Abstract
In order to enhance oral bioavailability and liver targeting delivery of silybin, two amphiphilic hyaluronic acid derivatives, hyaluronic acid-deoxycholic acid (HA-adh-DOCA) and hyaluronic acid-glycyrrhetinic acid (HA-adh-GA) conjugates, were designed and synthesized. Silybin was successfully loaded in HA-adh-DOCA and HA-adh-GA micelles with high drug-loading capacities (20.3% ± 0.5% and 20.6% ± 0.6%, respectively). The silybin-loaded micelles were spherical in shape with the average size around 130 nm. In vitro release study showed that two silybin-loaded micelles displayed similar steady continued-release pattern in simulated gastrointestinal fluids and PBS. Single-pass intestinal perfusion studies indicated that silybin-loaded micelles were absorbed in the whole intestine and transported via a passive diffusion mechanism. Compared with suspension formulation, silybin-loaded HA-adh-DOCA and HA-adh-GA micelles achieved significantly higher AUC and Cmax level. Moreover, liver targeting drug delivery of micelles was confirmed by in vivo imaging analysis. In comparison between the two micellar formulations, HA-adh-GA micelles possessed higher targeting capacity than HA-adh-DOCA micelles, owing to the active hepatic targeting properties of glycyrrhetinic acid. In the treatment of acute liver injury induced by CCl4, silybin-loaded HA-adh-GA micelles displayed better effects over suspension control and silybin-loaded HA-adh-DOCA micelles. Overall, pharmaceutical and pharmacological indicators suggested that the HA-adh-GA conjugates can be successfully utilized for liver targeting of orally administered therapeutics.
Introduction
Liver diseases, such as acute and chronic hepatitis, liver cirrhosis and hepatocellular carcinoma, remain to be serious health problems worldwide (Rohilla et al., Citation2015). As a natural remedy to treat hepatic disorders, silymarin is a standardized extract of flowers and leaves of Silybum marianum (St. Mary’s thistle, milk thistle) (Rainone, Citation2005; Abenavoli et al., Citation2010). The chemical structures and biologically active principles of silymarin were elucidated in the 1960s. In treatment of liver diseases, silybin is the main active ingredient of silymarin, which acts by antioxidative, antifibrotic, antiinflammatory, membrane stabilizing, immunomodulating and liver regenerating mechanisms (Kvasnicka et al., Citation2003; Trappoliere et al., Citation2009; Abenavoli et al., Citation2010). Recently, the cancer preventive activity of silybin has been reported in many literatures (Ramasamy & Agarwal, Citation2008; Zhang et al., Citation2013b; Liang et al., Citation2014). Despite the promising therapeutic potential, the application of silybin is limited by low oral bioavailability, owing to its low solubility in water, poor absorption from gastrointestinal tract and degradation in gastrointestinal fluids (El-Samaligy et al., Citation2006; Wang et al., Citation2014). In order to overcome these biopharmaceutical challenges, researches have focused on the development of new oral or parenteral drug delivery systems (DDS) of silybin to improve the solubility and bioavailability (Wang et al., Citation2012; Cao et al., Citation2013; Angelico et al., Citation2014). However, one of the crucial drawbacks of the conventional DDS arises from the insufficient delivery of therapeutics to the liver. Because oral administration is still the most convenient and preferred route of drug delivery, we utilize two polymeric micellar delivery systems of silybin to enhance the oral absorption and more importantly, the liver targeting capacity in the present work.
Currently, polymeric micelles have emerged as one of the most documented approaches to deliver drugs from a preclinical and clinical standpoint. For example, the micellar formulations, NK911 and Genexol-PM, have already advanced to the clinical trials (Matsumura, Citation2006; Ahn et al., Citation2014). Thanks to their distinctive core-shell structure, polymeric micelles possess high loading capacity of poorly water-soluble drugs within the hydrophobic inner core; whereas the hydrophilic corona confers aqueous solubility and steric stability, which also protects the encapsulated drug from inactivation in gastrointestinal components (Croy & Kwon, Citation2006; Liang et al., Citation2015). Importantly, due to the small size of micelles, previous reports described that the drug-loaded nanoparticles could be absorbed in intact form by enterocytes or M cells via endocytic pathway after oral administration (Mathot et al., Citation2007; Li et al., Citation2010; Al-Hilal et al., Citation2013). Thereafter, the micellar delivery system is possible to improve pharmacokinetic and biodistribution profiles, such as longer blood circulation and higher targeting activity by passive or active mechanisms (Lian et al., Citation2013; Li et al., Citation2015; Rezazadeh et al., Citation2015).
A number of natural or synthetic polymers have been used to form polymeric micelles. Among them, hyaluronic acid (HA), a non-toxic, biocompatible and biodegradable polysaccharide, has gained intense attention due to its potential as drug delivery carrier (Almeida et al., Citation2014; Laffleur et al., Citation2014; Liu et al., Citation2015). Many studies have reported that HA receptors in human body played important biological roles, such as endocytosis, degradation and signal transduction (Oh et al., Citation2010). Hyaluronic acid receptor for endocytosis (HARE) in liver sinusoidal endothelial cells is one of the specific receptors of HA, which triggers the signal transduction for liver cell trafficking of HA (Takei et al., Citation2004; Ohya et al., Citation2011). On these grounds, HA polymer is an attractive biomaterial to be applied as a targeting moiety in the development of liver-targeting drug delivery systems. However, free HA polymer could not encapsulate hydrophobic drugs due to its high hydrophilicity. To form HA-based polymeric micelles from amphiphilic derivatives, the strategies of chemical modification, mainly through hydroxyl and carboxylic groups in HA, have been adopted to introduce hydrophobic moieties to HA backbone (Oh et al., Citation2010).
Our previous study has reported a novel amphiphilic hyaluronic acid-deoxycholic acid (HA-adh-DOCA) conjugate, taking deoxycholic acid as hydrophobic moieties (Li et al., Citation2012). The micelle formed by HA-adh-DOCA conjugate has great capability in solubilization of water-insoluble drug, such as paclitaxel with the high drug-loading capacities of 33.0 ± 0.5%. In this study, we investigated the potential of HA-adh-DOCA micelles as carriers for orally administrated silybin. In order to evaluate the influence of different hydrophobic moieties on drug delivery, a similar amphiphilic HA-adh-GA conjugate with glycyrrhetinic acid (GA) as hydrophobic moieties was developed. Several studies reported that abundant receptors for GA existed on the cellular membrane of liver parenchymal cell and GA molecule could be used as targeting ligand for hepatocyte targeting (Zhang et al., Citation2012; Lin et al., Citation2014). Accordingly, using GA molecule as hydrophobic moiety and targeting ligand, the HA-adh-GA conjugate might further improve silybin distribution in liver tissue and therapeutic efficacy.
In the present work, HA-adh-DOCA conjugate and HA-adh-GA conjugate were used to encapsulate silybin by self-assembling into micelles to improve the in vitro and in vivo properties of drug. The synthesis of conjugates, drug-loading capacity for silybin, particle size distribution, stability, in vitro drug release profile, absorption mechanism, pharmacokinetics and liver targeting activity were investigated. Furthermore, the therapeutic efficacy of silybin-loaded micelles in terms of in vivo hepato-protective effect was assessed in CCl4-induced acute liver injury mice model.
Materials and methods
Materials
Sodium hyaluronic acid (HA, molecular weights 11 kDa) was purchased from Freda Biochem Co., Ltd. (Shandong, China). Silybin (purity >98.0%, HPLC), 1-ethyl-3 (3-dimethylaminopropyl) carbodiimide (EDC), N-hydroxysuccinimide (NHS), deoxycholic acid (DOCA) and adipic dihydrazide (adh) were purchased from TCI Development Co., Ltd. (Shanghai, China). Glycyrrhetinic acid (GA) was purchased from Sigma Chemical Co. (St. Louis, MO). Formamide and N,N-dimethyl formamide (DMF) were purchased from Sinopharm Chemical Reagent Co., Ltd. (Shanghai, China). The near-infrared dye DiR was obtained from Beijing Fanbo Science and Technology Co., Ltd. (Beijing, China). Methanol and methyl tert-butyl ether (America Tedia Co., Ltd., Fairfield, OH) were of HPLC grade. Water used in the experiment was doubly distilled and deionized. Ethanol and other chemicals were of analytical reagent grade.
Synthesis of amphiphilic HA-adh-DOCA conjugates and HA-adh-GA conjugates
Firstly, carboxylic acid groups of HA were modified with adipic dihydrazide (adh) by use of the EDC reaction, resulting in the formation of HA-adh conjugates, as described by our and other groups (Luo & Prestwich, Citation1999; Li et al., Citation2012). Secondly, amphiphilic HA-adh-DOCA conjugates and HA-adh-GA conjugates were synthesized by coupling HA-adh conjugate with deoxycholic acid (DOCA) and glycyrrhetinic acid (GA), respectively, via the formation of amide linkage (Li et al., Citation2012; Zhang et al., Citation2013a). In brief, carboxylic acid group of DOCA and GA was reactivated by equimolar amounts of EDC and NHS in DMF at 0 °C for 4 h and then at 20 °C overnight to form NHS ester of DOCA and GA, respectively. Then, HA-adh conjugates (0.1 g) were dissolved in formamide (10 mL) by gentle heating. Different amounts of NHS ester of DOCA and GA dissolved in DMF (20 mL) were mixed with HA-adh solutions at room temperature. The reaction continuously proceeded for 24 h with gentle agitation. The resulting solution was dialyzed against the excess amount of water/ethanol (1:1, v/v) for 1 day and then water for 2 days. After being freeze-dried, the final products were obtained as white floccule and stored at 4 °C until further use.
The structure of HA-adh-DOCA conjugate and HA-adh-GA conjugate was analyzed using 1H NMR spectra (AVACE AV-500 spectrometer, Bruker, Germany). HA was dissolved in D2O. DOCA and GA were dissolved in CD3OD. HA-adh-DOCA and HA-adh-GA conjugates were dissolved in the mixed solution of D2O:CD3OD (1:1, v/v). The degree of substitution (DS), defined as the number of DOCA and GA molecules per 100 sugar residues of HA was estimated by 1H NMR and UV measurements (λ = 250 nm), respectively.
Preparation and characterization of silybin-loaded micelles
In order to produce the silybin-loaded micelles, 18 mg of HA-adh-DOCA conjugates or HA-adh-GA conjugates were dissolved in 3 mL deionized (DI) water under stirring at room temperature. The silybin/ethanol solution (10 mg/mL, 0.5 mL) was added into the conjugate solution drop by drop and the mixture was ultrasonicated with a probe-type ultrasonicator (Scentz-950E, Ningbo Scentz Biotechnology Co., Ltd., Hangzhou, China) at 100 W for 30 min in an ice bath. The solution was then dialyzed against an excess amount of DI water with a dialysis bag (MWCO 3500) for 12 h at room temperature. To remove unloaded silybin, the micellar solution was centrifuged at 3000 rpm for 10 min, followed by filtering through a 0.45 μm pore-sized microporous membrane. After lyophilizing, powder was kept in a refrigerator at 4 °C until further use.
In vitro characterization of silybin-loaded micelles
Evaluation of entrapment efficiency and drug-loading capacity
Silybin-loaded micellar solution was diluted with 100 times volume of methanol to disrupt the micellar structure. The amount of encapsulated silybin was then measured using HPLC (Shimadzu LC-20AT System, Kyoto, Japan) with a Diamonsil® C18 column (250 × 4.6 mm, 5 μm particle size) at 288 nm. The mobile phase consisted of a mixture of methanol and 0.025 M KH2PO4 (50:50, v/v) and the pH was adjusted to 3.0 with phosphoric acid. The column temperature was maintained at 40 °C and the mobile phase was pumped at a flow rate of 1.0 mL/min. The injection volume for analysis was 20 μL. Since silybin is an isomeric compound, the sum of the area of the two peaks was calculated for analysis. The entrapment efficiency (EE) and drug-loading (DL) were calculated by the following equations:
Evaluation of particle size and zeta potential
The mean particle size and zeta potential value were determined by dynamic light scattering (DLS) and laser Doppler micro-electrophoresis, respectively, using a Zetasizer Nano ZS90 (Malvern instruments Ltd., Worcestershire, UK). DLS measurements were performed at a scattering angle of 90 degrees. The concentration of silybin-loaded micelles was kept constant at 1 mg/mL.
Transmission electron microscopy examination
The morphology and size distribution of silybin-loaded micelles were observed by transmission electron microscopy (TEM, JEOL-2000, Japan Electron Optics Laboratory Co., Ltd, Tokyo, Japan). Negative staining of samples was performed. One drop of sample solution (1 mg/mL) was placed onto a copper grid coated with carbon, followed by air-dried for 5 min and negatively stained with 0.03% phosphotungstic acid for 30 s. The samples were air-dried at room temperature overnight before observation.
Differential scanning calorimetry measurement
The interaction of HA-based conjugate with silybin was evaluated by differential scanning calorimetry (DSC) analysis using a 204 F1 Phoenix® differential scanning calorimeter (NETZSCH Scientific Instruments Trading (Shanghai) Ltd., Shanghai, China). Four samples including silybin, blank HA-adh-DOCA micelles, the physical mixture of silybin and blank HA-adh-DOCA micelles, silybin-loaded HA-adh-DOCA micelles were sealed on the aluminum pans. For measurement, the temperature range was set at 40–380 °C with a heating rate of 10 °C/min. HA-adh-GA micelles were tested as the same protocol by using HA-adh-GA conjugates.
Stability studies in simulated gastrointestinal fluids
The stability of silybin-loaded HA-adh-DOCA10 (the DS of DOCA was 10%) micelles and HA-adh-GA20 (the DS of GA was 20%) micelles were evaluated under various pH and enzymatic conditions, including simulated gastric fluid (SGF, pH 1.2; 0.5 N HCl, 2.5 g SDS, 2 g NaCl, added DI water to 1 L) with 1% pepsin, simulated intestinal fluid (SIF, pH 6.8; 3 mM sodium taurocholate, 0.75 mM lecithin, 3 g K2HPO4, 7.7 g KCl, 0.5 M NaOH, added DI water to 1 L) with 1% pancreatin and phosphate-buffered saline (PBS, pH 7.4, 0.1 M). Lyophilized silybin-loaded micelles were suspended in 5% glucose solution and placed in a dialysis tube (MWCO 3500). The samples were investigated in SGF for 2 h, and SIF and PBS for 6 h. To explore the changes when micelles were transferred from SGF to SIF, the samples were firstly incubated in SGF at 37 °C. After 2 h, the dialysis tube was transferred into SIF. The micelles were then evaluated for particle size, polydispersity index and zeta potential.
In vitro drug release profile
In vitro silybin release tests were investigated using a dialysis method. Lyophilized silybin-loaded micelles containing 0.5 mg silybin were suspended in 1 mL of 5% glucose solution and placed in a dialysis tube (MWCO 3500). The tube was incubated in 150 mL release media at 37 °C and gently shaken at a speed of 100 rpm. After 2 h incubation of silybin-loaded micelles in the SGF, the bags were transferred to the SIF up to 48 h. The release of the silybin-loaded micelles in PBS was investigated as above for 48 h. The formulation of release media, SGF, SIF and PBS, was the same as described in the Stability Study Section. At definite time intervals, 5 mL medium was taken out and the whole medium was refreshed. The concentration of silybin in the samples was calculated using HPLC, according to the method described above.
Animal
Sprague-Dawley rats (200–240 g) and ICR mice (18–22 g) were obtained from Vital River Laboratory Animal Technology Co., Ltd. (Beijing, China). All animals were pathogen free and allowed free to access food and water. All animal procedures were performed according to animal care protocols approved by the Ethics Committee of Capital Medical University in accordance with the National Institute of Health Guide for the Care and Use of Laboratory Animals.
In situ single-pass intestinal perfusion studies
Stability and binding studies
The stability of silybin-loaded HA-adh-DOCA10 and HA-adh-GA20 micelles was tested by incubation in the blank perfusate at 37 °C. The blank perfusate was obtained by flushing Krebs–Ringer’s buffer solution through intestine in situ at a flow rate of 0.2 mL/min. For the binding study, rat intestinal segments of 10 cm were clipped, everted carefully and put into drug solution at 37 °C. Samples were taken at 2 h post-incubation and immediately used for evaluation. The concentration of silybin used in the test was 100 μg/mL.
SPIP
The surgical procedure and in situ single-pass intestinal perfusion (SPIP) experiments were carried out using the established methods adapted from the literature (Li et al., Citation2010). In general, Sprague-Dawley rats were fasted 12 h before the experiment but had free access to water. Prior to the experiment, the rats were anesthetized with 20% urethane (1.2 g/kg body weight, i.p.) and then fixed on an operation table. Upon verification of loss of pain reflex, a minimal cut was made in the abdominal region. Aimed intestinal segments (duodenum, jejunum, ileum and colon) of 8–10 cm were isolated and cannulated with perfusion tubing, which were connected to a constant flow pump (BT100-1 L, Baoding Longer Precision Pump Co., Ltd., Hebei, China). The incision area was covered with a pled presoaked by isotonic saline solution to decrease moisture loss. Using constant flow pump, the intestinal segment was first washed with 37 °C isotonic saline solution, and then Krebs–Ringer’s buffer solution at a flow rate of 0.2 mL/min for 15 min to attain balance. The drug perfusion solution containing 100 μg/mL silybin and 24 μg/mL phenol red was then infused at a flow rate of 0.2 mL/min. After 30 min of saturation, the perfusate was collected every 15 min for a 90-min perfusion period. At the end of experiments, the length and inner diameter of intestinal segment was measured. The influence of secretion or absorption of water on silybin content was determined with phenol red method.
To explore the effect of drug concentrations on intestinal absorption of silybin-loaded micelles, ileum segment was selected and perfused with different drug solution containing 50, 100, 200 or 300 μg/mL silybin along with 24 μg/mL phenol red.
Sample analysis
Samples (0.5 mL) were diluted by mobile phase (4.5 mL) and centrifuged at 10 000 rpm for 10 min. Then, the concentration of silybin in the supernatant was measured by HPLC method as described above. On the other hand, the concentration of phenol red was determined using UV spectrophotometer (UV-2450, Shimadzu, Kyoto, Japan) at 558 nm, after 0.5 mL sample mixed with 4.5 mL NaOH (0.1 M).
Permeability calculations
The effective permeability, Peff, was calculated using the following equation:
where Q is the perfusion rate (mL/min), r is the radius of the intestine (cm), L is the length of the perfused intestinal segment (cm), Cin and Cout are the silybin concentration in the solution entering and exiting the intestine, respectively, PRin and PRout are the phenol red concentration in the solution entering and exiting the intestine, respectively.
In vivo pharmacokinetic studies in rat
Study design
Eighteen Sprague-Dawley rats were divided randomly into three groups. Group I received silybin suspension, group II received silybin-loaded HA-adh-DOCA10 micelles and group III received silybin-loaded HA-adh-GA20 micelles. The suspension was prepared by dispersing silybin in a solution of 0.5% (w/v) Tween 80 and 0.3% (w/v) hydroxy-propyl methyl cellulose (HPMC), followed by sonication for 30 min at 200 W. All the animal groups received an oral dose equivalent to 30 mg of silybin per kg of body weight. At predetermined times (0.08, 0.17, 0.33, 0.5, 1, 2, 4, 6, 8 and 12 h) after the oral administration, blood samples (0.5 mL) were taken immediately from postorbital venous plexus and placed into pre-heparinized tubes. The plasma harvested by centrifugation (4000 rpm, 10 min) was stored at −20 °C until HPLC analysis.
Sample preparation
Liquid–liquid plasma extraction procedure was carried out as follows: 150 μL of plasma sample was added to a 10-mL centrifuge tube, followed by adding 50 μL of an internal standard solution (α-naphthol, 5 μg/mL in methanol) and 200 μL of 0.1 M Na2HPO4. After vortex mixing for 1 min, 4 mL of methyl tert-butyl ether was added and vortexed for 5 min. The mixture was then centrifuged at 3000 rpm for 10 min, after which 3 mL of the organic layer was transferred to a clean centrifuge tube and evaporated under a gentle stream of nitrogen at 40 °C. The dried residues were resuspended in 100 μL of mobile phase, and 20 μL aliquot were injected into the HPLC system to determine the drug concentration.
HPLC analysis
The same chromatographic conditions were used to determine the silybin concentrations except that the mobile phase was a mixture of methanol and 0.025 M KH2PO4 (45:55, v/v) with the final pH adjusted to 3.0 by adding phosphoric acid.
A plot of plasma silybin concentration versus time was constructed. Non-compartmental pharmacokinetic parameters for extravascular input, that are AUC, Cmax, Tmax and MRT, were computed by Kinetica software (Thermo Fisher Scientific Inc., Waltham, MA, version 4.4.1).
In vivo imaging analysis
To monitor the in vivo biodistribution of micelles, HA-adh-DOCA10 and HA-adh-GA20 micelles were labeled with the near-infrared fluorescence (NIRF) dye, DiR, according to the method for silybin-loaded micelles. ICR mice were given an oral dose of DiR-loaded HA-adh-DOCA10 micelles and HA-adh-GA20 micelles (5 mg/kg). NIRF imaging experiments were performed at pre-determined times (1 h, 6 h, 12 h), using a Kodak in vivo imaging system FX PRO (Kodak, Rochester, NY). The excitation and emission wavelengths were set at 730 and 790 nm, respectively. After living imaging, the mice were sacrificed. Ex vivo fluorescence images of mice tissues were recorded using the same system. The images were analyzed using Kodak Molecular Imaging Software 5.X.
Efficacy of silybin-loaded micelles against acute liver injury
Model construction of acute liver injury in mice
In this experiment, 50 healthy ICR mice were randomly divided into five groups and treated daily for consecutive 7 days as follows: (i) normal control group and CCl4-treated model group were orally administrated with saline; (ii) positive control group was orally administrated with silybin suspension at 30 mg/kg body weight; (iii) two micellar formulation-treated groups were received silybin-loaded HA-adh-DOCA10 and HA-adh-GA20 micelles, respectively, with the same oral dosage (30 mg/kg). After 2 h of the last administration, mice were treated with 0.1% CCl4 (v/v, dissolved in olive oil, 10 mL/kg) via intraperitoneal administration except the normal control group. 16 h after the administration of CCl4, blood samples were collected, followed by centrifugation (4000 rpm, 10 min) at 4 °C to yield serum. The serum was stored at −20 °C until analysis. The liver tissues were excised and washed immediately with ice-cold saline to remove as much blood as possible.
Biochemical parameters of hepatic function
Serum aspartate aminotransferase (AST) and alanine aminotransferase (ALT) levels were measured using standard assay kits (Nanjing Jiancheng Institute of Biotechnology, Nanjing, China) according to the manufacturer’s instructions.
Liver histology
Liver samples were weighed and fixed with 10% neutral buffered formalin. The specimens were embedded in paraffin and cut into 4 μm sections. Then, the thin tissue sections were stained in the usual manner with hematoxylin and eosin (H & E) for histological observation (Eclipse 80i, Nikon Instrument, Tokyo, Japan).
Statistical analysis
All the results are presented as mean ± standard deviation (S.D.). The data were analyzed by Student’s t-test for two groups and one-way ANOVA for multiple groups. A value of p less than 0.05 was considered to be significant.
Results and discussion
Synthesis of HA-adh-DOCA conjugates and HA-adh-GA conjugates
The HA-adh-DOCA conjugates and HA-adh-GA conjugates were synthesized by coupling HA with adipic dihydrazide and then DOCA and GA, respectively (). The structure of HA-adh-DOCA conjugates and HA-adh-GA conjugates were characterized by 1H NMR. As shown in , the characteristic peaks of HA appeared at 2.0 ppm and 3.3–4.7 ppm. Successful introduction of deoxycholic acid (DOCA) and glycyrrhetinic acid (GA) into HA polymers was confirmed by the characteristic peaks, which appeared in the range of 0.6–1.6 ppm for DOCA and 1.0–1.4 ppm for GA (Li et al., Citation2012; Zhang et al., Citation2013a). In addition, the peaks at 8.04 and 8.02 ppm further confirmed the formation of new amide linkages in HA-adh-DOCA and HA-adh-GA conjugate, respectively.
Figure 1. (A) Synthetic route of HA-adh-DOCA conjugate and HA-adh-GA conjugate. (B) 1H NMR spectra of HA (B1), DOCA (B2), GA (B3), HA-adh-DOCA conjugate (B4) and HA-adh-GA conjugate (B5). (C) DSC profiles of HA-adh-DOCA10 conjugate (C1); silybin (C2); physical mixture of HA-adh-DOCA10 conjugate and silybin (C3); and silybin-loaded HA-adh-DOCA10 micelles (C4). (D) TEM image of silybin-loaded HA-adh-DOCA10 micelles (D1) and silybin-loaded HA-adh-GA20 micelles (D2). The magnification bar denotes 100 nm.
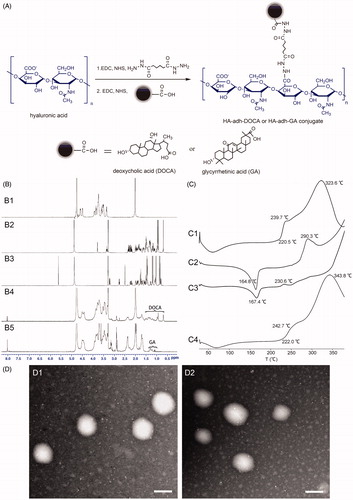
The degree of substitution (DS), defined as the average number of DOCA or GA molecule groups attached per 100 HA molecule, was determined by 1H NMR and UV spectrometry. The highest DS value was controlled around 10% for HA-adh-DOCA conjugate and 20% for HA-adh-GA conjugate, in that greater DS values resulted in water insolubility of conjugates due to the high hydrophobicity. Finally, HA-adh-DOCA10 (the DS of DOCA was 10%) conjugate and HA-adh-GA20 (the DS of GA was 20%) conjugate were chosen as the representative candidate for further studies because they can form self-assembled micelles bearing more hydrophobic inner core that can act as container for poorly water-soluble therapeutics (Choi et al., Citation2010).
Characterization of silybin-loaded micelles
The main physicochemical characteristics of silybin-loaded micelles are summarized in . Silybin were encapsulated efficiently in HA-adh-DOCA and HA-adh-GA micelles by using sonication method in water environment. The highest drug-loading (DL) of silybin was 20.3 and 20.6%, obtained from HA-adh-DOCA10 micelles and HA-adh-GA20 micelles, respectively. It should be emphasized that when silybin was loaded into micelles, the solubility in water was increased many times. For example, at the highest DL, the effective concentration of silybin in HA-adh-GA10 micelles was 2.2 mg/mL, which is 41.1-fold higher than its inherent water solubility of 43 μg/mL (Gazak et al., Citation2004). The results confirmed the presence of hydrophobic cores of micelles and the highly hydrophobic silybin functions as a hydrophobe in the core-forming process (Mahmud et al., Citation2007). Additionally, besides excellent DL, the entrapment efficiency of silybin-loaded micelles was quite high. Considering the expense of drug, high drug entrapment efficiency will be beneficial in reducing the final product cost.
Table 1. Characteristics of silybin-loaded HA-adh-DOCA10 micelles and HA-adh-GA20 micelles.
It has been well accepted that particle size is a critical parameter to be controlled, in that it might play a key role in determining the fate of nanocarriers after oral administration (Cai et al., Citation2010). Generally, small size particles (<100 nm) can be absorbed by endocytosis through enterocytes, while larger particles (>200 nm) are more likely uptaken and translocated via M cells (des Rieux et al., Citation2007; Mathot et al., Citation2007). As shown in , dynamic light scattering (DLS) measurement revealed that the mean particle size of two silybin-loaded micelles was around 130 nm and the size distribution was in the range of 60.3–269.7 nm. Therefore, silybin-loaded micelles might be transported in the small intestine by multiple pathways including endocytosis by enterocytes and M cells. TEM images revealed the nearly spherical shapes of silybin-loaded HA-adh-DOCA10 and HA-adh-GA20 micelles and similar particle size as measured using DLS (). Furthermore, the zeta potential of silybin-loaded micelles was below −25 mV (), which suggests that the micelles obtained in this study could repel each other and prevent aggregation or precipitation, thus resulting in good stability (Zou et al., Citation2012). Based on the above results, the two silybin-loaded micelles were expected to increase the uptake of intestinal cell and enhance the bioavailability of silybin.
DSC profile of HA-adh-DOCA10 conjugate, silybin, the physical mixture of silybin and HA-adh-DOCA10 conjugate and silybin-loaded HA-adh-DOCA10 micelles were shown in . HA-adh-DOCA10 conjugate showed a main exothermic peak at 323.6 °C (, C1), while silybin showed an exothermic peak at 164.8 °C and an endothermic peak at 290.3 °C (, C2). The typical peaks for silybin existed in the physical mixture of silybin and HA-adh-DOCA10 conjugate (, C3). In contrast, the silybin-loaded HA-adh-DOCA10 micelles only showed a similar calorimetric curve to HA-adh-DOCA10 conjugate, while the peaks of silybin disappeared (, C4). The DSC profile of silybin-loaded HA-adh-GA20 micelles was similar to that of silybin-loaded HA-adh-DOCA10 micelles (see Supplementary Figure S1). These results indicate that silybin was dispersed in an amorphous state or molecular state inside the micelles.
Stability in simulated gastrointestinal fluids
To evaluate the stability of micelles after oral delivery, we incubated silybin-loaded micelles in different pH value to simulate the environment of gastrointestinal tract (SGF, pH = 1.2; SIF, pH = 6.8) and blood circulation (PBS, pH = 7.4). As shown in , the mean particle size, polydispersity index and zeta potential of silybin-loaded HA-adh-DOCA10 and HA-adh-GA20 micelles in SIF and PBS were almost the same as the initial state. When SGF served as the medium, however, the mean particle size increased a little and the zeta potential was elevated to nearly neutral charge, suggesting a partial aggregation of micelles in SGF. These results were mainly due to the formation of –COOH from carboxylic acid group of HA in acidic environment, which significantly increased the zeta potential of micelles and reduced the electric repulsion among nanoparticles, leading to the aggregation of nanoparticles and increase of particle size in SGF. However, under the neutral pH conditions, the zeta potential of micelles was around the value of −25 mV owing to the formation of –COO−, which could offer more compact micelles and then increased the stability. Moreover, when the samples were transferred from SGF to SIF after 2 h incubation, the aggregated particles re-dispersed into smaller particles within 30 min, which were similar to the initial particle size (data not shown). Therefore, silybin-loaded HA-adh-DOCA10 and HA-adh-GA20 micelles could maintain relative stability in the gastrointestinal tract, which would protect silybin against inactivation in the harsh biological environment.
Table 2. Stability studies of silybin-loaded HA-adh-DOCA10 micelles and HA-adh-GA20 micelles in simulated gastrointestinal fluids and PBS (pH 7.4).
In vitro drug release studies
For both silybin-loaded micelles, the release profiles exhibited a steady continued-release pattern and no initial burst release was observed (). In simulated gastrointestinal fluids, a very small amount of silybin (<8%) was released within 2 h in SGF. After changing the release media to SIF, silybin-loaded HA-adh-DOCA10 and HA-adh-GA20 micelles released 18.9% ± 2.1% and 16.9% ± 2.6% of the initially loaded drug within 8 h, respectively. It was also found that the rates of drug release in PBS were slower than that in SGF/SIF. For instance, the cumulative release of silybin in both micellar formulations were less than 11% over a period of 8 h in PBS. The steady continued-release of silybin form micelles could protect the drug from rapid leakage, precipitation and degradation after oral administration, and probably increased drug absorption through intact form of micelles (Dahmani et al., Citation2012). The release characteristics of micelles could be explained by the reasons as follows. Firstly, hyaluronic acid formed an entangled network layer on the particle surface, which restricts the entry of water into the inner core as well as prevents diffusion of drug molecules from the core to the surrounding medium. Moreover, the strong hydrophobic interactions between silybin and the hydrophobic segments (deoxycholic acid and glycyrrhetinic acid) of conjugates also delayed the drug release.
Figure 2. Cumulative released amount of silybin as a function of time in SGF (pH = 1.2, 0–2 h) and SIF (pH = 6.8, 2–48 h) and in PBS (pH = 7.4, 0–48 h). Data are represented as the mean ± S.D. (n = 3).
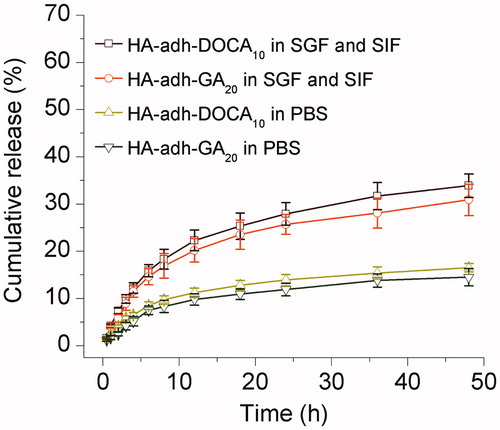
In this study, silybin-loaded HA-adh-DOCA10 showed a slight increased release rate compared to silybin-loaded HA-adh-GA20 micelles. This might be due to the more hydrophobic inner core of HA-adh-GA20 micelles than that of HA-adh-DOCA10 micelles.
In situ SPIP studies
Stability and binding studies
Before commencing the in suit single-pass intestinal perfusion (SPIP) studies, stability and binding studies were conducted. For the stability and binding studies, more than 98.5% of silybin was detected in two micellar formulations at 2 h compared with 0 h. These results indicated that silybin-loaded micelles were stable in the perfusates and hardly bind to the intestinal wall. Therefore, any loss of silybin-loaded micelles from the perfusates could be attributing to the intestinal absorption.
SPIP study of silybin-loaded micelles
In situ perfusion models were performed with silybin-loaded HA-adh-DOCA10 and HA-adh-GA20 micelles in Sprague-Dawley rats. The absorption of silybin at different drug concentrations was also carried out to investigate the intestinal absorption mechanism of micelles. As shown in , good absorption of silybin-loaded HA-adh-DOCA10 and HA-adh-GA20 micelles were occurred in the whole intestine tract. As demonstrated above, silybin-loaded micelles would maintain their particular structure in simulated gastrointestinal fluid and intestinal perfusates. Meanwhile, the delayed drug release characteristics could prevent the rapid drug leakage during delivery. Herein, the absorption of silybin in the whole intestine was achieved by encapsulated in HA-adh-DOCA10 and HA-adh-GA20 micelles, which were transported through whole intestine mainly via an endocytic pathway by enterocytes or M cells (Mathot et al., Citation2007; Li et al., Citation2010).
Figure 3. (A) Effective permeability (Peff) of silybin-loaded HA-adh-DOCA10 and HA-adh-GA20 micelles using single-pass intestinal perfusion. (B) Influence of different drug concentrations on the Peff of silybin across rat ileum segment. (C) Plasma concentration-time profiles of silybin after oral administration of silybin suspension, silybin-loaded HA-adh-DOCA10 micelles and silybin-loaded HA-adh-GA20 micelles at a dose of 30 mg/kg to Sprague-Dawley rats. Data are represented as mean ± S.D. (n = 6).
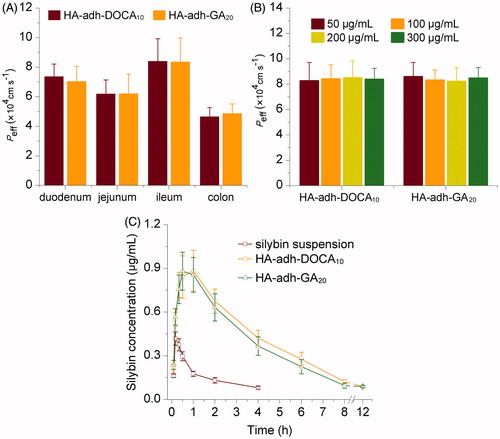
In this study, ileum segment was selected for the study at different drug concentrations, in that it exhibited higher absorption ability than duodenum, jejunum and colon segments (). Lee et al. (Citation2001) and Kim et al. (Citation2011) reported that deoxycholic acid would increase the absorption of macromolecules, such as heparin, into the intestinal, through interactions with bile acid transporters expressed on the ileum membrane. However, the permeability of silybin-loaded HA-adh-DOCA10 micelles at 50, 100, 200 and 300 μg/mL was (8.26 ± 1.44) × 10−4, (8.41 ± 1.11) × 10−4, (8.49 ± 1.36) × 10−4 and (8.38 ± 0.87) × 10−4 cm/s, respectively, showing no significant difference among different drug concentrations (p > 0.05). The result indicated that receptor-mediated uptake of HA-adh-DOCA10 conjugates in intestinal was affected due to the formation of self-assembled micelles in the aqueous solution. Deoxycholic acid, located within the interior of the micelles, can hardly interact with the transporters expressed on the ileum membrane. The permeability of silybin-loaded HA-adh-GA20 micelles at different drug concentration was similar with that of silybin-loaded HA-adh-DOCA10 micelles. Therefore, when drug concentration was during 50–300 μg/mL, silybin-loaded HA-adh-DOCA10 and HA-adh-GA20 micelles were transported through intestine via a passive diffusion mechanism.
In vivo pharmacokinetic studies in rat
The in vivo absorption of silybin-loaded micelles and silybin suspension was assessed in Sprague-Dawley rats after a single oral dose (30 mg/kg). The plasma concentration of silybin versus time profile is illustrated in . Silybin was very poorly absorbed in the suspension formulation and undetectable beyond 4 h in the circulation system. On the contrary, significant increase in absorption was observed with the silybin-loaded HA-adh-DOCA10 and HA-adh-GA20 micelles. The silybin level from two micellar formulations remained higher at each tested time point compared with those from silybin suspension. It was noteworthy that the drug was detectable in the plasma up to 12 h for two micellar formulations.
The mean pharmacokinetic parameters from different formulations, obtained by non-compartmental model, are summarized in . It was exhibited that there was no significant difference between silybin-loaded HA-adh-DOCA10 and HA-adh-GA20 micelles (p > 0.05), while they had a 4.38 and 4.03 times increase in AUC value and 2.12 and 2.10 times increase in Cmax compared with the suspension formulation, respectively. For the mean residence time (MRT), silybin-loaded HA-adh-DOCA10 and HA-adh-GA20 micelles was significantly longer than silybin suspension (p < 0.05).
Table 3. Pharmacokinetic parameters of silybin formulations after a single oral dose of 30 mg/kg to Sprague-Dawley rats.
This remarkable capacity for improving the oral absorption and bioavailability of silybin by HA-adh-DOCA10 and HA-adh-GA20 micelles might be determined by several key factors as follows. Firstly, the micelles displayed relative stability and steady continued-release characteristics in gastrointestinal fluids, which could prevent the rapid drug leakage and precipitation after oral administration. Secondly, taking advantage of the small particle size (∼130 nm), silybin-loaded micelles could be absorbed through an endocytic pathway by enterocytes and M cells to enter the blood and lymphatic circulation, respectively. Lastly, as some researchers reported, the hydrophilic corona of micelles could reduce the binding of serum proteins to drug, resulting in prolonged half-life (Alexis et al., Citation2008). All the above stated facts could explain the improved silybin oral bioavailability with HA-adh-DOCA10 and HA-adh-GA20 micelles.
In vivo imaging analysis
In this study, the HA-adh-DOCA10 and HA-adh-GA20 conjugates displayed pharmaceutically acceptable physicochemical properties and improved oral absorption of silybin. To further investigate the liver targeting drug delivery capacity of HA-adh-DOCA10 and HA-adh-GA20 micelles, the biodistribution of micelles in mice was analyzed by fluorescence imaging using an encapsulated NIRF dye (DiR) as indicator. As shown in , an obvious accumulation of DiR was observed in liver tissues relative to the other parts of mice bodies at each tested time point. Concretely, DiR-loaded micelles began to accumulate in the liver as early as 1 h, reached the maximum fluorescent intensity at 6 h, and then declined gradually, but still detectable until 12 h. These results provided decisive evidence for the accumulation of micelles in liver tissue, indicating that the micelles could assist silybin targeting to the liver organ. The ex vivo images of excised organs at the end of the test further confirmed the results observed above. As seen in , a remarkably stronger fluorescence signal was observed in the liver than that of other main organs. Interestingly, it was found that HA-adh-GA20 micelles shown stronger fluorescence signal in liver at all predetermined intervals than HA-adh-DOCA10 micelles.
Figure 4. In vivo imaging of ICR mice administrated with DiR-loaded micelles. (A) Images were taken after oral administration of HA-adh-DOCA10 and HA-adh-GA20 micelles at 1 h, 6 h and 12 h, respectively. (B) Ex vivo fluorescence images of tissues collected at 12 h post-administration of HA-adh-DOCA10 and HA-adh-GA20 micelles.
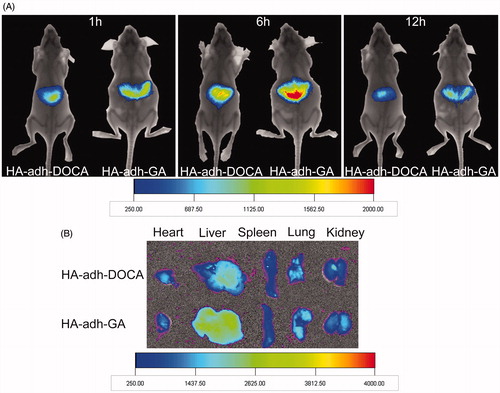
Several investigations have demonstrated that hyaluronic acid receptor for endocytosis (HARE) in liver sinusoidal endothelial cells is one of the specific receptors of the HA (Oh et al., Citation2010). The high liver accumulation of micelles might be due to the HA receptor-mediated endocytosis of HA-adh-DOCA10 and HA-adh-GA20 micelles into liver tissue. Moreover, the effects of non-specific binding and uptake by the reticuloendothelial system (RES) in liver could not be ignored. In comparison between the two micellar formulations, HA-adh-GA20 micelles had superior targeted drug delivery effects compared with HA-adh-DOCA10 micelles. The difference between HA-adh-DOCA10 and HA-adh-GA20 micelles was ascribed to the different hydrophobic moiety. Studies have described that GA-modified drug delivery systems accumulated particularly in the rat liver and maintained at a high level, because of the abundant receptors for GA on hepatocyte membranes (Zhang et al., Citation2012; Rohilla et al., Citation2014). In this study, although most of the hydrophobic GA molecules were embedded in the inner core of micelles, some GA molecules were still present on the surface of HA-adh-GA20 micelles, serving as liver-targeting ligands. Considering that both HA and GA are specific ligands to liver, the higher liver targeting capacity of HA-adh-GA20 micelles in vivo might be attributed to combined action of HA and GA.
In vivo assessment of silybin-loaded micelles against liver injury
Based on the above studies, HA-adh-DOCA10 and HA-adh-GA20 conjugates proved to be able to deliver the encapsulated drug to liver tissue. To better understand the practical superiority of silybin-loaded HA-adh-DOCA10 and HA-adh-GA20 micelles over silybin suspension, it is necessary to evaluate their in vivo hepato-protective efficacy. Mice were orally administrated with different formulations for consecutive 7 days and then treated with CCl4 to induce acute liver injury. The evaluation of the extent of liver injury in the mice was based on an analysis of serum ALT and AST activities (). Compared to the normal control group, the CCl4 group resulted in significant increasing of the AST and ALT activity (p < 0.001), indicating CCl4-induced damage to the hepatic cells. Conversely, three silybin formulations significantly decreased the elevated ALT and AST activity to the normal level, which implied that the formulations could attenuate severe liver injury. Moreover, the silybin-loaded HA-adh-GA20 micelles showed lower level of ALT and AST in comparison with that of silybin suspension and silybin-loaded HA-adh-DOCA10 micelles, which indicated better recovery of liver function using silybin-loaded HA-adh-GA20 micelles.
Figure 5. (A) Effects of silybin suspension, silybin-loaded HA-adh-DOCA10 micelles and silybin-loaded HA-adh-GA20 micelles on the AST and ALT activity. Each of the data was presented as mean ± SD (n = 10). ***p < 0.001 versus CCl4 group. #p < 0.05, ##p < 0.01 and ###p < 0.001 versus silybin-loaded HA-adh-GA20 micelles group. (B) Histological analysis of liver slices after treatment of saline (B1, normal control), CCl4 (B2), silybin suspension formulation (B3), silybin-loaded HA-adh-DOCA10 micelles (B4) and silybin-loaded HA-adh-GA20 micelles (B5) by H & E staining. All the images were captured by the Nikon microscope at a 200× magnification of the original images.
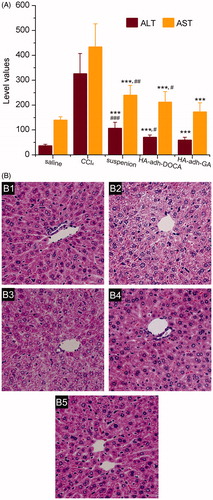
Histological assessment of liver was performed to further support the biochemical analysis. Liver sections from mice were examined using H & E staining and shown in . The normal hepatic cells showed well-preserved cytoplasm, prominent nucleus, radially arranged hepatocytes around the central vein and well-defined sinusoidal line. However, CCl4-treated mice showed severe cytoplasmic vacuolation, microvesicular fatty changes and inflammation, compared with the normal control group. Those abnormal morphological changes were remarkably ameliorated in the silybin-loaded HA-adh-DOCA10 micelles and silybin-loaded HA-adh-GA20 micelles, compared to the silybin suspension. Interestingly, mice pretreated with silybin-loaded HA-adh-GA20 micelles showed near normalization of liver tissues with no significant changes in hepatocytes. The histological analysis of three silybin formulations was in accordance with the results of the biochemical parameters of hepatic function.
Conclusion
In this study, amphiphilic hyaluronic acid-deoxycholic acid (HA-adh-DOCA) conjugates and hyaluronic acid-glycyrrhetinic acid (HA-adh-GA) conjugates have been successfully developed in order to encapsulate poorly water-soluble drug silybin. Silybin-loaded HA-adh-DOCA10 and HA-adh-GA20 micelles displayed high drug-loading capacity, narrow size distribution around 130 nm and good stability. In vitro drug release study showed that two silybin-loaded micelles exhibited similar steady continued-release pattern in simulated gastrointestinal fluids (pH 1.2/pH 6.8) and PBS (pH 7.4). The silybin-loaded micelles could be absorbed in the whole intestine through a passive diffusion mechanism and achieved significantly higher oral bioavailability compared with suspension formulation. In vivo imaging analysis confirmed the liver targeting activity of micelles after oral administration. In comparison between the two micellar formulations, the fluorescence signals of HA-adh-GA20 micelles in liver were stronger than that of HA-adh-DOCA10 micelles, owing to the active hepatic-targeting properties of glycyrrhetinic acid. In the liver injury mice model induced by CCl4, silybin-loaded HA-adh-GA20 micelles displayed better therapeutic effects over suspension control and silybin-loaded HA-adh-DOCA10 micelles. Therefore, HA-adh-GA20 conjugates show great potential for improving the bioavailability and liver targetability of silybin after oral administration.
Supplementary material available online
Supplementary Figure S1
Supplementary Figure S1
Download PDF (44.1 KB)Declaration of interest
The authors report no conflicts of interest. This work was supported by the project of the National Natural Science Foundation of China (No. 81302727), Beijing Natural Science Foundation (No. 7152020) and Special Research Fund for Traditional Chinese Medicine in Capital (No. 13ZY08).
References
- Abenavoli L, Capasso R, Milic N, et al. (2010). Milk thistle in liver diseases: past, present, future. Phytother Res 24:1423–32
- Ahn HK, Jung M, Sym SJ, et al. (2014). A phase II trial of Cremorphor EL-free paclitaxel (Genexol-PM) and gemcitabine in patients with advanced non-small cell lung cancer. Cancer Chemother Pharmacol 74:277–82
- Al-Hilal TA, Alam F, Byun Y. (2013). Oral drug delivery systems using chemical conjugates or physical complexes. Adv Drug Deliv Rev 65:845–64
- Alexis F, Pridgen E, Molnar LK, et al. (2008). Factors affecting the clearance and biodistribution of polymeric nanoparticles. Mol Pharm 5:505–15
- Almeida PV, Shahbazi MA, Makila E, et al. (2014). Amine-modified hyaluronic acid-functionalized porous silicon nanoparticles for targeting breast cancer tumors. Nanoscale 6:10377–87
- Angelico R, Ceglie A, Sacco P, et al. (2014). Phyto-liposomes as nanoshuttles for water-insoluble silybin-phospholipid complex. Int J Pharm 471:173–81
- Cai Z, Wang Y, Zhu LJ, et al. (2010). Nanocarriers: a general strategy for enhancement of oral bioavailability of poorly absorbed or pre-systemically metabolized drugs. Curr Drug Metab 11:197–207
- Cao X, Deng W, Fu M, et al. (2013). Seventy-two-hour release formulation of the poorly soluble drug silybin based on porous silica nanoparticles: in vitro release kinetics and in vitro/in vivo correlations in beagle dogs. Eur J Pharm Sci 48:64–71
- Choi KY, Chung H, Min KH, et al. (2010). Self-assembled hyaluronic acid nanoparticles for active tumor targeting. Biomaterials 31:106–14
- Croy SR, Kwon GS. (2006). Polymeric micelles for drug delivery. Curr Pharm Des 12:4669–84
- Dahmani FZ, Yang H, Zhou J, et al. (2012). Enhanced oral bioavailability of paclitaxel in pluronic/LHR mixed polymeric micelles: preparation, in vitro and in vivo evaluation. Eur J Pharm Sci 47:179–89
- des Rieux A, Fievez V, Theate I, et al. (2007). An improved in vitro model of human intestinal follicle-associated epithelium to study nanoparticle transport by M cells. Eur J Pharm Sci 30:380–91
- El-Samaligy MS, Afifi NN, Mahmoud EA. (2006). Increasing bioavailability of silymarin using a buccal liposomal delivery system: preparation and experimental design investigation. Int J Pharm 308:140–8
- Gazak R, Svobodova A, Psotova J, et al. (2004). Oxidised derivatives of silybin and their antiradical and antioxidant activity. Bioorg Med Chem 12:5677–87
- Kim SK, Huh J, Kim SY, et al. (2011). Physicochemical conjugation with deoxycholic acid and dimethylsulfoxide for heparin oral delivery. Bioconjug Chem 22:1451–8
- Kvasnicka F, Biba B, Sevcik R, et al. (2003). Analysis of the active components of silymarin. J Chromatogr A 990:239–45
- Laffleur F, Roggla J, Idrees MA, et al. (2014). Chemical modification of hyaluronic acid for intraoral application. J Pharm Sci 103:2414–23
- Lee Y, Nam JH, Shin HC, et al. (2001). Conjugation of low-molecular-weight heparin and deoxycholic acid for the development of a new oral anticoagulant agent. Circulation 104:3116–20
- Li H, Huo M, Zhou J, et al. (2010). Enhanced oral absorption of paclitaxel in N-deoxycholic acid-N,O-hydroxyethyl chitosan micellar system. J Pharm Sci 99:4543–53
- Li J, Huo M, Wang J, et al. (2012). Redox-sensitive micelles self-assembled from amphiphilic hyaluronic acid-deoxycholic acid conjugates for targeted intracellular delivery of paclitaxel. Biomaterials 33:2310–20
- Li J, Yin T, Wang L, et al. (2015). Biological evaluation of redox-sensitive micelles based on hyaluronic acid-deoxycholic acid conjugates for tumor-specific delivery of paclitaxel. Int J Pharm 483:38–48
- Lian H, Zhang T, Sun J, et al. (2013). Enhanced oral delivery of paclitaxel using acetylcysteine functionalized chitosan-vitamin E succinate nanomicelles based on a mucus bioadhesion and penetration mechanism. Mol Pharm 10:3447–58
- Liang N, Sun S, Hong J, et al. (2015). In vivo pharmacokinetics, biodistribution and antitumor effect of paclitaxel-loaded micelles based on alpha-tocopherol succinate-modified chitosan. Drug Deliv. [Epub ahead of print]. DOI: 10.3109/10717544.2015.1045103
- Liang Z, Yang Y, Wang H, et al. (2014). Inhibition of SIRT1 signaling sensitizes the antitumor activity of silybin against human lung adenocarcinoma cells in vitro and in vivo. Mol Cancer Ther 13:1860–72
- Lin J, Wang X, Wu Q, et al. (2014). Development of Salvianolic acid B-Tanshinone II A-Glycyrrhetinic acid compound liposomes: formulation optimization and its effects on proliferation of hepatic stellate cells. Int J Pharm 462:11–18
- Liu Q, Li J, Pu G, et al. (2015). Co-delivery of baicalein and doxorubicin by hyaluronic acid decorated nanostructured lipid carriers for breast cancer therapy. Drug Deliv. [Epub ahead of print]. DOI: 10.3109/10717544.2015.1031295
- Luo Y, Prestwich GD. (1999). Synthesis and selective cytotoxicity of a hyaluronic acid-antitumor bioconjugate. Bioconjug Chem 10:755–63
- Mahmud A, Xiong XB, Aliabadi HM, et al. (2007). Polymeric micelles for drug targeting. J Drug Target 15:553–84
- Mathot F, des Rieux A, Arien A, et al. (2007). Transport mechanisms of mmePEG750P(CL-co-TMC) polymeric micelles across the intestinal barrier. J Control Release 124:134–43
- Matsumura Y. (2006). [Micelle carrier system in clinical trial]. Nihon Rinsho 64:316–21
- Oh EJ, Park K, Kim KS, et al. (2010). Target specific and long-acting delivery of protein, peptide, and nucleotide therapeutics using hyaluronic acid derivatives. J Control Release 141:2–12
- Ohya Y, Takeda S, Shibata Y, et al. (2011). Evaluation of polyanion-coated biodegradable polymeric micelles as drug delivery vehicles. J Control Release 155:104–10
- Rainone F. (2005). Milk thistle. Am Fam Physician 72:1285–8
- Ramasamy K, Agarwal R. (2008). Multitargeted therapy of cancer by silymarin. Cancer Lett 269:352–62
- Rezazadeh M, Emami J, Hasanzadeh F, et al. (2015). In vivo pharmacokinetics, biodistribution and anti-tumor effect of paclitaxel-loaded targeted chitosan-based polymeric micelle. Drug Deliv. [Epub ahead of print]. DOI: 10.3109/10717544.2014.954281
- Rohilla R, Garg T, Bariwal J, et al. (2014). Development, optimization and characterization of glycyrrhetinic acid-chitosan nanoparticles of atorvastatin for liver targeting. Drug Deliv. [Epub ahead of print]. DOI: 10.3109/10717544.2014.977460
- Rohilla R, Garg T, Goyal AK, et al. (2015). Herbal and polymeric approaches for liver-targeting drug delivery: novel strategies and their significance. Drug Deliv. [Epub ahead of print]. DOI: 10.3109/10717544.2014.945018
- Takei Y, Maruyama A, Ferdous A, et al. (2004). Targeted gene delivery to sinusoidal endothelial cells: DNA nanoassociate bearing hyaluronan-glycocalyx. FASEB J 18:699–701
- Trappoliere M, Caligiuri A, Schmid M, et al. (2009). Silybin, a component of sylimarin, exerts anti-inflammatory and anti-fibrogenic effects on human hepatic stellate cells. J Hepatol 50:1102–11
- Wang Y, Wang L, Liu Z, et al. (2012). In vivo evaluation of silybin nanosuspensions targeting liver. J Biomed Nanotechnol 8:760–9
- Wang Y, Zhang L, Wang Q, et al. (2014). Recent advances in the nanotechnology-based drug delivery of Silybin. J Biomed Nanotechnol 10:543–58
- Zhang C, Wang W, Liu T, et al. (2012). Doxorubicin-loaded glycyrrhetinic acid-modified alginate nanoparticles for liver tumor chemotherapy. Biomaterials 33:2187–96
- Zhang L, Yao J, Zhou J, et al. (2013a). Glycyrrhetinic acid-graft-hyaluronic acid conjugate as a carrier for synergistic targeted delivery of antitumor drugs. Int J Pharm 441:654–64
- Zhang S, Yang Y, Liang Z, et al. (2013b). Silybin-mediated inhibition of Notch signaling exerts antitumor activity in human hepatocellular carcinoma cells. PLoS One 8:e83699
- Zou A, Huo M, Zhang Y, et al. (2012). Octreotide-modified N-octyl-O,N-carboxymethyl chitosan micelles as potential carriers for targeted antitumor drug delivery. J Pharm Sci 101:627–40