Abstract
The effective treatment of brain cancer is hindered by the poor transport across the blood–brain barrier (BBB) and the low penetration across the blood–tumor barrier (BTB). The objective of this work was to formulate transferrin-conjugated docetaxel (DTX)-loaded d-alpha-tocopheryl polyethylene glycol 1000 succinate (vitamin E TPGS or TPGS) micelles for targeted brain cancer therapy. The micelles with and without transferrin conjugation were prepared by the solvent casting method and characterized for their particle size, polydispersity, drug encapsulation efficiency, drug loading, in vitro release study and brain distribution study. Particle sizes of prepared micelles were determined at 25 °C by dynamic light scattering technique. The external surface morphology was determined by transmission electron microscopy analysis and atomic force microscopy. The encapsulation efficiency was determined by spectrophotometery. In vitro release studies of micelles and control formulations were carried out by dialysis bag diffusion method. The particle sizes of the non-targeted and targeted micelles were <20 nm. About 85% of drug encapsulation efficiency was achieved with micelles. The drug release from transferrin-conjugated micelles was sustained for >24 h with 50% of drug release. The in vivo results indicated that transferrin-targeted TPGS micelles could be a promising carrier for brain targeting due to nano-sized drug delivery, solubility enhancement and permeability which provided an improved and prolonged brain targeting of DTX in comparison to the non-targeted micelles and marketed formulation.
Introduction
Brain cancer is one of the most difficult challenges in oncology affecting many people worldwide. According to a report, in the USA, the incidence of primary brain tumors is 7–8 per 100 000, and it is expected that over 23 000 new cases will be diagnosed every year. Among different types of brain cancer, glioma is the most prevalent and aggressive type in adults with a survival time of 12–15 months and a 5-year survival rate of <5% (Cui et al., Citation2013; Burgo et al., Citation2014; Gaillard et al., Citation2014). Despite the development of chemotherapy, median survival span of brain cancer patients (i.e. 1 year) has not been significantly improved. The reduced efficacy of brain cancer chemotherapy is mainly attributed to the existence of blood–brain barrier (BBB), which limits the penetration of drug molecules into brain given systemically (Ren et al., Citation2010). Another obstacle is blood–tumor barrier (BTB), which hampers drug accumulation and uptake, results in poor drug accumulation in brain tumor (Cui et al., Citation2013).
To target, the anti-cancer drug into the brain is one of the most challenging areas of research for drug delivery scientists (Kulkarni & Feng, Citation2011; Wohlfart et al., Citation2012; Muthu et al., Citation2014a,Citationb). Since the efficiency of BBB penetration of anti-cancer drug is low, higher doses are needed to achieve a desired therapeutic effect, which usually produce unwanted side-effects (Alam et al., Citation2010; Gan & Feng, Citation2010). To solve these problems, many drug-targeting and delivery strategies to the brain cancer have been discovered and developed in view of manipulating endogenous transport systems to overcome the BBB without physically interfering with the brain tissues (Béduneaua, Citation2007; Jones & Shusta, Citation2007; Wohlfart et al., Citation2012; Yue et al., Citation2012). Mainly, many nanocarriers are under investigation such as albumin nanoparticles, gelatine nanoparticles, nanoconjugates, polymeric nanoparticles, solid–lipid nanoparticles, carbon nanotubes, micelles and liposomes (Muthu et al., Citation2009; Muthu & Feng, Citation2009, Citation2010; Muthu & Singh, Citation2009; Muthu & Wilson, Citation2010; Muthu et al., Citation2014a).
Among all nanocarriers, micelles have attracted much attention as a targeted drug carrier in recent years. Micelles are amphiphilic spherical nanostructures consisting of a hydrophobic core and a hydrophilic shell. It has many advantages over other drug carriers due to its dynamic structure which provide it much higher thermodynamic stability and kinetic stability. Its hydrophilic block stabilizes the micelle in an aqueous environment for intravenous (i.v.) delivery and the hydrophobic core stores a payload of drug for therapy. They cannot be easily detected and eliminated by reticuloendothelial system due to their small scale dimension (diameter <50 nm) and their hydrophilic shell, thus shows enhanced permeability and retention effect (EPR effect) (Muthu & Singh, Citation2009; Kim et al., Citation2010).
d-alpha-Tocopheryl polyethylene glycol 1000 succinate (TPGS) is as an amphiphilic copolymer used for the preparation of micelles because of its valuable advantages. TPGS is a derivative of the natural vitamin E (alpha-tocopherol), which is conjugated with polyethylene glycol (PEG) 1000 (Zhang et al., Citation2012; Vijayakumar et al., Citation2013; Kutty et al., Citation2015). TPGS is an efficient and safer macromolecule for the preparation of drug- and/or diagnostic material-loaded micelles. TPGS is non-ionic, amphiphilic nature (Mi et al., Citation2011; Kutty & Feng, Citation2013; Zhao et al., Citation2013b; Bao et al., Citation2014). It becomes highly saturated with water molecules which results in enhanced stability of TPGS-coated structures, enhanced cellular uptake, prolonged blood circulation and hence enhanced bioavailability. Its bulky structure and large surface area makes it an excellent emulsifier and solubilizer of hydrophobic drugs (Feng, Citation2006; Zhao & Feng, Citation2010; Muthu et al., Citation2011). For example, Muthu et al. (Citation2012) have prepared docetaxel (DTX)-loaded TPGS micelles, which showed three times increase of cytotoxicity than control DTX in brain cancer cells. Biodistribution study in normal rats shows that micelles of DTX were found to be present for longer time in brain, lungs and blood in comparison to control DTX. In another study, theranostic TPGS micelles containing supraparamagnetic iron oxide were prepared for diagnosis and therapy of cancer. It had improved thermal and magnetic properties, in vitro cellular uptake, higher cytotoxicity and better in vivo imaging effects in comparison with commercial Resovist® and Pluronic® F127 micelles (Chandrasekharan et al., Citation2011).
N-debenzoyl-N-tert-butoxycarbonyl-10-deacetyl-paclitaxel (DTX) is a semi-synthetic derivative of the taxoid family of anti-neoplastic agents. It is an analogue of paclitaxel which is extracted from the needles of the European yew tree (Taxus baccata L.). DTX has been found to be more effective than paclitaxel against breast, ovarian, lung, brain and neck cancers (Bissery et al., Citation1991). Currently, Taxotere® (DTX formulated in polysobate 80) is available in market, which causes side effects despite of higher patient response than Taxol® (paclitaxel formulated in Cremophor EL). Better adjuvant formulation has been under intensive investigation (Liu et al., Citation2010). Another problem of DTX is its poor drug permeability across the BBB for brain cancer chemotherapy (Gan & Feng, Citation2010).
Transferrin (β-1 glycopeptide) is a hydrophilic transport vector, controls the extracellular iron level in the body fluid by binding and sequestering. Transferrin receptor overexpression has been targeted for improving the BBB transport of drugs by numerous researchers (Kuo et al., Citation2011; Li et al., Citation2012). Also, transferrin receptor expression on cancer cells can be up to 100-fold higher than the average expression in normal cells owing to their rapid proliferation rate and iron demand, thereby transferrin has been explored as a targeting ligand for nanocarriers to deliver therapeutic and diagnostic agent into cancer cells (Jones & Shusta, Citation2007; Ulbrich et al., Citation2009).
We aim in this research to develop TPGS micelles for transferrin receptor-mediated active delivery of DTX for brain cancer therapy. DTX used as a model anti-cancer agent and transferrin as a model brain-targeting ligand. Firstly, acid functionalized d-alpha-tocopheryl polyethylene glycol 1000 succinate (TPGS-COOH) was conjugated covalently to the amino ends of transferrin (i.e. TPGS-Tf). Secondly, conjugate (TPGS-Tf) was assembled on the surface of the micelles and a therapeutic agent (i.e. DTX) was encapsulated in the lipophilic core of the micelles by solvent casting (SC) method. The micelles were then characterized for their size and size distribution, surface morphology, drug encapsulation efficiency, drug loading and drug release profile. Further, micelles were tested in vivo for brain distribution and its kinetics after i.v. administration to prove the transport efficiency of DTX across the BBB and its targeted delivery into brain.
Materials and methods
The drug of choice in the present investigation, DTX, was obtained from Neon Laboratories Ltd, Mumbai, India, as gift sample. TPGS was obtained from Antares Health Products, St. Charles, MO, as gift sample. Dialysis membrane (Spectra/Por7®) of 1 kDa molecular weight cut off was purchased from Spectrum Laboratories Inc., Rancho Dominguez, CA. Docel™ was purchased from RPG Life Sciences Limited, Mumbai, India. Dialysis bag with a 12 kDa molecular weight cut off was purchased from Sigma–Aldrich Chemicals, St. Louis, MO. All other chemicals were of analytical grade.
Synthesis of TPGS-COOH (activation of TPGS) and its conjugates
For conjugating transferrin to TPGS, TPGS was first activated (i.e. TPGS-COOH) by succinic anhydride through ring opening polymerisation reaction in the presence of DMAP () (Raju et al., Citation2013; Muthu et al., Citation2015b). In brief, TPGS (0.77 g, 0.5 mM), succinic anhydride (0.10 g, 1 mM) and DMAP (0.12 g, 1 mM) were mixed and heated at 100 °C under nitrogen atmosphere for 24 h. The mixture was cooled to room temperature, dissolved in 5 ml of cold dichloromethane, filtered to remove excessive succinic anhydride and then precipitated in 100 ml of diethyl ether at −10 °C overnight. The white TPGS-COOH precipitate was filtered and dried in vacuum.
Figure 1. Schematic diagram for (A) synthesis of (i) TPGS-COOH, (ii) TPGS-Tf and (B) preparation of (i) non-targeted (DTX-TPGS-150) and (ii) transferrin receptor-targeted micelles (DTX-TPGS-Tf). DTX-TPGS-150, DTX-loaded TPGS micelles; DTX-TPGS-Tf, transferrin-conjugated DTX-loaded TPGS micelles.
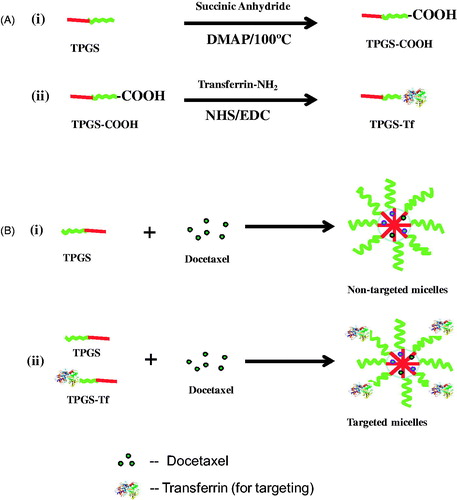
TPGS-COOH carboxyl group was conjugated to the transferrin by carbodiimide chemistry with the help of EDC and NHS in phosphate buffer saline (pH 5.5) () (Muthu et al., Citation2015b). In brief, for conjugation of transferrin to TPGS-COOH, 1-ethyl-3-(3-dimethylaminopropyl) carbodiimide (EDC) and N-hydroxysuccinimide (NHS) (both are catalyst) were added into TPGS-COOH with a molar ratio of 1:5 (TPGS-COOH : EDC or NHS) in phosphate buffer saline (pH 5.5). In brief, TPGS-COOH (200 mg), EDC (96 mg) and NHS (74 mg) were mixed in 2 ml of phosphate buffer saline (pH 5.5) at 25 °C for 5 h, stored in a refrigerator at 4 °C over 24 h, mixed with 1 ml of 0.2% (w/v) transferrin and stirred at 4 °C over 8 h. The resulted product was then dialyzed using a dialyzing membrane (MWCO: 12 kDa) against phosphate buffer saline (pH 5.5) for 48 h in order to remove the excess TPGS-COOH, NHS and EDC. The dialyzed product (i.e. TPGS-Tf) was freeze dried and used for micelle formation.
Characterization of TPGS-COOH and its conjugates
Fourier transform infrared spectroscopy
Fourier transform infrared (FTIR) spectrophotometer (Perkin Elmer Spectrum Two, Waltham, MA) was used to investigate the molecular structure of activated TPGS (TPGS-COOH) and transferrin-conjugated TPGS (TPGS-Tf). The scanning range was 400–4000 cm−1 and the resolution used was 2 cm−1 (Ha et al., Citation2010; Raju et al., Citation2013).
Proton nuclear magnetic resonance (1H NMR) spectrophotometery
The structure of synthesized TPGS-COOH and TPGS-Tf were confirmed by 1H NMR spectrophotometery (JEOL FX Fourier Transform Multinuclear NMR Spectrometer, Japan). For nuclear magnetic resonance (NMR) analysis, TPGS-COOH and TPGS-Tf were solubilized in CDCl3 and D2O, respectively (Zhang et al., Citation2012).
Preparation of TPGS micelles
Solvent casting method
TPGS micelles were prepared according to the SC method (Muthu et al., Citation2012) ( and ). DTX-loaded micellar nanosuspension was prepared with 150 mg of TPGS (DTX-TPGS-150). About 3 mg of DTX and 150 mg of TPGS were dissolved in 3 ml of chloroform. From this mixture, chloroform was evaporated using a Buchi Rotary Evaporator R-210 Advanced, Switzerland at 37 °C and formed a thin film of drug-dispersed TPGS. Drug-loaded micelles were formed by resuspending the drug-loaded film in 0.1 M phosphate buffered saline (PBS), with pH 7.4. The mixture was incubated in orbital water bath shaker at 37 °C for 48 h. The excess non-incorporated drug was separated by filtration (using 0.22 µm filter) before characterization. The targeted formulations were prepared with different concentration of TPGS-Tf to obtain higher drug encapsulation, drug loading and controlled drug release ().
Table 1. Formulas of preparations.
Micelle characterization
Particle size, polydispersity and surface morphology
Particle sizes and polydispersity of DTX-loaded TPGS micelles (both non-targeted and targeted) were measured by photon correlation spectroscopy (PCS) using Zetasizer ver. 7.03, Malvern instruments Ltd, Malvern, UK) which analyzed particle size in the range from 0.6 nm to 6 µm. The measurements were taken without dilution of micelles.
Surface morphology (particle size and the shape) was studied by a transmission electron microscopy (TEM) system (Philips CM-12, Fullerton, CA). Samples of micelles were prepared by placing one drop on a copper grid and dried under vacuum pressure. The dried micelles being stained with 1% phosphotungstic acid for 30 s were finally examined.
Additionally, surface morphology of micelles was visualized by atomic force microscopy (AFM) under normal atmospheric conditions. The Explorer™ atomic force microscope (SOLVER NEXT, NT-MDT Co., Moscow, Russia) was in tapping mode, using high resonant frequency (F0 = 346 kHz) pyramidal cantilevers with silicon probes having force constants of 41 N/m. Scan speeds were set at 1 Hz. The samples were diluted twice with distilled water and then dropped onto glass slides, followed by vacuum drying during 24 h at 25 °C. The measurements were obtained using AFM image analysis software (NOVA-Px-3.1.0-Rev 3880, Moscow, Russia).
Determination of encapsulation efficiency and drug loading of micelles
The encapsulation efficiency of micelle was determined by UV spectrophotometer (Shimadzu 1800, Japan). A 3 ml aliquot of micelles formulation was evaporated to dryness by rotary evaporator under reduced pressure at 35 °C. The residue was dissolved in 20 ml of methanol. Further, 1 ml aliquot of this solution was further diluted to 10 ml with methanol. Then, light absorption was measured at 229 nm using spectrophotometer. The drug encapsulation efficiency was defined as the ratio between the amount of DTX encapsulated in the micelles and that added in the micelles preparation process. The drug loading was calculated as the weight of drug (µg) per 1 mg of the drug-loaded micelles. All samples were done in triplicate.
In vitro drug release studies
The dialysis bag diffusion technique was used to study the in vitro drug release of drug-loaded micelles (Muthu et al., Citation2012). About 2 ml of drug-loaded micellar nanosuspension (equivalent to 200 μg/ml of DTX) were placed in the dialysis bag (cellulose membrane, molecular weight cut off 1000 D), hermetically sealed and immersed into 50 ml of phosphate buffered saline (pH 7.4). The entire system was kept at 37 ± 0.5 °C with continuous shaking at 100 rpm/min. Samples were withdrawn from the receptor compartment at predetermined time intervals and replaced by fresh medium. The amount of drug released was determined by UV spectrophotometer at 229 nm.
In vivo study – brain distribution of micelles in rats
Charles Foster rats (200 ± 20 g) of Institute of Medical Sciences, Banaras Hindu University, India, were used to study the brain distribution of DTX micelles in rats. Before experimentation they were kept in groups of six and fed with standard diet with water ad libitum. All experimental procedures were reviewed and approved by the animal and ethics review committee of Banaras Hindu University, India (Dean/11-12/CAEC/258).
The rats were randomly distributed into nine groups with four rats in each group (n = 4). Groups 1–3 received an i.v. injection (via lateral tail vein) of marketed formulation Docel™ (as control) while Groups 4–6 and Groups 7–9 received i.v. injection of the DTX-loaded non-targeted and brain-targeted micellar formulation, respectively. Totally, 36 rats were used for this study. The Docel™ (control) and drug-loaded non-targeted or targeted micelle formulations were prepared in 0.9% w/v sodium chloride solution (normal saline). The dose and volume of formulations were maintained at 5 ml/kg. The formulations were administered through the lateral tail vein at a DTX dose of 1 mg/kg of body weight.
All animals were regularly monitored for their general health condition, clinical signs, stress, movement and activity or mortality. At different time points after the treatment (0.5, 2 and 4 h), rats were given with anesthesia (urethane – 1.5 g/kg of rat, intraperitonealy) and brains were harvested after decapitation from different treatment groups. The brain samples were stored at −80 °C until analysis. Then, the samples were freeze-dried and homogenized into powder. For brain distribution analysis, liquid–liquid extraction using ethyl acetate was performed to extract the drug from the brain samples. Subsequently, 100 mg of the powder was extracted with 1 ml ethyl acetate. The mixture was vigorously stirred on vortex shaker for 3 min and extracted the drug. After that, the mixture was centrifuged at 11 000 rpm for 15 min. The organic layer was transferred into a new tube for drying. Then, the drug was reconstituted in 200 μl of high-performance liquid chromatography (HPLC) mobile phase (acetonitrile: water 50:50 v/v). Then, 90 μl of solution was transferred into HPLC insert for analysis and 20 µl was injected into the column (Ascentis®C18, 4.6 × 250 mm, 5 µm) under a flow rate 1.0 ml/min. Total run time was 15 min. The DTX content of the brain samples (obtained from control, non-targeted and targeted micelles) was then calculated based on the pre-established calibration curve using blank tissue (brain). The column effluent was detected with a UV-VIS detector at 229 nm (Gan et al., Citation2010; Muthu et al., Citation2012).
Statistical analysis
Statistical analysis was performed by Student’s t-test. All results are given as mean ± SD. Differences are considered statistically significant at a level of p < 0.05.
Results and discussion
Characterization of TPGS, TPGS-COOH and its conjugate by FTIR
shows FTIR spectra of the TPGS, TPGS-COOH and TPGS-Tf. The carbonyl band (–C=O) of TPGS appears at 1736.37 cm−1. Overlapping of the –CH stretching band of TPGS was observed at 2870.45 cm−1. The peaks at 1141.94 and 1250.57 cm−1 were observed for (–C–O–) stretching of TPGS. The peak for –C–C– stretching in aromatic ring was appeared at 1458.37 cm−1. The peaks for –CH2 group of PEG chain was observed at 1377.67 cm−1. For TPGS-COOH, intensity of peak for –C=O stretching vibration in ester group was increased and observed at 1738.47 cm−1 that confirmed the formation of carboxylic group. Overlapping of the –CH stretching band at 2922.98 cm−1 was observed. The peaks for other groups are almost similar to TPGS. In both TPGS and TPGS-COOH, the absorption band at 3422.27 and 3450.04 cm−1 was due to the terminal hydroxyl group (). In transferrin-conjugated TPGS, a characteristic peak at 1634 cm−1 was appeared which corresponds to the bonded amide stretching vibrations, thus confirming the successful conjugation of transferrin to the TPGS-COOH. Peak at 3452 cm−1 was observed for terminal hydroxyl group (). The above results indicate the formation of TPGS-Tf conjugate.
Characterization of TPGS-COOH and TPGS-Tf by 1H NMR spectrophotometer
NMR spectra of TPGS-COOH and TPGS-Tf represented the characteristic peaks which confirmed the synthesis of TPGS-COOH (activated TPGS) and conjugation of Tf to TPGS-COOH. In 1H NMR spectra of TPGS-COOH, the triplet signals at 3.156, 2.932 and 2.910 ppm were observed which belongs to –CH2 protons (poly ethyleneoxide) part of TPGS-COOH, respectively. The signals at 0.853 and 0.875 ppm belong to protons of aliphatic chain. The succinoyl methylene (–CH2) gave signals at 1.968–2.007 ppm which confirm the formation TPGS-COOH. The signal at 5.572 is observed for alkene proton and the signals at 6.636, 7.284 and 8.255 were observed for aromatic protons of TPGS (). The results confirmed the formation of TPGS-COOH. The 1H NMR spectra of TPGS-Tf demonstrated a signal at 4.238 ppm which confirms the conjugation between free amino group of Tf and carboxylic group of TPGS-COOH.
Particle size, polydispersity and surface morphology of micelles
The mean particle size and polydispersity of the DTX-loaded micelles (non-targeted and targeted) are listed in . The particle size and its population SD of the DTX-loaded non-targeted micelles (DTX-TPGS-150) were found to be 15.29 ± 4.02 nm. While the mean sizes of DTX-loaded transferrin-targeted micelles were found 16.79 ± 3.28 nm, 18.28 ± 3.24 and 20.36 ± 3.19 nm for batches DTX-TPGS-Tf1 (contains 50 mg of TPGS-Tf and 100 mg of TPGS), DTX-TPGS-Tf2 (contains 75 mg of TPGS-Tf and 75 mg of TPGS) and DTX-TPGS-Tf3 (contains 100 mg of TPGS-Tf and 75 mg of TPGS), respectively. The particle size results indicate that incorporation of targeting ligand (transferrin) on the micelles surface that leads to an increase in the size in comparison to DTX-loaded non-targeted micelles. However, there was no significant difference in particle size between these batches (p > 0.05). The higher number of Tf molecules available to the surrounding of the micelles leads to the formation of larger micelles ( and ). The polydispersity of all the batches showed quite narrow size distribution, which is nearer to 0.2–0.5. The particle size distribution curves for all the samples were unimodal (). It was reported that micelles in the sizes between 10 and 100 nm are suitable for cancer drug delivery. Micelles in such size enhance EPR effect to tumor by passive targeting (Muthu & Singh, Citation2009; Muthu et al., Citation2012).
Figure 4. Particle size results of non-targeted and targeted DTX-loaded TPGS micelles. DTX-TPGS-150, DTX-loaded TPGS micelles; DTX-TPGS-Tf, transferrin-conjugated DTX-loaded TPGS micelles.
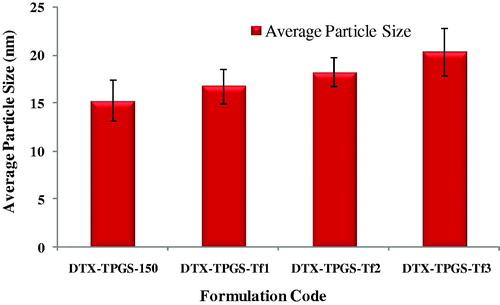
Figure 5. Histogram showing particle size and size distribution of DTX-loaded TPGS micelles (A) DTX-TPGS-150 (non-targeted), (B) DTX-TPGS-Tf1, (C) DTX-TPGS-Tf2 and (D) DTX-TPGS-Tf3. DTX-TPGS-150, DTX-loaded TPGS micelles; DTX-TPGS-Tf, transferrin-conjugated DTX-loaded TPGS micelles; DTX-TPGS-150, DTX-loaded TPGS micelles; DTX-TPGS-Tf, transferrin-conjugated DTX-loaded TPGS micelles.
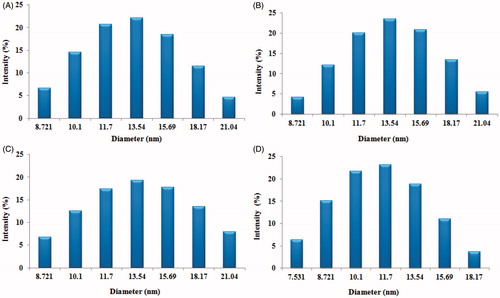
Table 2. Particle size, polydispersity index, encapsulation efficiency and drug loading.
shows TEM of (A) non-targeted DTX-loaded micelles in 100 nm scale, (B) non-targeted DTX-loaded micelles in 50 nm scale and (C) transferrin-targeted DTX-loaded micelles in 50 nm scale, (D) transferrin-targeted DTX-loaded micelles in 100 nm scale which revealed that the DTX-loaded non-targeted and targeted micelles were spherical in shape (). The micelles size as observed by TEM correlated, which also agrees well with that measured by PCS. Additionally, TEM images also confirmed the presence of transferrin coating on the micelles surface ().
Figure 6. Transmission electron microscope (TEM) image of (A) multiple non-targeted micelles (DTX-TPGS-150) in 100 nm scale, (B) multiple non-targeted micelles (DTX-TPGS-150) in 50 nm scale, (C) multiple-targeted TPGS micelles (DTX-TPGS-Tf3) in 100 nm scale and (D) multiple-targeted micelles (DTX-TPGS-Tf3) in 50 nm scale.
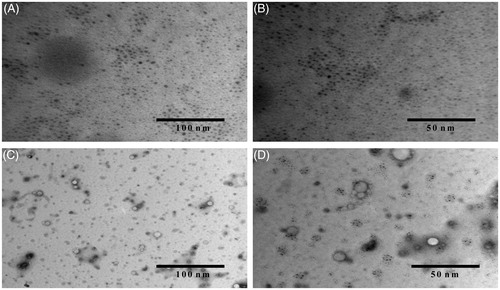
The AFM image (3D image) of micelles is shown in . Image shows the smooth surface without any noticeable pinholes or cracks. AFM also revealed that the DTX-loaded TPGS micelles was spherical in shape of size <30 nm, which agrees quite well with the result measured by PCS and TEM ().
Drug encapsulation efficiency and drug loading of micelles
The drug encapsulation efficiency of the DTX-loaded targeted TPGS micelles were 84.00 ± 0.90%, 80.83 ± 0.65% and 74.58 ± 0.47% for batches DTX-TPGS-Tf1, DTX-TPGS-Tf2 and DTX-TPGS-Tf3, respectively (). The targeted micelles showed significant decrease (p < 0.05) in the encapsulation efficiency with the increase in TPGS-Tf concentrations. This may be because of the influence of TPGS-Tf (conjugate) in the micelle formation that leads to the disturbance of hydrophobic core of micelles, which produced the drug loss in the preparation process. But non-targeted DTX-loaded TPGS micelles (DTX TPGS-150) showed higher encapsulation efficiency in comparison to targeted micelles, which was found 85.77 ± 0.45%. In the non-targeted micelles, higher concentration of TPGS which made strong hydrophobic core of the micelles with DTX resulted in higher encapsulation efficiency (Muthu et al., Citation2015a) ().
Drug loading is defined as the weight of drug (µg) per mg of the drug-loaded micelles. The drug loading of the non-targeted DTX-loaded TPGS micelles (DTX TPGS-150) was 22.81 ± 0.23 µg/mg. The drug loading of the targeted DTX-loaded TPGS micelles were 21.00 ± 0.02, 19.88 ± 0.09 and 18.62 ± 0.45 µg/mg for batches DTX-TPGS-Tf1, DTX-TPGS-Tf2 and DTX-TPGS-Tf3, respectively (). In comparison to non-targeted micelles, targeted micelles showed significant decrease (p < 0.05) in the drug loading. The targeted micelles showed significant decrease (p < 0.05) in the drug loading with the increase in TPGS-Tf concentrations. As mentioned earlier, the influence of TPGS-Tf (conjugate) in the micelle formation that leads to the disturbance of hydrophobic core of micelles, which produced the drug loss in the preparation process ().
In vitro drug release studies
shows the cumulative percentage release of drug from the non-targeted micelles (DTX-TPGS-150) and the different targeted micelles in phosphate buffered saline (pH 7.4). Non-targeted micelles (DTX-TPGS-150) and the targeted micellar formulations showed slow and sustained release for >24 h. However, in first 4 h, drug was released up to 15–40% due to initial burst release of micelles. After 24 h of dialysis in phosphate buffered saline (pH 7.4), the percentage of drug released from the micelles was 70.26% for non-targeted micelles, and 58.66%, 50.24% and 42.11% for targeted formulations, i.e. DTX-TPGS-Tf1, DTX-TPGS-Tf2 and DTX-TPGS-Tf3, respectively (). It was found that coating of transferrin on the surface of micelles leads to much slower release kinetic of drug in comparison to non-targeted micelles. Further, as the concentration of TPGS-Tf was increased, drug release became more sustained. The t50% (time at which 50% of the drug was released in medium) of the formulations were ∼12, 16, 24 and 48 h for the DTX-TPGS-150, DTX–TPGS-Tf1, DTX-TPGS-Tf2 and DTX-TPGS-Tf3, respectively ().
In vivo – brain distribution study in rats
The brain distribution of DTX formulated in the non-targeted (DTX-TPGS-150) and targeted TPGS micelles (DTX-TPGS-Tf3) were investigated in comparison with marketed formulation Docel™ as control preparation after i.v. administration (). Here, DTX-TPGS-Tf3 formulation was selected to explore the targeting effectiveness owing to its sustained release pattern. The key brain distribution pharmacokinetic parameters were analyzed using Phoenix WinNonlin 6.4 software and results are listed in , which include Tmax, the time at which drug present in maximum concentration, Cmax, the peak concentration of DTX and AUC(0–4 h), the total area under the curve which represents the brain DTX concentration. From results, it was found that the mean concentration of DTX in the brain tissue at 0.5, 2 and 4 h after the i.v. administration was significantly higher for the transferrin-targeted TPGS micelles than non-targeted TPGS micelles and Docel™ (p < 0.05). It was observed that the mean concentration of DTX in brain tissue at 0.5, 2 and 4 h after the i.v. administration was in the order of DTX-TPGS-Tf3 > DTX-TPGS-150 > Docel™. It is worthy note that the DTX-loaded non-targeted micelles achieved higher concentration in brain compared with Docel™. This could be due to the effect of enhanced cellular uptake and sustained release manner of DTX from the micelles. Further, the DTX-loaded targeted micelles (DTX-TPGS-Tf3) resulted in much higher concentration in brain tissue compared with non-targeted micelles (DTX-TPGS-150) and Docel™. The drug concentrations in brain for each of three preparations, namely Docel™, DTX-TPGS-150 and DTX-TPGS-Tf3 micelles were 57 ± 3.05, 288 ± 12.74 and 1122 ± 26.53 ng/g, respectively, which imply that drug formulated in the non-targeted and targeted micelles could be 4.05- and 18.68-folds more efficient than Docel™ after 0.5 h of treatment, respectively. The drug concentrations in brain for each of the three preparations were 96 ± 13.00, 504 ± 14.37 and 2313 ± 26.10 ng/g, respectively, which imply that drug formulated in the non-targeted and targeted micelles could be 4.25- and 23.09-folds more efficient than Docel™ after 2 h of treatment, respectively. The drug concentrations in brain for each of the three preparations were 71 ± 12.20, 360 ± 10.53 and 1284 ± 23.31 ng/g, respectively, which imply that drug formulated in the non-targeted and targeted micelles could be 4.07- and 17.08-folds more efficient than Docel™ after 4 h of treatment, respectively (). It is straightforward to understand that targeted micelles showed 2.89 -, 3.58- and 2.56-folds more drug concentration in brain tissue after 0.5, 2 and 4 h of treatment in comparison with non-targeted formulation () which is mainly because of transferrin receptor-mediated endocytosis mechanism (Sawant et al., Citation2014; Muthu et al., Citation2015b). The results show the controlled release and targeting effect of the targeted micelles for extended duration. The Cmax in brain for each of three preparations, namely Docel™, DTX-TPGS-150 and DTX-TPGS-Tf3 micelles were 96 ± 13.00, 504 ± 14.37 and 2313 ± 26.10 ng/g, respectively. Brain distribution kinetics of DTX in brain shows that maximum folds of drug concentrations in brain tissue were achieved at 2 h (Tmax) after the treatment for all the preparations (). Moreover, the AUC(0–4 h) for targeted micelles was 6453.75 ± 72.59 ngh/g which is 3.21-folds of non-targeted micelles (1530 ± 43.60 ngh/g) and 20.80-folds of Docel™ (296 ± 40.00 ngh/g) ().
Figure 9. (A) Brain distribution and (B) brain distribution kinetics of DTX formulated in Docel™, non-targeted (DTX-TPGS-150) and targeted micelles (DTX-TPGS-Tf3) after 0.5, 2 and 4 h of i.v. administration at the dose of 1 mg/kg (n = 4). DTX-TPGS-150, DTX-loaded TPGS micelles; DTX-TPGS-Tf, transferrin-conjugated DTX-loaded TPGS micelles.
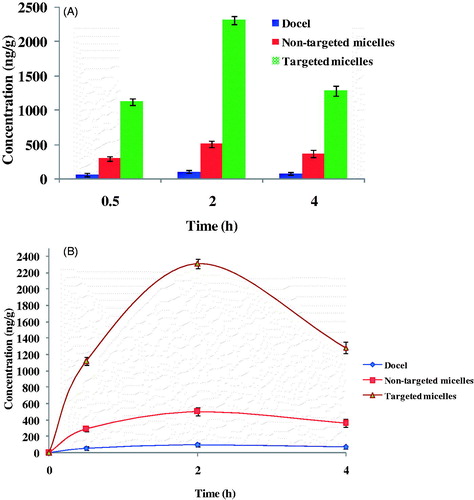
Table 3. Brain distribution kinetics of Docel™, non-targeted micelles (DTX-TPGS-150) and targeted micelles (DTX-TPGS-Tf3) after i.v. injection at an equivalent dose of 1 mg/kg.
The result suggests that the transferrin decoration on micelles could significantly increase the BBB transport of DTX as active targeting (delivery). The higher expression of transferrin receptors on BBB enabled much greater proportion of targeted micelles to be entered into brain via endocytosis mechanism relative to non-targeted micelles or Docel™. Non-targeted micelles also showed significant higher mean concentration of DTX in brain tissue in comparison to Docel™. It was thus demonstrated that the BBB might be overcome by micelles with a size of ∼<50 nm and cause non-selective accumulation of DTX in brain. The TPGS micelles were able to inhibit p-glycoprotein (p-gp)-based drug efflux in BBB that leads to enhancement in brain DTX concentrations (Zhao et al., Citation2013a; Song et al., Citation2014). Further, the control DTX showed the lowest concentration in the brain as a result of the resistance from the BBB as well as the enhanced elimination rate from the body.
Conclusion
In this work, we developed transferrin-conjugated TPGS micelles for targeted delivery of DTX into brain cancer cells. We have synthesized TPGS-COOH from TPGS then it was conjugated with transferrin. The characteristics of TPGS-COOH and conjugate (TPGS-Tf) were analyzed by FTIR spectrophotometer and NMR spectrophotometer. FTIR spectra and NMR spectra have revealed the characteristic peaks associated with the formation TPGS-COOH and TPGS-Tf. Firstly, we have prepared both non-targeted micelles and targeted micelles by SC method. Further, non-targeted and targeted micelles were evaluated for particle size, surface morphology, polydispersity index, drug encapsulation efficiency, drug loading and in vitro study. Particle size and polydispersity of both non-targeted and targeted micelles were found to be satisfactory. The drug encapsulation efficiency was achieved up to 85% for both types of formulations. In vitro release of DTX from the targeted formulation and non-targeted formulations showed slow and sustain release. Here, TPGS has been proven as efficient spacer for targeted nano drug delivery. In addition, we performed in vivo study to confirm the brain-targeting potential of transferrin receptor-targeted TPGS micelles. In comparison with the marketed formulation Docel™ and non-targeting micelles, the transferrin receptor-targeted micelles showed significantly higher drug concentration in brain tissue due to targeting effect of transferrin modified micelles. As maximum non-targeted and targeted micelles could be 4.25- and 23.09 fold more efficient when compared with Docel™ after 2 h treatment, respectively. DTX delivery through transferrin receptor can greatly increase the specificity of drug to brain tumor bearing mice, and thus enhances its therapeutic effects and reduce its side effects. Further, DTX-loaded transferrin receptor-targeted TPGS micelles can be an efficient and cost-effective drug delivery system for clinical application. In future, cellular uptake of these micelles by brain cancer cells and their efficacy study on brain tumor bearing mice will be conducted.
Acknowledgements
M.S. Muthu acknowledges the Science & Engineering Research Board (SERB), New Delhi, India for a research project under Fast track scheme for young scientists (OYS). Project file number is SR/FT/LS-132/2011. We acknowledge Interdisciplinary School of Life Sciences (ISLS), Faculty of Science, Banaras Hindu University, Varanasi, India, for doing HPLC analysis of samples. We also acknowledge Electron Microscopy Facility, Department of Anatomy, Institute of Medical Sciences, Banaras Hindu University, Varanasi, India, in carrying out TEM analysis of samples.
Declaration of interest
The authors report no conflicts of interest. The authors alone are responsible for the content and writing of the article.
References
- Alam MI, Beg S, Samad A, et al. (2010). Strategy for effective brain drug delivery. Eur J Pharm Sci 40:385–403
- Bao Y, Guo Y, Zhuang X, et al. (2014). d-α-Tocopherol polyethylene glycol succinate-based redox sensitive paclitaxel prodrug for overcoming multidrug resistance in cancer cells. Mol Pharm 11:3196–209
- Béduneaua A, Saulnier P, Benoit JP. (2007). Active targeting of brain tumor using nanocarriers. Biomaterials 28:4947–67
- Bissery MC, Guenard D, Gueritte-Voegelein F, et al. (1991). Experimental antitumor activity of taxotere (RP 56976, NSC 628503), a taxol analogue. Cancer Res 51:4845–52
- Burgo LSD, Hernández RM, Orive G, et al. (2014). Nanotherapeutic approaches for brain cancer management. Nanomedicine 10:905–19
- Chandrasekharan P, Maity D, Yong CX, et al. (2011). Vitamin E (D-alpha-tocopheryl-co-poly(ethylene glycol) 1000 succinate) micelles superparamagnetic iron oxide nanoparticles for enhanced thermotherapy and MRI. Biomaterials 32:5663–72
- Cui Y, Xu Q, Chow PK, et al. (2013). Transferrin-conjugated magnetic silica PLGA nanoparticles loaded with doxorubicin and paclitaxel for brain glioma treatment. Biomaterials 34:8511–20
- Feng SS. (2006). New-concept chemotherapy by nanoparticles of biodegradable polymers: where are we now? Nanomedicine (Lond) 1:297–309
- Gaillard PJ, Appeldoorn CCM, Dorland R, et al. (2014). Pharmacokinetics, brain delivery, and efficacy in brain tumor-bearing mice of glutathione pegylated liposomal doxorubicin (2B3-101). PLoS One 9:e82331
- Gan CW, Chien S, Feng SS. (2010). Nanomedicine: enhancement of chemotherapeutic efficacy of docetaxel by using a biodegradable nanoparticle formulation. Curr Pharm Des 16:2308–20
- Gan CW, Feng SS. (2010). Transferrin-conjugated nanoparticles of poly (lactide)-D-α-tocopheryl polyethylene glycol succinate diblock copolymer for targeted drug delivery across the blood brain barrier. Biomaterials 31:7748–57
- Ha PT, Nguyet, Tran TMN, et al. (2010). The synthesis of poly(lactide)-vitamin ETPGS (PLA-TPGS) copolymer and its utilization to formulate a curcumin nanocarriers. Pham Adv Nat Sci: Nanosci Nanotechnol 1:1–7
- Jones AR, Shusta EV. (2007). Blood–brain barrier transport of therapeutics via receptor mediation. Pharm Res 24:1759–71
- Kim S, Shi Y, Kim JY, et al. (2010). Overcoming the barriers in micellar drug delivery: loading efficiency, in vivo stability, and micelle–cell interaction. Expert Opin Drug Deliv 7:49–62
- Kulkarni SA, Feng SS. (2011). Effects of surface modification on delivery efficiency of biodegradable nanoparticles across the blood–brain barrier. Nanomedicine (Lond) 6:377–94
- Kuo YC, Lin PI, Wang CC. (2011). Targeting nevirapine delivery across human brain microvascular endothelial cells using transferrin-grafted poly(lactide-co-glycolide) nanoparticles. Nanomedicine (Lond) 6:1011–26
- Kutty RV, Chia SL, Setyawati MI, et al. (2015). In vivo and ex vivo proofs of concept that cetuximab conjugated vitamin E TPGS micelles increases efficacy of delivered docetaxel against triple negative breast cancer. Biomaterials 63:58–69
- Kutty RV, Feng SS. (2013). Cetuximab conjugated vitamin E TPGS micelles for targeted delivery of docetaxel for treatment of triple negative breast cancers. Biomaterials 34:10160–71
- Li Y, He H, Jia X, et al. (2012). A dual-targeting nanocarrier based on poly (amidoamine) dendrimers conjugated with transferrin and tamoxifen for treating brain gliomas. Biomaterials 33:3899–908
- Liu Y, Li K, Pan J, et al. (2010). Folic acid conjugated nanoparticles of mixed lipid monolayer shell and biodegradable polymer core for targeted delivery of docetaxel. Biomaterials 31:330–8
- Mi Y, Liu Y, Feng SS. (2011). Formulation of docetaxel by folic acid-conjugated d-α-tocopheryl polyethylene glycol succinate 2000 (Vitamin E TPGS (2k)) micelles for targeted and synergistic chemotherapy. Biomaterials 32:4058–66
- Muthu MS, Feng SS. (2009). Pharmaceutical stability aspects of nanomedicines. Nanomedicine (Lond) 4:857–60
- Muthu MS, Feng SS. (2010). Nanopharmacology of liposomes developed for cancer therapy. Nanomedicine (Lond) 5:1017–9
- Muthu MS, Kulkarni SA, Liu Y, Feng SS. (2012). Development of docetaxel-loaded vitamin E TPGS micelles: formulation optimization, effects on brain cancer cells and biodistribution in rats. Nanomedicine (Lond) 7:353–64
- Muthu MS, Kulkarni SA, Xiong J, Feng SS. (2011). Vitamin E TPGS coated liposomes enhanced cellular uptake and cytotoxicity of docetaxel in brain cancer cells. Int J Pharm 421:332–40
- Muthu MS, Kutty RV, Luo Z, et al. (2015b). Theranostic vitamin E TPGS micelles of transferrin conjugation for targeted co-delivery of docetaxel and ultra bright gold nanoclusters. Biomaterials 39:234–48
- Muthu MS, Leong DT, Mei L, Feng SS. (2014a). Nanotheranostics-application and further development of nanomedicine strategies for advanced theranostics. Theranostics 4:660–77
- Muthu MS, Mei L, Feng SS. (2014b). Nanotheranostics: advanced nanomedicine for the integration of diagnosis and therapy. Nanomedicine (Lond) 9:1277–80
- Muthu MS, Sahu AK, Sonali S, et al. (2015a). Solubilized delivery of paliperidone palmitate by D-alpha-tocopheryl polyethylene glycol 1000 succinate micelles for improved short-term psychotic management. Drug Deliv. [Epub ahead of print]. DOI:10.3109/10717544.2014.909907
- Muthu MS, Rajesh CV, Mishra A, Singh S. (2009). Stimulus responsive targeted nanomicelles for effective cancer therapy. Nanomedicine (Lond) 4:657–67
- Muthu MS, Singh S. (2009). Targeted nanomedicines: effective treatment modalities for cancer, AIDS and brain disorders. Nanomedicine (Lond) 4:105–18
- Muthu MS, Wilson B. (2010). Multifunctional radionanomedicine: a novel platform for effective cancer imaging and therapy. Nanomedicine (Lond) 5:169–71
- Raju A, Muthu MS, Feng SS. (2013). Trastuzumab-conjugated vitamin E TPGS liposomes for sustained and targeted delivery of docetaxel. Expert Opin Drug Deliv 10:747–60
- Ren WH, Chang J, Yan CH, et al. (2010). Development of transferrin functionalized poly (ethylene glycol)/poly(lactic acid) amphiphilic block copolymeric micelles as a potential delivery system targeting brain glioma. J Mater Sci Mater Med 21:2673–81
- Sawant RR, Jhaveri AM, Koshkaryev A, et al. (2014). Targeted transferrin-modified polymeric micelles: enhanced efficacy in vitro and in vivo in ovarian carcinoma. Mol Pharm 11:375–81
- Song Q, Tan S, Zhuang X, et al. (2014). Nitric oxide releasing d-α-tocopheryl polyethylene glycol succinate for enhancing antitumor activity of doxorubicin. Mol Pharm 11:4118–29
- Ulbrich K, Hekmatara T, Herbert E, Kreuter J. (2009). Transferrin- and transferrin-receptor-antibody-modified nanoparticles enable drug delivery across the blood–brain barrier (BBB). Eur J Pharm Biopharm 71:251–6
- Vijayakumar MR, Muthu MS, Singh S. (2013). Co-polymers of poly (lactic acid) and D-α-tocopheryl polyethylene glycol 1000 succinate based nanomedicines: versatile multifunctional platforms for cancer diagnosis and therapy. Expert Opin Drug Deliv 10:529–43
- Wohlfart S, Gelperina S, Kreuter J. (2012). Transport of drugs across the blood–brain barrier by nanoparticles. J Control Release 161:264–73
- Yue J, Liu S, Wang R, et al. (2012). Transferrin-conjugated micelles: enhanced accumulation and antitumor effect for transferrin-receptor-overexpressing cancer models. Mol Pharm 9:1919–31
- Zhang Z, Tan S, Feng SS. (2012). Vitamin E TPGS as a molecular biomaterial for drug delivery. Biomaterials 33:4889–906
- Zhao J, Mi Y, Feng SS. (2013a). Targeted co-delivery of docetaxel and siPlk1 by herceptin-conjugated vitamin E TPGS based immunomicelles. Biomaterials 34:3411–21
- Zhao L, Feng SS. (2010). Enhanced oral bioavailability of paclitaxel formulated in vitamin E-TPGS emulsified nanoparticles of biodegradable polymers: in vitro and in vivo studies. J Pharm Sci 99:3552–60
- Zhao S, Tan S, Guo Y, et al. (2013b). pH-Sensitive docetaxel-loaded d-α-tocopheryl polyethylene glycol succinate-poly(β-amino ester) copolymer nanoparticles for overcoming multidrug resistance. Biomacromolecules 14:2636–46