretical calculated value. Proofs above demonstrated the successful conjugation of Py-SS-MPA and DOX·HCl, but they could not identify whether the carboxyl group of Py-SS-MPA was conjugated to the amine group of DOX·HCl or the hydroxyl group of DOX·HCl. The FTIR spectrum could identify the site of conjugation. As shown in , there were characteristic peaks of amide bond in the FTIR spectrum of the product, indicating the conjugation was via the amine group of DOX, the peak of 3417.16 was the N–H stretching vibration and the peak of 1659.40 was the C=O stretching vibration. The yield of the product was 29.9%.
Next, pHLIP was conjugated to Py-SS-DOX via thiol-disulfide exchange reaction. To ensure complete reaction, excess Py-SS-DOX was used (2:1 molar ratio of Py-SS-DOX/pHLIP). The successful synthesis of pHLIP-SS-DOX was validated by MALDI-TOF MS. The MW of pHLIP found in was 4212.64, which was in accordance with the theoretical calculated MW of 4211. After conjugation with Py-SS-DOX, the MW shifted to 4841.11 (the calculated MW of pHLIP-SS-DOX was 4840). In the HPLC spectrum of pHLIP-SS-DOX, we found that the peaks of pHLIP and Py-SS-DOX disappeared, meanwhile a new peak appeared, which is pHLIP-SS-DOX (). The UV spectra of pHLIP-SS-DOX and DOX·HCl also indicated that DOX was successfully conjugated to pHLIP. Besides, it also showed that the conjugation of pHLIP did not affect the UV property of DOX·HCl (). The yield of the product was 72.7%.
Figure 3. Characteristics of pHLIP-SS-DOX and DOX-SS-DOX. (A) MALDI-TOF spectra of pHLIP, pHLIP-SS-DOX, (B) HPLC spectra of Py-SS-DOX, pHLIP, pHLIP-SS-DOX, (C) UV spectra of DOX, pHLIP-SS-DOX. And (D) ESI/MS spectrum of DOX-SS-DOX.
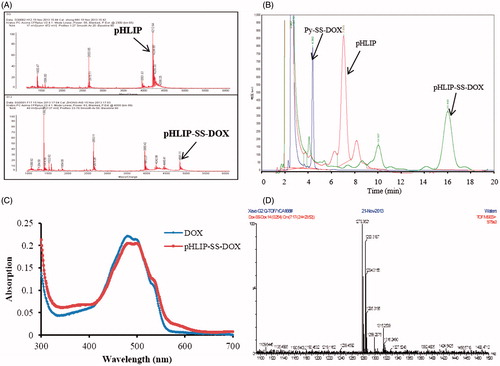
At last, we synthesized DOX-SS-DOX to produce DOX-SH in situ. After cleavage of the disulfide bond, the conjugate pHLIP-SS-DOX released a sulfhydryl-modified DOX (DOX-SH) which displayed different properties with free DOX. So DOX-SH was synthesized as a control. As shown in , the peak of m/z 1278.3617 was M + , the peak of m/z 1283.3190 was M + Na+. In the HPLC spectrum, after the addition of DTT, the new peak of DOX-SH increased with increased time and the peak of DOX-SS-DOX decreased with increased time (Figure S2). The yield of the product was 76.7%.
Verification of the property of pHLIP
To study the three states of pHLIP, we prepared blank liposomes composed of EPC and cholesterol to simulate cellular membranes. Steady-state fluorescence and CD spectroscopy were used to monitor the environmental and conformational changes as pHLIP experienced transitions between its three principal states. We used Trp as reporter group to monitor the insertion of pHLIP into lipid bilayers, because the Trp fluorescence emission was sensitive to the polarity of the environment. Insertion was accompanied by a change of polarity and there were two tryptophan residues in the sequence of pHLIP with at least one of them in the transmembrane region of the peptide. To observe the three states obviously, we chose pH 8.0 and pH 4.0 as neutral and acidic conditions. To observe the state of pHLIP existed under experimental conditions, we also chose pH 6.0 used in subsequent experiments. At pH 8.0, the Trp fluorescence emission maximum of pHLIP was centered around 340 nm (), indicating that Trp residues were exposed to polar, aqueous environments (Burstein et al., Citation1973). Lowering the pH from 8.0 to 4.0 led to significant blue shifts of the maximum and increase in fluorescence intensity. These changes were characteristic for Trp residues buried in hydrophobic environments and suggested that pHLIP was inserted into vesicles at pH 4.0 (Thevenin et al., Citation2009).
Figure 4. Verification of pHLIP's property. Trp fluorescence (A) and CD (B) spectra of pHLIP. Blue line represents fluorescence and CD spectra of pHLIP at pH 8.0 in the absence of liposomes; red, after 30 min of incubation with liposomes at pH 8.0; purple, after incubation with liposomes at pH 4.0. (C) was the interactions between FITC-pHLIP and MCF cells at different conditions for 3 h. Left, pH=7.4; right, pH=6.0.
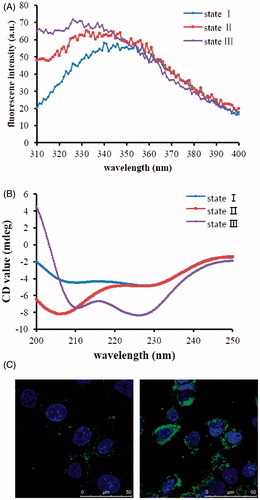
CD spectroscopy can be used to monitor the secondary structure of peptides. When peptides are in unstructured conformation, there is a negative band around 195 nm and low ellipticity above 210 nm. When peptides form α helices, there are two negative bands around 208 and 222 nm and a positive band at 193 nm (Greenfield, Citation2006). As shown in , at pH 8.0, there was a negative band around 206 nm and a low ellipticity above 210 nm indicating that pHLIP adopted an unstructured configuration at pH 8.0, when pH decreased to 4.0, there appeared two negative bands around 210 and 228 nm indicating the formation of α helical structure. Results of the fluorescence and CD spectroscopy were in accordance with the literature, indicating that the peptide indeed had three states. pHLIP was inserted into the lipid bilayers as α helical conformation at low pH, while at neutral pH it did not insert into lipid bilayers with unstructured conformation.
Afterwards, we used laser scanning confocal microscopy to observe the interaction of pHLIP with cell membranes at different pH conditions. Here, considering the conditions in the body, we used pH 7.4 and pH 6.0 to simulate normal tissue environment and tumor tissue environment. After incubation at pH 6.0, pHLIP accumulated mainly around the cell membrane, neither be washed by PBS nor enter the cell, showing that it inserted across the membrane. As contrast, the fluorescence around the cell incubated at pH 7.4 was evidently weaker than that at pH 6.0, indicating pHLIP did not insert into cell membrane at pH 7.4 and stayed outside the cell all the time, and the PBS washing procedure washed them away (). These results rendered us to make the assumption that pHLIP could deliver DOX into cells only under acidic conditions, such as the tumor tissues.
In general, the extracellular pH value of normal cells was 7.4, and the tumor microenvironment was acidic. Based on this fact and the unique insertion behavior of pHLIP, we deduced that it could not insert into cell membranes at the neutral microenvironment of normal cells. So we used pH 7.4 to present the normal tissue and pH 6.0 to present the tumor tissue.
In vitro uptake study of the conjugate
LDH leakage assay
Since the pH used in our experiment was lower than normal pH, in order to eliminate the possibility that the uptake at lower pH was due to the membrane leakage induced by pH itself, we conducted LDH leakage assay to evaluate if the acidity used in our experiment would induce membrane leakage. LDH is a type of stable cytosolic enzyme existed in all cells, and it will release into the cell culture medium immediately once the cell membrane is damaged, so the concentration of LDH in medium is related to the degree of membrane damage. We incubated cells with pHLIP at different pH conditions (pH 7.4, pH 6.0, pH 5.0 and pH 4.0) for 3 h. The incubation time was in accordance with that used in subsequent uptake study. As shown in , there was no LDH leakage at all pH conditions within 3 h, reflecting that the pH conditions used in our experiment did not induce any membrane leakage and the internalized fluorescene intensity in the following uptake study was due to the cellular uptake of the conjugate.
Figure 5. LDH tests of pHLIP-SS-DOX at different pH conditions on MCF-7 cells for 3 h (A) and in vitro flow cytometry analysis of the uptake of DOX, pHLIP-SS-DOX, Py-SS-DOX, DOX-SH on MCF-7 (B) and MCF-7/Adr (C) cells for 3 h. The concentrations of the conjugates are all 7 μM. *p < 0.05 uptake at pH 6.0 versus uptake at pH 7.4, ***p < 0.001 uptake at pH 6.0 versus uptake at pH 7.4.
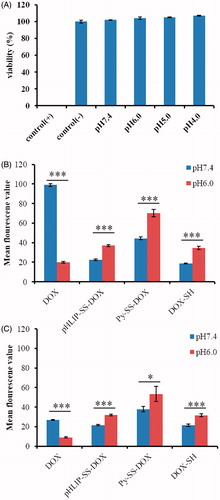
Flow cytometry analysis
Flow cytometry was used to evaluate the uptake capacity of different conjugates quantitatively.
showed the results for MCF-7 cells. For free DOX, the uptake level at pH 7.4 was much higher than that at pH 6.0. This was unfavorable to tumor cells to internalize DOX because the tumor cells had an acidic extracellular microenvironment. We consider this might be resulted from the ionization state change of DOX influenced by pH conditions. At pH 7.4, the amine group of DOX was in free state, as a result, DOX had a relatively lower ionization degree and was easily internalized into cells. While at pH 6.0, the amine group was highly protonized, which caused the higher ionization degree and lower uptake of DOX.
At pH 7.4, the uptake of Py-SS-DOX, DOX-SH and pHLIP-SS-DOX was all lower than that of DOX. However, at pH 6.0, the uptake of Py-SS-DOX, DOX-SH and pHLIP-SS-DOX was all higher than that of DOX. This is a very interesting result. It indicated that these products might increase the cellular uptake in tumor cells where was in low pH condition. We analyzed the general reason for this result might be from: (1) the free amine group was coupled with other groups; (2) the introduction of pyridyl for Py-SS-DOX, sulfydryl for DOX-SH, pHLIP for pHLIP-SS-DOX, all increased the hydrophobicity of DOX (from the prolonged retention time in HPLC spectra); (3) the specific effect of modification structure, such as the CPP function of pHLIP.
As expected, the cellular uptake of pHLIP-SS-DOX at pH 7.4 was lower than that at pH 6.0. At pH 7.4, pHLIP-SS-DOX stayed outside the cells and was easily washed away before the fluorescene measurement. At pH 6.0, because the insertion of pHLIP-SS-DOX into cell membranes, DOX was translocated into cells.
As for Py-SS-DOX and DOX-SH, we also observed the cellular uptake at pH 6.0 was higher than that at pH 7.4. We tried to explain the result from the structure of Py-SS-DOX and DOX-SH, both of them possessed amide bond while DOX was with amine group. The difference in structure might induce the completely opposite pH-responsive activity. For example, the amine group of DOX was in free state at pH 7.4, as a result, DOX had a relatively lower ionization degree and was easily internalized into cells. While at pH 6.0, the amine group was highly protonized, which caused the higher ionization degree and lower uptake of DOX. After modified with nonpolar group, the hydrophobicity was increased, thus increasing the cellular uptake. However, because Py-SS-DOX would release toxic pyridine group and the free sulfydryl group of DOX-SH was not stable in vitro, they did not have druggability.
showed the results for MCF-7/Adr cells. For free DOX, the uptake level at pH 7.4 decreased dramatically because of the efflux mediated by ABC transporters. The uptake level at pH 6.0 also decreased for the same reason and it was still lower than that at pH 7.4 due to the difference in ionization state. For pHLIP-SS-DOX, the cellular uptake levels at pH 7.4 and pH 6.0 were almost the same with that of MCF-7 cells. This result demonstrated that pHLIP-SS-DOX could overcome MDR. For Py-SS-DOX and DOX-SH, the uptake levels at pH 7.4 and pH 6.0 were also similar with that of MCF-7 cells, indicating that Py-SS-DOX and DOX-SH could also overcome MDR. On the basis of these results, we supposed that modification of DOX’s amine group might be significant for overcoming MDR (Ohkawa et al., Citation1993; Minko et al., Citation1998; Guillemard & Uri et al., Citation2004; Liang & Yang et al., Citation2005; Kono et al., Citation2008; Aroui et al., Citation2010; Soudy et al., Citation2013; Szwed et al., Citation2014).
Confocal microscopy studies
Next, we collected laser confocal microscopy images to further confirm the results from flow cytometry analysis. DOX was detected with red fluorescene and the cellular nuclei were stained with Hoechst 33258 with blue fluorescene. depicted the internalization and distribution of DOX in MCF-7 and MCF-7/Adr cells after incubation at pH 7.4 or pH 6.0.
Figure 6. Laser scanning confocal analysis of the uptake of DOX, pHLIP-SS-DOX, Py-SS-DOX, DOX-SH on MCF-7 cells (A, B) and MCF-7/Adr (C, D) cells at pH 7.4 (A, C) or pH 6.0 (B, D) for 3 h. The concentrations the conjugates are all 7 μM.
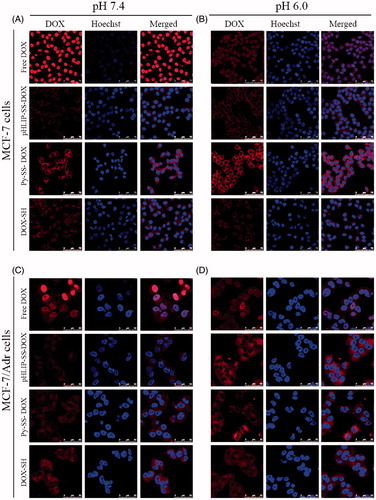
shows the results for MCF-7 cells. For free DOX, the uptake level at pH 7.4 was much higher while the uptake level at pH 6.0 was much low which was in accordance with the cytometry results, and through the co-localization with nucleus, we found that DOX accumulated mostly in the nucleus, where it exerted its cytotoxic effect. For Py-SS-DOX, DOX-SH and pHLIP-SS-DOX, the uptake levels were all in accordance with the cytometry results. But their co-localization with nucleus reflected that after incubated with cells for 3 h, they mainly distributed in cytoplasm, which demonstrated that the modification of DOX slowed down its entry into nuclei. The nucleus of free DOX was relatively dark compared to other images, this was because the binding sites of DOX and Hoechst were both nucleus, and their binding with nucleus was competitive. In the free DOX group, most binding sites were occupied by free DOX because of too much DOX entering nucleus, resulting in the weak fluorescene of Hoechst. For other groups, the uptake of nucleus was low and the occupation of binding sites was low, leaving enough binding sites for Hoechst.
For MCF-7/Adr cells, results are illustrated in . The uptake level of different conjugates was in line with the data obtained from flow cytometry. As for the drug distribution, DOX was accumulated in nucleus while other conjugates were distributed in cytoplasm, which was similar with the results from MCF-7 cells. The uptake level and the DOX distribution both demonstrated that pHLIP-SS-DOX, Py-SS-DOX, DOX-SH could reverse multiple drug resistance.
Both the flow cytometry and confocal microscopy results demonstrated that acidic pH obviously facilitated the cellular uptake of pHLIP-SS-DOX for both drug-sensitive and drug-resistant breast cancer cells and pHLIP-SS-DOX had the ability to reverse MDR. This was attributed to the pHLIP’s specific cell-penetrating function. At low pH, pHLIP was inserted into cells with its C-terminus inside the cell, DOX was released through the cleavage of the disulfide bond. The above results also indicated that acidic environment, such as tumor tissues, was unfavorable to DOX internalization and the modification of DOX’s amine group might be significant for overcoming MDR (Ohkawa et al., Citation1993; Minko et al., Citation1998; Guillemard & Uri et al., Citation2004; Liang & Yang et al., Citation2005; Kono et al., Citation2008; Aroui et al., Citation2010; Soudy et al., Citation2013; Szwed et al., Citation2014).
In vitro cytotoxicity mediated by GSH
The GSH-mediated cytotoxicity was performed to evaluate the effect of reductive environment on tumor inhibition efficacy. GSH with the anionic nature is not transported into cells effectively, but the neutralized GSH-OEt can penetrate cellular membranes and rapidly reach a high intracellular concentration of GSH through hydrolysis in the cytoplasm (Koo et al., Citation2008).
Generally, MCF-7 cells were preincubated with 10 mM GSH-OEt for 2 h to obtain a high intracellular concentration of GSH and then were incubated with the conjugate pHLIP-SS-DOX for another 48 or 72 h at pH 7.4 or pH 6.0. The cell viability of the group treated with cell culture medium was considered as 100%. As shown in , in both groups pretreatment with or without GSH-OEt, after incubation with pHLIP-SS-DOX, the viability of MCF-7 cells significantly decreased at pH 6.0, and the cytotoxicity showed a reductive condition dependent. However, at pH 7.4, no matter in with GSH-OEt pretreatment group or in without GSH-OEt pretreatment group, pHLIP-SS-DOX both showed poor cytotoxicity, especially without reductive condition-dependent character (). The results indicated that at neutral pH 7.4 pHLIP-SS-DOX did not insert into cells and could not make contact with the intracellular environment, while at acidic pH 6.0, pHLIP-SS-DOX was inserted into cells and released more drugs under the more reductive environment. That is to say, the pH-sensitive CPP can deliver drugs into tumor cells with acidic microenvironment and cannot deliver drugs into normal cells with neutral microenvironment. This meant that the delivery system could decrease the side effect of DOX on normal cells. Experimental results at pH 6.0 also showed that with the incubation time prolonged, the cytotoxicity of pHLIP-SS-DOX in the GSH-OEt pretreatment group increased, which reflected that it required time to cleave disulfide bonds to release DOX.
Figure 7. GSH-OEt mediated cytotoxicity against MCF-7 cells after incubation with pHLIP-SS-DOX. Cells were treated with 10 mM GSH-OEt for 2 h before the following treatment with pHLIP-SS-DOX for 48 h or 72 h at pH 7.4 or pH 6.0. **p < 0.01 cell viability without GSH-OEt versus cell viability with GSH-OEt, ***p < 0.001 cell viability without GSH-OEt versus cell viability without GSH-OEt.
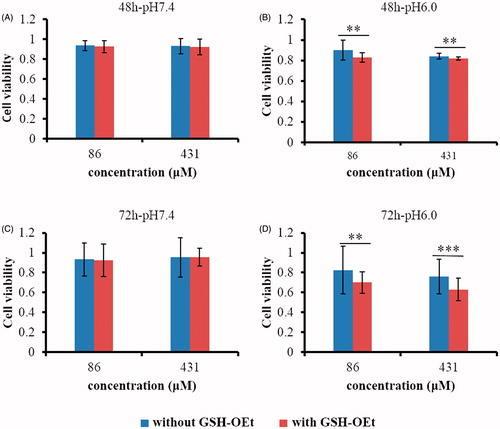
The chemotherapy effect of DOX disappeared after conjugation with pHLIP, because pHLIP-SS-DOX showed no cytotoxicity at pH 7.4, at this condition the conjugate was intact. And the cytotoxicity at pH 6.0 was due to the released DOX. However, the cytotoxicity of pHLIP-SS-DOX (IC50 > 400 μM) was much lower than that of free DOX (IC50 ≈ 0.653 μM). The reason might be the slow breakage of disulfide bonds and the resulting slow release of DOX from pHLIP-SS-DOX. The releasing experiment (data not shown) also showed that the DOX-SH release was low with the present of DTT. Here, the disulfide bond might not be a very sensitive linker to release drugs. So we proposed to use more sensitive linkers to obtain quick drug release, thus improving the drug efficacy. We also proposed to administrate pHLIP-linker-DOX together with free drugs, for example, preparation nanoparticles with pHLIP-linker-DOX to entrap free drugs to achieve the effect of programmed drug release.
Conclusion
We synthesized a pH and reduction dual-responsive targeting DOX conjugate, pHLIP-SS-DOX. It combined several benefits, including pH-sensitive CPPs, reduction-responsive drug release, tumor targeting and reversal of MDR. The pH-sensitive CPP could deliver drugs into tumor cells with acidic microenvironment and could not deliver drugs into normal cells with neutral microenvironment. Fluorescene and CD spectra demonstrated pHLIP inserted into cells as α-helix at acidic conditions. After incubated with tumor cells, it accumulated around the tumor cellular membranes at acidic conditions and did not accumulate around the cell membranes at neutral conditions. Uptake data showed here demonstrated that the conjugate internalized specifically into tumor cells and it was a promising strategy to treat drug resistant cancers. Reduction-responsive drug release was realized by disulfide bond, which stayed stable in circulation and broke in the cytoplasm. But the breakage was a slow process, resulting in the slow cytotoxicity of pHLIP-SS-DOX. In vitro cytotoxicity study showed that GSH-OEt pretreatment would improve the effect of pHLIP-SS-DOX at pH 6.0. In summary, pHLIP-SS-DOX is a promising strategy to target drugs to tumors and provides a possibility to overcome MDR. The antitumor effects in vivo need further investigation.
Supplementary material available online
Supplementary figures S1 and S2.
SUPPLEMENTAL
Download PDF (66.8 KB)Declaration of interest
The authors report no declarations of interest.
This study was supported by the National Natural Science Foundation of China (Nos. 81130059, 81273456 and 81473159).
References
- Ai S, Duan J, Liu X, et al. (2011). Biological evaluation of a novel doxorubicin-peptide conjugate for targeted delivery to EGF receptor-overexpressing tumor cells. Mol Pharm 8:375–86
- Andreev OA, Dupuy AD, Segala M, et al. (2007). Mechanism and uses of a membrane peptide that targets tumors and other acidic tissues in vivo. Proc Natl Acad Sci USA 104:7893–8
- Andreev OA, Karabadzhak AG, Weerakkody D, et al. (2010). pH (low) insertion peptide (pHLIP) inserts across a lipid bilayer as a helix and exits by a different path. Proc Natl Acad Sci USA 107:4081–6
- Aroui S, Brahim S, Waard MD, Kenani A. (2010). Cytotoxicity, intracellular distribution and uptake of doxorubicin and doxorubicin coupled to cell-penetrating peptides in different cell lines: a comparative study. Biochem Biophys Res Commun 391:419–25
- Bao Y, Guo Y, Zhuang X, et al. (2014). D-alpha-tocopherol polyethylene glycol succinate-based redox-sensitive paclitaxel prodrug for overcoming multidrug resistance in cancer cells. Mol Pharm 11:3196–209
- Bechara C, Sagan S. (2013). Cell-penetrating peptides: 20 years later, where do we stand? Febs Lett 587:1693–702
- Burstein EA, Vedenkina NS, Ivkova MN. (1973). Fluorescence and the location of tryptophan residues in protein molecules. Photochem Photobiol 18:263–79
- Chen W, Shi Y, Feng H, et al. (2012). Preparation of copolymer paclitaxel covalently linked via a disulfide bond and its application on controlled drug delivery. J Phys Chem B 116:9231–7
- Chhikara BS, St JN, Mandal D, et al. (2011). Fatty acyl amide derivatives of doxorubicin: synthesis and in vitro anticancer activities. Eur J Med Chem 46:2037–42
- Chuan X, Song Q, Lin J, et al. (2014). Novel free-paclitaxel-loaded redox-responsive nanoparticles based on a disulfide-linked poly(ethylene glycol)-drug conjugate for intracellular drug delivery: synthesis, characterization, and antitumor activity in vitro and in vivo. Mol Pharm 11:3656–70
- Drachuk I, Shchepelina O, Lisunova M, et al. (2012). pH-responsive layer-by-layer nanoshells for direct regulation of cell activity. ACS Nano 6:4266–78
- Dubikovskaya EA, Thorne SH, Pillow TH, et al. (2008). Overcoming multidrug resistance of small-molecule therapeutics through conjugation with releasable octaarginine transporters. Proc Natl Acad Sci USA 105:12128––33
- Fendos J, Engelman D. (2012). pHLIP and acidity as a universal biomarker for cancer. Yale J Biol Med 85:29–35
- Geng Q, Sun X, Gong T, Zhang ZR. (2012). Peptide–drug conjugate linked via a disulfide bond for kidney targeted drug delivery. Bioconjug Chem 23:1200–10
- Girotti AW, Minotti G. (2013). Development of a tumor-specific photoactivatable doxorubicin prodrug. Photochem Photobiol 89:1009–10
- Greenfield NJ. (2006). Using circular dichroism spectra to estimate protein secondary structure. Nat Protoc 1:2876–90
- Guillemard V, Uri SH. (2004). Prodrug chemotherapeutics bypass p-glycoprotein resistance and kill tumors in vivo with high efficacy and target-dependent selectivity. Oncogene 23:3613–21
- He X, Li J, An S, Jiang C. (2013). pH-sensitive drug-delivery systems for tumor targeting. Ther Deliv 4:1499–510
- Hunt JF, Rath P, Rothschild KJ, Engelman DM. (1997). Spontaneous, pH-dependent membrane insertion of a transbilayer alpha-helix. Biochemistry-US 36:15177–92
- KCR Thapa B, Xu P. (2012). pH and redox dual responsive nanoparticle for nuclear targeted drug delivery. Mol Pharm 9:2719–29
- Kono K, Kojima C, Hayashi N, et al. (2008). Preparation and cytotoxic activity of poly(ethylene glycol)-modified poly(amidoamine) dendrimers bearing adriamycin. Biomaterials 29:1664–75
- Koo AN, Lee HJ, Kim SE, et al. (2008). Disulfide-cross-linked PEG-poly(amino acid)s copolymer micelles for glutathione-mediated intracellular drug delivery. Chem Commun (Camb) 48:6570–2
- Kratz F, Beyer U, Roth T, et al. (1998). Transferrin conjugates of doxorubicin: synthesis, characterization, cellular uptake, and in vitro efficacy. J Pharm Sci 87:338–46
- Kuppusamy P, Li H, Ilangovan G, et al. (2002). Noninvasive imaging of tumor redox status and its modification by tissue glutathione levels. Cancer Res 62:307–12
- Liang JF, Yang VC. (2005). Synthesis of doxorubicin-peptide conjugate with multidrug resistant tumor cell killing activity. Bioorg Med Chem Lett 15:5071–5
- Maksimenko A, Dosio F, Mougin J, et al. (2014). A unique squalenoylated and nonpegylated doxorubicin nanomedicine with systemic long-circulating properties and anticancer activity. Proc Natl Acad Sci USA 111:E217–26
- Mali SM, Ganesh KM, Katariya MM, Gopi HN. (2014). HBTU mediated 1-hydroxybenzotriazole (HOBt) conjugate addition: synthesis and stereochemical analysis of beta-benzotriazole N-oxide substituted gamma-amino acids and hybrid peptides. Org Biomol Chem 12:8462–72
- Milletti F. (2012). Cell-penetrating peptides: classes, origin, and current landscape. Drug Discov Today 17:850–60
- Minko T, Kopeckova P, Pozharov V, Kopecek J. (1998). HPMA copolymer bound adriamycin overcomes MDR1 gene encoded resistance in a human ovarian carcinoma cell line. J Control Release 54:223–33
- Mura S, Nicolas J, Couvreur P. (2013). Stimuli-responsive nanocarriers for drug delivery. Nat Mater 12:991–1003
- Nasrollahi SA, Taghibiglou C, Azizi E, Farboud ES. (2012). Cell-penetrating peptides as a novel transdermal drug delivery system. Chem Biol Drug Des 80:639–46
- Ohkawa K, Hatano T, Tsukada Y, Matsuda M. (1993). Chemotherapeutic efficacy of the protein-doxorubicin conjugates on multidrug resistant rat hepatoma cell line in vitro. Br J Cancer 67:274–8
- Pan YJ, Chen YY, Wang DR, et al. (2012). Redox/pH dual stimuli-responsive biodegradable nanohydrogels with varying responses to dithiothreitol and glutathione for controlled drug release. Biomaterials 33:6570–9
- Reshetnyak YK, Andreev OA, Segala M, et al. (2008). Energetics of peptide (pHLIP) binding to and folding across a lipid bilayer membrane. Proc Natl Acad Sci USA 105:15340–5
- Reshetnyak YK, Segala M, Andreev OA, Engelman DM. (2007). A monomeric membrane peptide that lives in three worlds: in solution, attached to, and inserted across lipid bilayers. Biophys J 93:2363–72
- Santra S, Kaittanis C, Santiesteban OJ, Perez JM. (2011). Cell-specific, activatable, and theranostic prodrug for dual-targeted cancer imaging and therapy. J Am Chem Soc 133:16680–8
- Shi NQ, Gao W, Xiang B, Qi XR. (2012). Enhancing cellular uptake of activable cell-penetrating peptide–doxorubicin conjugate by enzymatic cleavage. Int J Nanomed 7:1613–21
- Soudy R, Chen C, Kaur K. (2013). Novel peptide–doxorubucin conjugates for targeting breast cancer cells including the multidrug resistant cells. J Med Chem 56:7564–73
- Szwed M, Kania KD, Jozwiak Z. (2014). Relationship between therapeutic efficacy of doxorubicin–transferrin conjugate and expression of P-glycoprotein in chronic erythromyeloblastoid leukemia cells sensitive and resistant to doxorubicin. Cell Oncol (Dordr) 37:421–8
- Tetin SY, Prendergast FG, Venyaminov SY. (2003). Accuracy of protein secondary structure determination from circular dichroism spectra based on immunoglobulin examples. Anal Biochem 321:183–7
- Thevenin D, An M, Engelman DM. (2009). pHLIP-mediated translocation of membrane-impermeable molecules into cells. Chem Biol 16:754–62
- Vasconcelos L, Parn K, Langel U. (2013). Therapeutic potential of cell-penetrating peptides. Ther Deliv 4:573–91
- Wilson JT, Keller S, Manganiello MJ, et al. (2013). pH-responsive nanoparticle vaccines for dual-delivery of antigens and immunostimulatory oligonucleotides. ACS Nano 7:3912–25
- Wu Q, Yang Z, Nie Y, et al. (2014). Multi-drug resistance in cancer chemotherapeutics: mechanisms and lab approaches. Cancer Lett 347:159–66
- Xie H, Braha O, Gu LQ, et al. (2005). Single-molecule observation of the catalytic subunit of cAMP-dependent protein kinase binding to an inhibitor peptide. Chem Biol 12:109–20
- Yoo HS, Lee KH, Oh JE, Park TG. (2000). In vitro and in vivo anti-tumor activities of nanoparticles based on doxorubicin-PLGA conjugates. J Control Release 68:419–31
- Zhang W, Song J, Zhang B, et al. (2011). Design of acid-activated cell penetrating peptide for delivery of active molecules into cancer cells. Bioconjug Chem 22:1410–15
- Zhao BX, Zhao Y, Huang Y, et al. (2012). The efficiency of tumor-specific pH-responsive peptide-modified polymeric micelles containing paclitaxel. Biomaterials 33:2508–20