Abstract
Lung cancer poses one of the most significant challenges to modern medicine, killing thousands every year. Current therapy involves surgical resection supplemented with chemotherapy and radiotherapy due to high rates of relapse. Shortcomings of currently available chemotherapy protocols include unacceptably high levels of systemic toxicity and low accumulation of drug at the tumor site. Loco-regional delivery of nanocarriers loaded with anticancer agents has the potential to significantly increase efficacy, while minimizing systemic toxicity to anticancer agents. Local drug administration at the tumor site using nanoparticulate drug delivery systems can reduce systemic toxicities observed with intravenously administered anticancer drugs. In addition, this approach presents an opportunity for sustained delivery of anticancer drug over an extended period of time. Herein, the progress in the development of locally administered nanomedicines for the treatment of lung cancer is reviewed. Administration by inhalation, intratumoral injection and means of direct in situ application are discussed, the benefits and drawbacks of each modality are explored.
Introduction
Lung cancer is one of the most significant medical challenges in Australia and worldwide (Joshi et al., Citation2011). According to the Australian Bureau of Statistics, in 2011, cancer-related deaths made up 30% of all deaths in Australia, with lung cancer being the fourth biggest killer in the country behind ischemic heart disease, Alzheimer’s disease and dementia, and cerebrovascular diseases (Australian Bureau of Statistics, Citation2015). Among cancers, it is by far the most deadly tumor in both males and females, killing more than breast cancer, prostate cancer, and melanoma diseases (Parker, Citation2001; Australian Bureau of Statistics, Citation2006).
Lungs are a common site of involvement by primary and metastatic malignancy, being highly exposed to the external and internal environment through airflow and pulmonary blood flow respectively (Rao et al., Citation2003). Lung cancer is characterized by uncontrolled cellular growth in the tissue of the lung that may metastasize and spread into other tissue in the lung or elsewhere in the body (Jinturkar et al., Citation2012). Of the known types of lung cancer, there are two main forms – small cell lung carcinomas (SCLC) and non-small cell lung carcinomas (NSCLC), of these, the majority of lung cancers fall into the latter category (∼80%) with a significant proportion of the remainder falling into the category of SCLC (Celikoglu et al., Citation2008; Jinturkar et al., Citation2012). Despite significant research and developments in the detection and treatment of NSCLC, the majority of tumors are not detected until the cancer is at an advanced stage, meaning the prognosis is poor and treatment options are limited. SCLC sufferers have a very poor prognosis with less than 10% survival rates at 5 years (Jinturkar et al., Citation2012; Zarogoulidis et al., Citation2012a). The preferred treatment option is surgical resection of the cancerous tissue; however, being difficult to ensure that all of the cancerous tissue is removed, resection is supplemented with radiotherapy or chemotherapy (Kang et al., Citation2011; Jinturkar et al., Citation2012).
Current chemotherapy approach employs high dosage of intravenous cytotoxic drugs. These treatments in many cases display limited efficacy due to poor selectivity, dose-limiting levels of systemic toxicity (including anemia, nausea, vomiting, neurotoxicity, and nephrotoxicity), and the development of multidrug resistance such as that seen with P-glycoprotein and multi-drug-resistant pumps (Tseng et al., Citation2009; Jinturkar et al., Citation2012; Videira et al., Citation2012). Furthermore, many of the most effective treatments for lung cancer such as paclitaxel, docetaxel, doxorubicin, camptothecins, and quercetin are highly lipophilic molecules with very poor aqueous solubility (El-Gendy & Berkland, Citation2009; Willis et al., Citation2012; Verma et al., Citation2013). Low aqueous solubility coupled with unacceptable systemic toxicity limit the therapeutic effect of the commercially available formulations of the aforementioned drugs, with low levels of drug actually reaching the site of action (Tseng et al., Citation2009; Zarogoulidis et al., Citation2012a).
For this reason, much research has been focused on developing new mechanisms of delivery that will maximize drug concentration in the cancerous tissue while minimizing exposure of major organs to limit serious systemic toxicity. Indeed, many of the drugs currently delivered by systemic administration (5-fluorouracyl, cisplatin, doxorubicin, pemetrexed, gemcitabine, interleukin-2 (IL2), and many others) have the potential to be delivered loco-regionally, minimizing exposure of vital organs to cytotoxic agents and reducing side effects (De Souza et al., Citation2010; Zarogoulidis et al., Citation2012a). In addition, the concept of developing a chemotherapeutic therapy for the cancer patient not requiring hospitalization, such as an aerosolized treatment to be administered at home, avoiding all the accompanied costs and systemic side effects of a traditional chemotherapy, appears particularly appeal.
Clinical studies of aerosolized chemotherapy date back to 1967, but despite promising preclinical and encouraging early clinical studies, progresses have been limited and few investigations have been carried out. Results have been hampered by relatively fast drug organ elimination kinetics. Even if with pulmonary delivery high local concentrations can be achieved, they are often short-lived as the drug can be quickly removed from the lung through various clearance mechanisms, such as mucociliary clearance and reticulo-endothelial system (RES) cells uptake. To improve the inhaled chemotherapy approach delivery systems able to enhance the treatment efficacy of the drug by increasing pulmonary residence time and reducing lung clearance, are needed (Lebhardt et al., Citation2011). At the same time some concerns have been raised about safety and organ toxicity of the treatment. In fact, adverse respiratory effect, such as acute form of pneumonitis, pulmonary edema, and alveolar hemorrhage, were observed in few animal studies (Zarogoulidis et al., Citation2012b).
In the last two decades, the formulation of chemotherapeutic drugs into nanoparticles has been object of intense investigation and expectations that these carriers would improve cancer therapy have been high. In fact, nanomedicines, i.e. drug-loaded into nano-sized drug delivery systems, such as liposomes, micelles and polymeric or lipid nanoparticles, offers several advantages to improve delivery of chemotherapeutics. Such advantages can involve drug solubilization and protection from degradation, control of drug release, evasion of the RES, prolonged exposure of the tumor to the drug, and accumulation in the tumor parenchyma, as a consequence of tumor blood vessels leakiness and lower accumulation in healthy organs (Blanco et al., Citation2011).
Quite interestingly, some of the investigated nanocarriers have been proposed for local or regional delivery, which looks to be a promising way forward in the management of difficult-to-treat cancers. The use of nanoparticles actually helps to focus the administration to the diseased organ and limiting as much as possible the distribution of the nanoformulation to surrounding tissues.
This approach appears especially interesting because of the benefit of pulmonary administration (non-invasive, no first-pass metabolism, local treatment) with the nanocarrier systems advantages (enhanced drug solubility, sustained-release, possibly reduced dosing frequency, delivery of macromolecules, limited side effects, improved patient compliance, uniform distribution in deep lung, possible preferential drug internalization by or targeting of diseased cells) (Feng et al., Citation2014; Kaur et al., Citation2015).
If efficacy is increased, decreased side effects can effectively be achieved with the development of loco-regional delivery of nanoparticle encapsulated chemotherapy formulations. It can be expected that an increased focus on research in this area has significant potential to have nationwide economic benefits as well as an improved prognosis and quality of life for lung cancer patients.
This review will focus on the recent research in the area of pharmaceutical nanotechnologies for loco-regional delivery of chemotherapeutic agents in the treatment of lung cancers. The methods of delivery that will be discussed include: inhalation; intratumoral injection and other technologies for local delivery such as films for implant and use of magnetic nanoparticles for magnetically directed targeting and hyperthermia. Both the benefits and challenges of each of the delivery approaches will be discussed in relation to research papers reporting clinical trials or clinically relevant animal models.
Aerosolized chemotherapy
One promising area of research for the treatment of lung diseases is drug delivery by inhalation. Loco-regional deposition of drugs in the lung via inhalation offers the potential for increased and sustained drug concentrations directly at the disease site (Gill et al., Citation2011). One considerable advantage of delivery by inhalation for the treatment of lung cancers is that it may allow for an increased accumulation of drug in the tumor. This effect can be further enhanced by loading the drugs into nanoparticles for increased solubility, improved retention time, or cellular uptake at the desired site of action (Videira et al., Citation2012).
However, nanoparticles delivery to the lung faces some important challenges, as some physiological and pathophysiological factors that can restrict the effectiveness of this mode of delivery. In fact, it has been shown that for an efficient deposition in the deep lung, aerosols should have optimal properties in term of aerodynamic particle size. If the particle size exceeds the threshold value of 5 µm, the particles tend to deposit in the upper airways including the main conducting airways, such as the larger bronchi, as well as the nose and throat where they can be rapidly cleared. If particles are smaller than 1 µm, they settle very slowly and can be exhaled before they are able to deposit in the lung. Ideally, the aerodynamic diameter of the particles needs to range between 1 µm and 5 µm, to obtain a substantial deep-lung deposition (). Furthermore, the presence of co-morbidities, such as chronic obstructive pulmonary disease (COPD), asthma, chronic inflammation, and cystic fibrosis may affect airway conduction. Such conditions can affect the angles of bifurcation in the lung due to lung remodeling, the degree of mucous production, and rate of mucociliary clearance, all of which will affect the deposition in the lung and the degree of accumulation in the tumor (Zarogoulidis et al., Citation2012a). Another barrier to the effectiveness of inhaled delivery is the size of the tumor. It is generally agreed that a tumor size greater than 5 cm will substantially reduce the efficacy of the inhaled chemotherapeutic agent to due poor access to the tumor site and changes in airflow patterns around the tumor (Kleinstreuer et al., Citation2008; Zhang et al., Citation2008).
Figure 1. Mass fractional deposition of particles in the lungs as a function of aerodynamic diameter, assuming that particles are spherical with unit density. Adapted by permission from Macmillan Publishers Ltd: Nature Reviews Drug Discovery (Patton & Byron, Citation2007), © 2007.
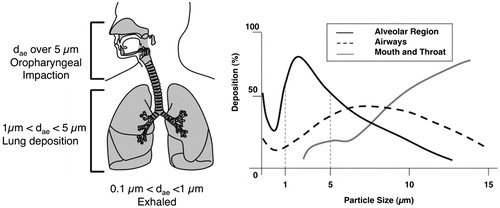
Finally, there have been a number of concerns raised regarding the safety of nanoparticles, particularly via the pulmonary route of administration. However, this is likely due to evidence obtained from epidemiological studies of inhaled pollutant nanoparticles by several groups (Ibald-Mulli et al., Citation2002; Hoet et al., Citation2004; Oberdörster et al., Citation2005) rather than studies focused specifically on inhaled nanomedicines. In this regard, a study by Dailey and co-workers compared two inhaled biodegradable polymer-based nanoparticle systems: the novel dimethylaminopropylaminopropylamine polyvinyl alcohol-grafted poly(lactic-co-glycolic acid) nanoparticles (DEAPA-PVAL-g-PLGA NP) and poly(lactic-co-glycolic acid) nanoparticles (PLGA NP), to non-biodegradable polystyrene-based nanoparticles (PS NP) of the same size (75 and 220 nm). It was found that the biodegradable systems produced reduced toxicity and inflammation in lungs of mice following intratracheal instillation. In particular, significantly increased levels of lactate dehydrogenase (a marker of cellular damage) and higher recruitment of polymorphonucleocyte was evidenced in animals treated with the PS NP, whereas no significant difference was found between the biodegradable systems, DEAPA-PVAL-g-PLGA NP and PLGA NP, and the negative control, an isotonic glucose solution (Dailey et al., Citation2006).
In another study, nanoparticles with different particle size (50–150 nm) and surface properties (hydrophobic versus hydrophilic) were used to assess acute respiratory toxicity when administered to the lungs of mice. Three different nanoparticles: polystyrene, polyvinyl acetate (PVAc), and PEGylated lipid nanoparticles were characterized for their surface properties by hydrophobic interaction chromatography (HIC) and classified according to a HIC index, a hydrophobicity scale ranging from 0.00 (hydrophilic) to 1.00 (hydrophobic). Only particles with higher hydrophobicity (HIC >0.8) showed dose-dependent inflammatory reaction with increase of neutrophils and inflammatory cytokines in bronchoalveolar lavage. The hydrophilic PEGylated lipid nanoparticles (HIC ∼0.5), selected as representative nanomedicine formulations for pulmonary delivery, elicited no inflammation and only mild evidence of tissue damage. Particle size did not appear to be a factor as relevant as surface hydrophobicity in affecting acute respiratory toxicity (Jones et al., Citation2014).
A recent comprehensive review regarding the safety of nebulized particles has found that many of the toxic effects observed to date are reduced when optimal distribution of the aerosol throughout the lung is achieved. However, the opposite is generally the case when high doses led to aggregation of droplets at particular sites; the toxicity observed was increased (Darwiche et al., Citation2013). This is an important consideration in obstructed airway diseases, which may lead to increased deposition of particles due to changes and interruption of airflow in specific lung regions or in the case of specific nanomaterials prone to agglomeration. For example, carbon nanotubes, despite having been indicated as promising drug delivery systems for a number of applications (Badrzadeh et al., Citation2015; Singh et al., Citation2015), tend to agglomerate and cause localized damage to airway epithelial monolayers, as a consequence of altered the apoptotic and proliferative rate of epithelial cells in close proximity to the aggregates (Rotoli et al., Citation2015).
The data collected by these investigations point out that the impact of the nanomedicines on the lung is influenced by a series of controllable factors such as material biodegradability, surface properties, particle size, surface area, and propensity toward particle aggregation as a consequence of interaction with biological fluids or of the aerosolization process employed by the delivery device.
Four clinically successful pulmonary aerosolizing devices are available namely, nebulizers, pressurized metered dose inhalers (pMDIs), dry powder inhalers (DPIs), and soft-mist inhalers (SMIs). Nebulizers produce liquid aerosols from few milliliters of solutions or suspensions using an external power supply: compressed gas flow in air jet nebulizers, piezoelectric crystals vibrating at high frequency in ultrasonic nebulizers, and an oscillating perforated membrane in vibrating mesh nebulizers. pMDIs use liquefied gases, hydrofluoroalkanes, as propellants and offer portability and convenience to patients. DPIs are breath-actuated devices and use the inhalation force of the patient to disaggregate microparticles to form an inhalable aerosol. SMIs are patient-independent devices that produce an aerosol mechanically, by passing few microliters of drug solution through two nozzles able to create converging jets of solution that collide, generating a fine aerosol of inhalable droplets (Zarogoulidis et al., Citation2012a; Muralidharan et al., Citation2015).
It has been evidenced in several works that the selection of the type of technology used for the aerosolization and the device characteristic is critical for nanoparticle delivery. The delivered fraction of the dose and the state of aggregation of the particles deposited in the lung is highly dependent on the combination of physicochemical properties of the nanoparticles and the stress acting on the material to create the aerosol within the device (Dailey et al., Citation2003; Hernández-Trejo et al., Citation2005).
Furthermore, the safety of the mode of administration needs also to be considered, with respect to potential toxicity toward health-care providers or family members in the immediate vicinity at the time of dosing. This appears a particular issue especially with nebulized formulations and will be discussed further in the following section (Darwiche et al., Citation2013).
Considering their relative low potencies, the dose necessary to treat a lung cancer patient via inhalation may well be in the order of milligrams (Carvalho et al., Citation2011). Hence, this section of the manuscript will focus on the description of chemotherapy nanoformulation to be delivered with nebulizers or DPI since these systems offer the possibility to deliver such doses of drug. Even if the formulation of anticancer drugs in metered dose inhalers (MDIs) has been described (Haynes et al., Citation2003; Fulzele et al., Citation2006), no reports have been found in literature of pMDI for the delivery of anticancer drug-loaded nanoparticles. In fact, pMDIs have been rarely used for nanoparticle formulations, as a consequence of some technical challenges such as the presence as a dispersant of a liquefied hydroflouroalkane gas instead of water and the difficulty in monitoring the aggregation status of nanoparticles within the pressurized canister and during the aerosolization phase.
Nebulizers
Nebulizers have been till date the most used devices for creating aerosols with nanoparticle formulations. Nanosystems for the treatment of lung cancers are water dispersions and nebulizer devices can produce less than 5 µm highly inhalable droplets aerosols loaded with nanoparticles. Viscosity, surface tension, pH, ionic strength, and osmolarity of the nanoparticle formulation will affect the aerosol created but apart from these adjustments, the formulation will be used directly in these devices without transformation. In addition, large doses of formulation can be delivered with a device requiring little or no patient coordination.
Jet nebulizers and ultrasonic nebulizers are the most commonly devices available and have been used clinically for many years. Despite their reliability and ease of administration, these devices present some drawbacks too, that are relevant for their application in chemotherapy.
Nebulized aerosol is generated continuously and the time necessary for the patient to inhale the required dose through a mouthpiece or facemask is generally of several minutes. Obviously, at least 50% of the aerosol will not be available, as a consequence patient’s exhalation during tidal breathing. Generally, only around 10% of the dose is deposited in the lung with the remainder either deposited in the upper airways or lost to the environment (Byron, Citation2004). Furthermore, not all the formulation is aerosolized, a residual volume is still present in the device at the end of the administration, requiring proper waste disposal and device cleaning. These latter characteristics can pose a significant occupational risk to health-care providers and family members (Darwiche et al., Citation2013).
Some new nebulizer devices however offer some interesting solutions to those issues. Most recently, new inlets for aerosol inhalation with breath-controlled valves have been introduced in the market, while several nebulizer manufacturers (e.g. Pari, Aerogen) have introduced vibrating mesh technology devices that greatly reduced the time taken to generate and deposit a given volume of formulation, minimizing drug losses due to patient exhalation. Most recently, some of these devices have become computer-controlled systems able to monitor each patient’s breathing pattern and administer aerosol only in phase with inspiration (I-neb, Adaptive Aerosol Delivery System, Philips) (Byron, Citation2004; Zarogoulidis et al., Citation2012a).
SMIs circumvent this problem by being trigger or breath actuated, dosing a small amount of aqueous formulation and having only a short duration of nebulization. In this way, only what the patient can inhale is delivered with each breath and almost none of the dose is lost as an aerosol to the environment (Geller, Citation2002).
Nanocarrier formulations administered as liquid aerosols
The most investigated nanocarriers for lung delivery are by far liposomes. Liposomes are spherical closed lipid vesicles formed by phospholipids in water. Phospholipids self-organize themselves in one or several generally concentric bilayers with an aqueous phase inside and between the layers (Torchilin, Citation2005). Due to their distinctive structure, liposomes are attractive drug delivery vehicles for delivering hydrophilic, lipophilic, and/or amphiphilic drugs (). Moreover, being phospholipids natural endogenous compounds and one of the main constituent of alveolar surfactants, they appear especially suitable for pulmonary administration. Finally, liposomal formulations are already approved and clinically available both for systemic (Caelyx, Janssen Cilag, Beerse, Belgium; DaunoXome, Galen Ltd, Craigavon, UK; DepoCyte, Pacira Pharmaceuticals, Parsippany, NJ; Myocet, Enzon, Piscataway, NJ; Marqibo, Spectrum Pharmaceuticals, Henderson, NV; DepoDur, SkyePharma, Muttenz, Switzerland; AmBisome, Gilead, Foster City, CA; Visudyne, Novartis, Basel, Switzerland) and pulmonary administration (Alveofact®, Dr Karl Thomae GmbH, Germany) (Mansour et al., Citation2009; Chang & Yeh, Citation2012).
Figure 2. A. Liposomes loaded with water soluble (a) and water-insoluble drugs (b); B. Antibody-targeted immunoliposome with antibody covalently coupled (c) or hydrophobically anchored (d) to the surface; C. Liposome modified with a protective polymer such as PEG (e), which reduce interactions with opsonizing proteins (f); D. Liposome-bearing antibodies attached to the surface (g) or, to the end of PEG polymeric chain (h); E. Multifunctional liposome, which displays some of the following features: protective polymer (i), targeting ligand (j), diagnostic label (k), positively charged lipids (l) allowing for the complexation with DNA (m); stimuli-sensitive lipids (n); stimuli-sensitive polymer (o); cell-penetrating peptide (p) and incorporation of viral components (q), magnetic particles (r) for magnetic targeting and/or colloidal gold or silver particles (s) for electron microscopy.
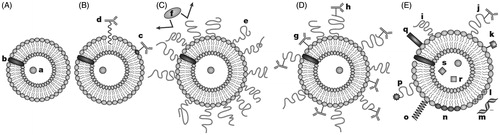
There are three main types of liposomes: multi-lamellar vesicles (MLV), large unilamellar vesicles (LUV), and small unilamellar vesicles (SUV) (Akbarzadeh et al., Citation2013; Zhang et al., Citation2013). MLV have an average diameter ranging from a few hundred nanometers to several micrometers and are composed of many concentric lipid bilayers. These are the most thermodynamically stable of the liposomes and are highly suited to encapsulation of lipophilic drugs, including the majority of anticancer drugs such as paclitaxel and docetaxel within its lipid bilayers. LUV and SUV have smaller diameters and have a single lipid bilayer with an aqueous core. LUV and SUV are particularly suited for encapsulating hydrophilic molecules inside the aqueous core, with larger payloads possible in the LUV (Willis et al., Citation2012). Additionally, it is also possible to obtain non-concentric structures, when multiple unilamellar vesicles may form inside of larger vesicles producing multivesicular vesicles (MVV) (Akbarzadeh et al., Citation2013).
Traditionally, liposomal formulation for inhalation has been delivered using nebulizers, however, recent studies have shown that liposome formulation can be used in powder form for administration with DPIs, further demonstrating the versatility of this drug delivery system.
A review of the potential applications of liposomal formulations for inhalation by Willis and colleagues discussed the benefits of liposomal drug encapsulation for loco-regional delivery. It was particularly highlighted that such formulations are able to produce similar efficacy with lower doses and reduced systemic side effects with the additional potential for local sustained release when compared to oral and intravenous formulations of the same drugs (Willis et al., Citation2012).
In fact, liposomes can be formulated to allow for a sustained release preparation, decreasing frequency of administration and increasing patient adherence. Surface modification of liposomes with polyethylene glycol (PEG) has been shown to increase the elimination half-life of drugs by minimizing the uptake of the drug by alveolar macrophages, further enhancing the drugs residence time at the site of action (Willis et al., Citation2012). Surface modification can be used also to improve bioadhesion or to molecularly target the vesicle toward cancer cells through antibody or ligand mediated recognition (Akbarzadeh et al., Citation2013).
Camptothecins are a class of potent anticancer agents that act by inhibiting topoisomerase I, a key enzyme for DNA replication and cell proliferation, overexpressed in malignant cells. A lipophilic derivative, 9-nitrocamptothecin (9NC), was shown to be more potent and to possess the highest activity/toxicity ratio in comparison to the parent compound and hydrophilic derivatives, however its use with parenteral administration is severely hindered by poor aqueous solubility and serum protein binding. As a consequence, a liposomal formulation of 9NC for pulmonary administration has been developed and tested in preclinical and clinical studies (Koshkina et al., Citation2003). See for a summary of the clinical studies investigating the delivery of anticancer nanoformulations via inhalation.
Table 1. Clinical trials of anticancer drugs nanoformulations administered via inhalation.
Dilauroylphosphatidylcholine (DLPC) liposomes were loaded with 9-nitrocamptothecin (9NC-DLPC) by freeze-drying of a butanol/DMSO solution of drug and phospholipids followed by extemporaneous re-dispersion with water for injection. The selected liposomal suspension, containing 0.5 mg 9NC/ml, showed particle size in the range of 100–400 nm, drug encapsulation higher than 80% and mass median aerodynamic diameter (MMAD) of 1.2 µm when the liposomal formulation was aerosolized with an air jet nebulizer (Aerotech II, CIS-US, Bedford, MA). Preliminary studies were performed in mice using the subcutaneous human lung tumor xenograft and human osteosarcoma pulmonary metastasis models. The first experiment showed a significant reduction in tumor volume in animals treated with aerosolized 9NC-DLPC, even compared to groups of animals receiving the same dose orally or intramuscularly (Knight et al., Citation1999). In the metastases model, the aerosolized formulation of the camptothecin derivative showed the capacity to reduce the number of animals with disease and the number and size of lung nodules in the fraction of animals showing pulmonary metastases (Koshkina et al., Citation2000).
A phase I clinical trial with the proposed formulation was carried out in patients with primary or metastatic diseases in the lung, non-responsive to standard treatments. Six patients were enrolled and received 6.7 µg 9NC/kg by aerosolization daily for 5 consecutive days every 3 weeks, if disease remained stable. This feasibility study showed that aerosol delivery of liposomal 9-NC was well tolerated with no side effects higher than grade 2 and 9-NC was absorbed systemically showing a plasmatic concentration peak 2 h post-administration. Disease stabilization was achieved in two patients (Verschraegen et al., Citation2004).
A further study was focused on safety of the aerosolized treatment and to recommend a dosage for a Phase II trial with an 8-week daily treatment schedule. In this study, 25 patients were treated 5 d a week for up to 8 consecutive weeks followed by 2 weeks of rest. Drug doses were increased stepwise from 6.7 µg/kg/day up to 26.6 µg/kg/day. Remarkably low hematological toxicity was evidenced in this study, considering that anemia and neutropenia are the main toxic effect of 9NC when administered orally. Dose-limiting toxicities were chemical pharyngitis and general fatigue with muscular aches for the 26.6 µg/kg/day and 20 µg/kg/day dosages, respectively. Respiratory functions, such as forced expiratory volume in 1 s (FEV1), forced vital capacity, total lung capacity, and diffusing capacity for carbon monoxide (DLCO) were monitored and showed a clinically acceptable decrease during the therapy, but values recovered pre-treatment baseline during the rest period. As a consequence, the recommended dose for Phase II studies was 13.3 µg/kg/day on a daily 60-min exposure, 5 consecutive days/week for 8 weeks. Partial remissions were observed in two patients with endometrial cancer, and stabilization occurred in three patients with primary lung cancer (Verschraegen et al., Citation2004).
Another drug studied clinically in form of liposomal formulation for administration by inhalation has been an IL2. The IL2 is a cytokine with proven antitumor effect mediated by the activation and proliferation of immune cells, such as T lymphocytes, natural killer cells, and macrophages. However, IL2 when administered parenterally shows a narrow therapeutic index and dose-dependent debilitating toxicities, including fever, chills, fatigue, vascular leak syndrome, anemia, and thrombocytopenia. A different administration route and a liposomal formulation are able to change the drug pharmacodynamics, modifying its release and distribution as well as promoting mononuclear phagocyte uptake and lymphatic absorption. MLV of dimyristoylphosphatidylcholine loaded with IL2 (IL2-DMPC) were produced by hydration of the phospholipids with a dispersion of the protein in saline followed by three freeze/thaw steps, necessary to obtain over 95% drug incorporation (Anderson et al., Citation1992). The liposome formulation could be aerosolized using a Twin Jet Nebulizer (Puritan Bennet Corp., USA) obtaining droplets with a MMAD of 2.0 µm. Animal studies were carried out in dog having pulmonary metastases or primary lung tumors to show safety and efficacy of the treatment. Minimal toxicity was evidenced in dogs with administration of inhaled IL-2 liposomes twice or three times daily for 30 d. Of the nine dogs treated, two dogs with metastatic pulmonary osteosarcoma showed complete metastasis regression and one dog with lung carcinoma had long-term stabilization of disease (Khanna et al., Citation1997). A Phase I clinical trial designed to asses the feasibility and toxicity of IL2 liposomes administration by aerosol to patients with pulmonary metastases was conducted at the Mayo Clinic (Rochester, NY). Patients inhaled for about 20 min 1.5, 3.0, or 6.0 × 106 IU of IL2 in form of liposomes three times a day. No significant toxicity was evidenced throughout the study, with respiratory parameters not significantly modified, except FEV1 decreased after 28 d of treatment, but the reduction was of no clinical relevance. Of the nine patients treated, one patient with melanoma eventually developed a complete remission post-treatment, two patients with sarcoma had a stable disease as well as one patient with renal cell carcinoma (Skubitz & Anderson, Citation2000). Despite these interesting results obtained at the dawn of the millennium, it seems that liposomes as drug delivery vehicle for anticancer drugs lost their appeal for some time, possibly as a consequences of a number of concerns related to the safety of the administration procedure or to the effect of the nebulization on the formulations; in fact, the shear forces necessary for the aerosolization process are able to tremendously affect liposomes size and integrity.
More recent studies have seen the investigation of liposomal formulations of cisplatin. Cisplatin is one of the most active anticancer agents in a number of malignancies; however, it shows a number of dose-limiting toxicities, i.e. nephrotoxicity, peripheral neuropathy, ototoxicity, nausea, and vomiting. A Sustained Release Lipid Inhalation Targeting (SLIT) cisplatin was developed to overcome these issues and underwent distribution, toxicity, and efficacy studies in rats, dogs, and mice (Lewis lung metastasis model), showing that the treatment was well tolerated and provided antitumor activity with low systemic exposure. The formulation produced by Transave Inc. (Monmouth Junction, NJ) consisted in liposomes composed of dipalmitoylphosphatidylcholine (DPPC), cholesterol, and 1 mg/ml of cisplatin. SPLIT cisplatin formulation upon aerosolization with a PARI LC Star nebulizer showed a MMAD of 3.7 µm, and released immediately 40–50% of total cisplatin, while the rest showed a sustained release.
A Phase I clinical trial was conducted to evaluate safety profile and pharmacokinetic of SLIT cisplatin in patients with primary or metastatic lung cancer. A number of preventive measure were put in place to limit the unwanted exposure of health-care professionals and patients to the cytotoxic drugs, i.e. full barrier personal protective equipment, a demistifier tent isolating the patient during treatment and filters collecting the exhaled aerosols in the nebulizer (Wittgen et al., Citation2006). Patients received SLIT cisplatin by inhalation during 1–4 d in cycles of 21 d, doses based on body surface area were escalated from 1.5 to 60 mg/m2. No dose-limiting toxicity was observer in the 17 patients enrolled in the study, most of the side effects were related to respiratory systems with dyspnea, cough, and hoarseness observed more frequently. Decrease in FEV1 was reversible in all cases but one, a patient with the chemical bronchitis. Typical systemic toxicities were not observed. Monitored respiratory parameters, FEV1 and DLCO, decreased during treatment but effects were reversible in all but one case. Twelve patients had stable disease and four had progressive disease; no complete or partial responses were observed (Wittgen et al., Citation2007). This study was followed by a Phase Ib/IIa, 3-center, open label study in patients with recurrent/progressive osteosarcoma with lung metastases. Two dose levels were administered (24 and 36 mg/m2) on an every 2-week cycle. Three patients experienced severe adverse events requiring hospitalization, i.e. grade 3 vomiting, severe dyspnea with chest pain, and worsening of malignant pleural effusion. Out of 19 patients, only patients with lesions smaller than 2 cm or that underwent resection of the lung disease experienced a sustained benefit from the inhaled liposomal cisplatin formulation: two patients achieved a complete response after metastasectomy, one patient achieved a complete response after the inhalation treatment, and one had a sustained partial response, other patients discontinued the drug due to progression of disease (Chou et al., Citation2013).
These studies open the possibility to the development of new and more sophisticated formulations for other drugs such as paclitaxel. Paclitaxel, a poorly water-soluble antimitotic drug targeting cancer cell microtubules, was recently formulated in pulmonary surfactant mimetic, pH responsive liposomes. The formulation contained DPPC and dioleoylphosphoethanolamine (DOPE), an unsaturated phospholipid claimed to act as a surfactant similarly to the surfactant protein-B (SP-B), present in endogenous lung surfactant. Furthermore, DOPE acts as fusogenic lipid, able to release the drug at lower pH values present at the tumor environment (pH 6.5–7) or inside cytosolic endosome/lysosome organelles (pH 4–6). Liposomes prepared by thin film hydration method showed small particle size (115 nm), negative zeta potential (−30 mV), narrow distribution, high encapsulation efficiency, and drug loading (80% and 30%, respectively). The formulation was administered using an air jet nebulizer (Micelfluss F400, Flaem Nuova, Italy) to mice with pulmonary metastases of murine melanoma (B16F10). The aerosol treatment (10 ml of 0.5 mg/ml paclitaxel liposomal formulation for 5 consecutive days every week) was compared to intravenous treatment with two commercial formulations of the drug Taxol® and Abraxane® (10 mg/kg once every 3 d). The aerosol treatment showed no major pulmonary toxicities such as fibrosis and chronic inflammation and produced a significant reduction in the number of metastases and lung surface occupied by the lesions, with an overall metastases inhibition of nearly 75%, superior to the parenteral treatment with both commercial product (around 50% inhibition for both treatments) (Joshi et al., Citation2014).
A recent study, compared the lung retention of liposomes and of a number of nanocarriers and nanomaterials administered intravenously and by pulmonary administration, i.e. micelles, mesoporous silica nanoparticles (MSNs), polypropyleneimine (PPI) dendrimer–small-interfering RNA (siRNA) complex nanoparticles, quantum dots (QDs), and PEG polymers. It was highlighted how the main differences in lung uptake were found after the inhalation delivery of lipid and non-lipid-based nanoparticles, i.e. the lung accumulation of liposomes and micelles in lungs remained relatively high even 24 h after inhalation whereas other nanoparticles mainly accumulated in the liver, kidneys, and spleen. Inhalation delivery of doxorubicin by liposomes (600 nm, 14 µg DOX/kg) significantly enhanced its anticancer effect and prevented severe adverse side effects of the parenteral treatment in mice bearing an orthotopic model of lung cancer, further demonstrating how the advantage of inhaled nanoformulations resides in the local administration coupled with specific organ retention and control of drug release (Garbuzenko et al., Citation2014a).
Several non-liposomal nebulized nanocarriers for loco-regional delivery of chemotherapeutic drugs have been recently proposed but they are still at preclinical development level. Gill and co-authors developed paclitaxel-loaded poly(ethylene oxide)-block-distearoylphosphatidylethanolamine (PEG-DSPE) micelles, focusing mainly on the pharmacokinetic aspects of the drug after loco-regional administration in rats (Gill et al., Citation2011). Polymeric micelles are particles with diameters typically smaller than 100 nm, formed by the self-assembling of amphiphilic polymers dispersed in aqueous media used for the formulation of drugs with low water solubility (Lukyanov & Torchilin, Citation2004). Paclitaxel-loaded PEG-DSPE micelles were obtained by solvent evaporation and thin film hydration and showed very small particle size, around 5 nm and 95% paclitaxel incorporation (1 mg/ml, 1:40 drug/polymer ratio). The micelles were administered intratracheally and confronted with an intravenous administration of the same formulation or an intratracheal instillation of Taxol® commercial formulation. It was found that the pulmonary administration of the micelle formulation led to decreased systemic distribution and increased accumulation of paclitaxel in the lung, also demonstrated by reduced systemic toxicity. A significant sustained release profile for micelles was evidenced in in vitro studies with only 22% of the loaded paclitaxel released in the first hour into simulated lung fluid containing 0.02% Infasurf®. This explained why the paclitaxel lung concentration after intratracheal administration of micelles continued to rise, leading to a 39 times larger paclitaxel concentration in the lung at 12 h compared to Taxol administered in the same way (Gill et al., Citation2011).
Liposome and micelles along with a number of desirable properties present some problems related to their peculiar structures, such as limited drug loading, drug leaking upon storage, and instability in biological environment. Since 1990s, nanoparticles composed of lipids that are solid at room temperature (solid lipid nanoparticles, SLN; nanostructured lipid carriers, NLC) have been developed as an alternative to both liposomes and polymer nanoparticles, conjugating the use of endogenous and/or highly biocompatible lipids typical of the former with higher payloads, improved stability, and prolonged release characteristic of the latter (Badrzadeh et al., Citation2015). Interestingly, these delivery systems have been proposed for the formulation of drugs that are not established anticancer agents adding further interest to the eventual product development for lung cancer.
Cyclooxygenase-2 enzyme has elevated expression in various human cancers and is considered to have a role in the development of non-small cell lung cancer. Celecoxib (CXB), a highly selective COX-2 inhibitor has been proposed alone or in combination to treat lung cancer. However, to aerosolize this lipophilic drug a co-solvent and surfactants are necessary in order to increase water solubility and the resulting formulation lacks long-term stability. In order to overcome these issues, CXB was encapsulated in the nanostructured lipid carrier (CXB-NLC) nanoparticles prepared with Compritol, Miglyol, and sodium taurocholate using a high pressure homogenizer (Emulsiflex-C5, Avestin, Canada). The mean particle size was around 220 nm and drug loading 4% (1.8 mg/ml). The formulation was nebulized using a Pari LC star jet nebulizer, showing a fine particle fraction (FPF, dae < 5 µm) of 75% and a MMAD of 1.6 μm. In mice, CXB-NLC delivered by aerosol demonstrated four-fold higher bioavailability in lungs compared to the drug solution, as a result of slower clearance (Patlolla et al., Citation2010).
In another study, Videira and colleagues showed reduced systemic toxicity and even showed a complete remission of tumors, in mice with a lung metastasis model, using nebulized SLN composed of glycerol palmitoyl stearate and polysorbate 80 loaded with paclitaxel (PTX-SLN). The 100 nm nanoparticles eliminated completely the lung disease in a prolonged treatment group: eight pulmonary administrations PTX-SLN 1 mg/kg twice weekly. This result outdid previously reported anti-metastasis efficacy reported in similar tumor models using aerosolized PTX liposomal formulations. The differences evidenced were explained with a possibly improved cell internalization and drug release in the intracellular space for the SLN, responsible also for a very low toxicity profile (Videira et al., Citation2012).
The versatility of lipid nanoparticles is demonstrated by a nanomedicine platform suitable for inhalation developed by the group of Tamara Minko at Rutgers able to provide: local delivery of anticancer drugs (paclitaxel or doxorubicin), suppression of resistance with siRNA, targeting cancer cells using an analogue of luteinizing hormone-releasing hormone (LHRH) as a targeting moiety. NLC were prepared by a melting and ultrasonic dispersion method, using Precirol ATO 5 as solid lipid, squalene as liquid lipid component, soybean lecithin, Tween-80, and the cationic lipid dioleoyltrimethylammonium propane (DOTAP) as surfactants; DSPE–PEG–COOH was added to preparation to provide increased steric stabilization, avoidance of particle uptake by reticulo-endothelial system cells and anchoring of the target moiety (). The nanoparticles had mean particle size of 110 nm, 5% drug loading both with doxorubicin and were physically stable for at least 30 d at 4 °C. When delivered to mice bearing an orthotopic lung tumor using a Collison nebulizer, it was evidenced a preferential accumulation in tumor tissue was obtained with targeted particles, while a uniform distribution in lungs was the result of the administration of non-targeted particles. Antitumor activity experiments showed that enhancement of antitumor activity compared to free paclitaxel was obtained with paclitaxel-targeted particles, but tumor almost complete regression was obtained only when siRNA silencing proteins related to efflux and anti-apoptotic defense mechanisms was co-delivered with the multifunctional nanoparticles (Taratula et al., Citation2013).
Figure 3. Nanostructured lipid carrier platform able to provide pulmonary co-delivery of an anticancer drug, siRNA and targeting of cancer cells with a peptide. Reproduced from Taratula et al. (Citation2013) with permission.
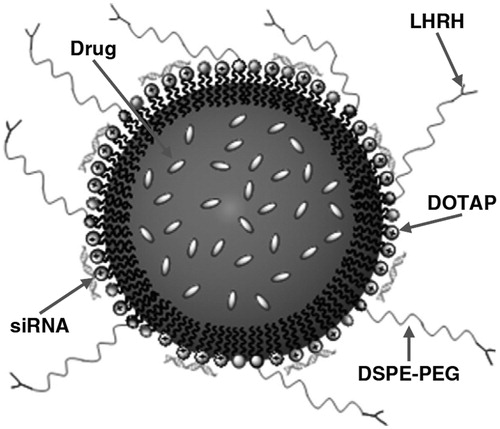
While uptake from phagocytic cells is generally considered an issue to avoid in order to prolong lung persistence of inhaled nanocarriers, but a study has suggested that alveolar macrophages could contribute to the anticancer efficacy of polymer nanoparticles. Polymer nanoparticles for pulmonary delivery are produced with biocompatible and biodegradable polymers, such as PLA, PLGA, gelatin, and albumin. In this study, poly(isobutyl cyanoacrylate) loaded with doxorubicin (DOX-PIBCA, 135 nm) prepared using an emulsion polymerization method was able to activate alveolar macrophages against cancer cells, in a way that was specific to nanoparticle treatment and not shown by naïve macrophages or macrophages treated with a drug solution. This macrophages-mediated antitumor efficacy was related to the release back of fragments of NPs that were previously phagocytized and production of a number of Th1-cytokines, including tumor necrosis factor alpha, and interferon gamma. A secondary cytotoxicity effect could be then obtained by having macrophages acting as nanoparticles reservoirs and cell-mediated immune response inducers at lymph nodes level (Al-Hallak et al., Citation2010).
The most investigated polymer nanocarriers for delivery by nebulization have been gelatin nanoparticles. Gelatin, a common pharmaceutical excipient with generally recognized as safe (GRAS) status, can be used to reliably obtain nanoparticles of around 120 nm with a two-step dehydration method followed by cross-linking with glutaraldehyde. For pulmonary delivery, the surface chemistry of the nanoparticle was modified by conjugation with NeutrAvidin-biotinylated epidermal growth factor (EGF) to facilitate EGFR-mediated endocytosis in non-small lung cancer cells. In a first study, this novel EGF targeted gelatin nanoparticles passively loaded with cisplatin, showed high levels of cytotoxicity in vitro with a high level of selectivity for cells over expressing EGFR and a sustained drug release profile attributed to the high degree of cross-linking and complex formation between gelatin and the drug. When nanoparticles were nebulized at a dose of 12 mg/kg (AP-100100, APEX Medical Corp., Taiwan) to treat mice with lung metastases, a significant reduction in tumor volume was obtained with cisplatin, non-targeted nanoparticles, and EGF-targeted nanoparticles. However, targeted particles were superior to other treatments both in antitumor efficacy and in reduction of nephrotoxicity, one of the most frequent and severe side effect of cisplatin (Tseng et al., Citation2009). The same targeted particles have been proposed recently with a slightly modified manufacturing procedure in order to load the anticancer drug doxorubicin and showed similar efficacy, in vitro and in vivo further highlighting the potential of the local inhalation delivery in combination with the targeting of cancer cells (Long et al., Citation2014).
A unique drug delivery system able to co-deliver at the same time as an hydrophobic and an hydrophilic anticancer drug has been proposed again by the group of Professor Minko for pulmonary delivery to treat lung cancer. The anisotropic biodegradable particles proposed, named Janus particles, have a peculiar shape with two clearly separated phases, i.e. a polymeric and a lipid one. Particles were produced with a water-in-oil-in-water multiple emulsion solvent evaporation method using PLGA and Precirol ATO 5 as main components and were loaded with doxorubicin and curcumin, as model hydrophilic and lipophilic anticancer drugs respectively. Two different types of nanoparticles produced had mean particle size of 150 and 450 nm, negative zeta potential, relatively narrow particle size distribution and could be nebulized (Collison nebulizer) without altering particle characteristics. Particles were demonstrated to lack of cyto- or genotoxic effects and the potential for being avidly internalized by cancer cells. In vivo distribution studies interestingly evidenced a better lung accumulation and prolonged retention of particles with higher particle size after inhalation in comparison to smaller ones. These larger particles loaded with doxorubicin and curcumin suppressed tumor growth in an orthotopic murine lung tumor model. The same results could not be achieved with particles loaded with only one of the two drugs, confirming synergistic action of the two drugs (Garbuzenko et al., Citation2014b).
Also inorganic nanoparticles have find application in pulmonary administration, mainly as diagnostic but more recently as materials for targeted drug delivery and theranostic applications. MSNs can be used for delivery of doxorubicin, cisplatin, siRNA and allow surface functionalization for targeting cancer cells (Taratula et al., Citation2011). Nebulized gadolinium-based nanoparticles have been demonstrated as imaging probe ad radiosensitizing agent in an orthotopic murine lung cancer model. These ultrasmall nanoparticles (<6 nm) allowed for tumor detection in animals by fluorescence tomography or ultrashort echo-time magnetic resonance imaging (UTE-MRI) and prolonged animals mean survival time after a single dose of dose conventional radiation (10 Gy) demonstrated their radiosensitizing effect (Dufort et al., Citation2015).
Superparamagnetic iron oxide nanoparticles (SPIONs) not only can claim a long-running use in clinical setting as contrast agents for MRI, but provide interesting solutions for aerosolization chemotherapy as well. In fact, their magnetic properties allow for the targeting of their aerosol in lung regions affected by disease using a strong magnetic field (Tewes et al., Citation2014) or for antitumor hyperthermia by application of an alternating magnetic field (Sonvico et al., Citation2005). For the first type of approach, SPIONs have been coated with PLGA and could be used to load a model anticancer drug such as quercetin. These core-shell particles of around 300 nm were biocompatible, well tolerated by mice after inhalation and when loaded with quercetin produced cancer cells growth inhibition, upon aerosolization over human lung carcinoma cell line A549 with a vibrating mesh nebulizer (Aeroneb® Pro, Aerogen, Ireland) (Verma et al., Citation2013).
Targeted hyperthermia for lung cancer was obtained using inhalable EGFR-targeted SPIONs. EGFR-targeted SPIOs nanoparticles (around 370 nm) were synthesized from ferric and ferrous chloride by the addition of ammonium hydroxide and subsequent coating with myristic acid and pluronic F127 conjugated with an EGFR-targeting peptide. Nanoparticles dispersions showed rapid heating rates when placed in alternating magnetic field (6 kA/m, 386 kHz). The nanoparticles were administered to mice carrying an orthotopic lung cancer model using an ultrasonic atomizer (MMAD 1.1 µm) and EGFR targeting induced enhanced tumor retention and resulted in significant reduction of lung tumor growth over controls, i.e. animals non-treated, treated with non-targeted nanoparticles, or treated with targeted or non-targeted SPIONs but not subjected to the magnetic hyperthermia treatment (Sadhukha et al., Citation2013).
While there have been a number of successful studies in nebulized nanoparticles for inhalation, there are some potential problems that may have to be dealt with before any of these products become available. Some major issues encountered include potential for nanoparticles to agglomerate in suspension, poor stability due to hydrolysis and degradation of drugs, potential to induce bronchoconstriction, poor deposition due to shallow tidal breathing and risks posed to those administering the dose (Mansour et al., Citation2009; Willis et al., Citation2012; Zarogoulidis et al., Citation2012a). While some solutions to such issues have been proposed, as the addition of 5–7% carbon dioxide in the aerosol to force the patient to breathe deeply and slowly increasing tidal volume by up to 180% (Koshkina et al., Citation2001), lyophilization of nanoparticles for resuspension, and development of breath actuated nebulizers to limit the environmental dispersion of the formulation (Mansour et al., Citation2009; Zarogoulidis et al., Citation2012b), a lot more work need to be done to make this a viable alternative and preferable option for clinical treatment of lung cancer.
Dry powder inhalers
DPIs may be able to overcome some of the potential misgivings about anticancer drug delivery by nebulization, in particular those related to poor stability and hazards to patients, carers, and the environment through release of aerosols. However, nanosized particles have a low lung deposition and are more likely to be exhaled. Moreover, as a result of their size and high specific surface, nanoparticles show high surface energy that results in non-redispersible aggregates when they are dried (Lebhardt et al., Citation2011). Nanoparticles have to be administered after incorporation into larger structures with appropriate size for pulmonary drug delivery. Few different methods have been employed to temporary shift nanoparticles to micron-sized particles to allow for efficient dosage, aerosolization, and deposition of the powder in the lung. Most importantly, once particles deposit on the pulmonary mucosa, nanoparticles should recover their primary size and perform their therapeutic action. Among different approaches, spray-dried powders containing nanoparticles and water soluble excipients have been the most developed and studied. Spray drying technique offer several advantages, such as the relatively straightforward production of particles with narrow size distribution and with desired properties in term of pulmonary administration. This overcomes constraints associated with other drying methods, such as freeze-drying: formation of hard cakes, need for further micronization, wide and/or heterogeneous particle size distributions, need for coarse carriers for dosage and aerosolization, and poor aerodynamic performance (Colombo et al., Citation2012).
Moreover, DPIs as pulmonary delivery device offer some advantages over other technologies: long-term stability of formulation, no need of no hand-lung inhalation coordination being generally breath-actuated inhalers, improved patient compliance, uniform deposition of high dose of drug locally, liquid and/or propellant free formulation and opportunity for patent protection.
Formulation strategy could overcome problems such as particle aggregation by reducing inter-particle attraction using techniques such as blending of nanoparticles with larger carrier particles like lactose, forming ordered mixtures thus enhancing product stability (Willis et al., Citation2012). Perhaps most notably, production of aerosols is not an issue in DPIs as aerosol production is breath actuated and hence, no aerosol is lost to the environment.
As evidenced in the section related to nebulizers, the use of liposomes in pulmonary administration has many potential advantages, including carrier suitability for most lipophilic drugs, aqueous compatibility, sustained release, and intracellular delivery. Despite the liposomal formulations for inhaled therapy have been classically delivered by nebulization, there has been a significant level of interest in developing those nanocarriers into dry powder formulations. In fact, in aqueous dispersions, liposomes show lipid degradation, sedimentation, aggregation, leakage of drugs, or fusion during storage, while aerosolization may result in chemical and physical instability. Their formulation into dry powder achieves long-term stability and allows for their administration with DPIs (Misra et al., Citation2009). A 2012 study developed a cationic liposome formulation by thin film hydration of DOTAP, DOPE, DPPC, and cholesterol for the simultaneous delivery of two anticancer drugs, i.e. etoposide and docetaxel, and plasmid DNA encoding the tumor suppressor gene p53 for a triple action chemotherapy to be delivered by dry powder inhalation. The powder was obtained by freeze-drying using trehalose as cryoprotectant (Jinturkar et al., Citation2012). This method of delivery of the drugs and the p53 gene was developed to reduce the incidence of tumor p53 inactivation mediated drug resistance, which is a common cause for treatment failure in all types of chemotherapy, by supplementing the endogenous p53 genes and inducing p53-mediated apoptosis (Lowe et al., Citation1994). The authors were able to demonstrate that the pDNA delivered was completely protected from degradation by enzymes such as DNAses by the lipoplex formed and observed a significant increase in cytotoxicity with the co-delivery of the two cytotoxic drugs along with the p53 gene with a 30% increase in cell death compared to free drug solution. Enhanced cytotoxicity was observed with pre-treatment with the liposomally encapsulated p53 as well, with an observed increase in sensitivity to the drugs by up to 60%. The dry powder formulation demonstrated a potential significant lung deposition using an Anderson Cascade Impactor and Rotahaler (Cipla, India) as a model capsule-based aerosolizing device with an average aerodynamic diameter of 3–4 µm and a fine particle fraction around 35% (Jinturkar et al., Citation2012).
Pactlitaxel-loaded lung surfactant mimetic PEGylated phospholipid micro/nanoparticles have been produced by organic solution co-spray drying of dilute feed methanol solution of the drug with DPPC and dipalmitoylphosphatidylethanolamine poly(ethylene glycol) (DPPE-PEG) for lung cancer drug delivery. The aerosolization performance of the powders was studied in vitro using the high-resistance capsule-based Handihaler® device (Boheringer Ingelheim, Germany) and the Next Generation Impactor (NGI™). The paclitaxel/phospholipid powders showed mass median aerodynamic diameters in the range of 3.4–6.8 µm and respirable fractions in the range of 44.0–54.5% (Meenach et al., Citation2013).
Despite the high versatility of liposomes as drug delivery devices, it seems from the available literature that, in the specific case of inhaled chemotherapy, nanoparticulate carriers have been preferred over phospholipid vesicles in the case of dry powder inhalation, possibly as a consequence of their peculiar structure more sensitive to the drying process compared to nanoparticles.
A straightforward approach proposed is the production of controlled dry assembly of nanoparticles with good aerosolizing properties. Paclitaxel nanoparticles were prepared by ultrasound-controlled precipitation in the presence of lecithin, polyvinylpyrrolidone, or cetyl alcohol as stabilizers and subsequently assembled in form of low-density microparticles using the amino acid leucine as colloid destabilizer and freeze-dried. The powders obtained not only provided faster dissolution rates compared to micronized paclitaxel but also showed small MMAD (<2 µm) with high fine particle fractions (>80%) when tested directly without a DPI device using a Tisch Ambient Cascade Impactor (El-Gendy & Berkland, Citation2009).
Another study focused on an anticancer drug nanoparticle-based dry powder inhalation therapy developed poly(butylcyanoacrylate) nanoparticles loaded with doxorubicin and coated with polysorbate 80 in order to enhance translocation across the alveolar capillary barrier. Nanoparticles were prepared by emulsion polymerization and then embedded in lactose to form the micron-sized carrier particles using a spray freeze-drying process. This formulation demonstrated reduced particle agglomeration, nanoparticles showed mean particle size around 170 nm after dissolution of the micron-sized carrier particles, and were stable for at least 6 months in storage (). The mean geometric particle size of these produced particles was 10 µm, but the aerodynamic particle size determined using an Andersen Cascade Impactor and a proprietary device was found to be much lower, i.e. 3.4 µm. Additionally, the authors were able to demonstrate significantly increased cytotoxic efficacy on H460 and A549 lung cancer cells at lower doses than those required for free doxorubicin. It was hypothesized that this may be partially attributed to uptake of drug by endocytosis, as dose-related cytotoxicity was observed also for blank nanoparticles. This observed formulation effect may be due to the presence of polysorbate 80 in the coating of the particles and would require further evaluation in term of safety for pulmonary administration (Azarmi et al., Citation2006).
Figure 4. Confocal microscopy image of doxorubicin-loaded nanoparticles embedded into lactose micron-sized particles via spray drying. Reproduced from Azarmi et al. (Citation2006) with permission.
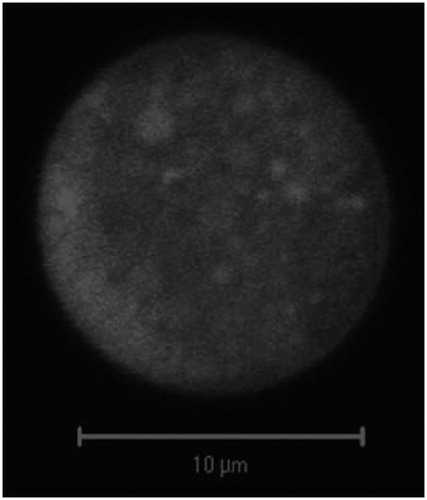
In another work in which PLGA nanoparticles loaded with the novel anticancer topoisomerase I and II inhibitor TAS-103 were prepared in the form of nanocomposite particles for dry powder lung administration. The nanocomposite particles, consisting of drug-loaded nanoparticles of 200 nm prepared by emulsion solvent evaporation and trehalose, were obtained by spray drying. The anticancer effects of the nanocomposite particles against the lung cancer cell line A549 were superior to the free drug. Nanocoposites showed a MMAD of 2.5 µm and FPF of 14% when tested with the capsule-based Jethaler device (Hitachi Ltd., Japan). In animal experiments, the nanocomposites provided an elevated lung concentration of the drug in the lungs up to 8 h, while plasma concentrations were find to be barely detectable, probably as a consequence of the rapid systemic metabolism (Tomoda et al., Citation2009).
Recently, a brominated derivate of an alkaloid of Papaver somniferum, 9-bromonoscapine (9-BN), has been found to have anticancer properties, due to a double action both on tubulin polymerization and on angiogenesis. The application of the drug is hindered by the high lipophilicity and poor aqueous solubility of the drug leading to suboptimal concentrations at the site of action. Lipid nanoparticles containing the drug (9-BN NLC) were prepared using the hot nanoemulsion method with stearic acid as main component and lecithin and sodium glycocholate as surfactant and co-surfactant. Nanoparticles presented particle size below 20 nm with a negative surface charge. Inhalable powders were obtained then by lyophilization in presence of lactose and with or without effervescence generating excipients, i.e. sodium carbonate and citric acid, in order to obtain powders able to exploit effervescence to rapidly release nanoparticles (9-BN NLC RR). Not only the 9-BN NLC RR formulation showed an improved release profile of the lipophilic drugs but were also superior to the other nanoparticulate formulation and the drug powder in pharmacokinetic studies, providing improved lung exposure to the drug and prolonged drug half-life (Jyoti et al., Citation2015).
The effectiveness of effervescent particles to improve nanoparticle dispersion has been successfully demonstrated by the group of Raimar Löbenberg at University of Alberta. Doxorubicin-loaded nanoparticles were prepared by polymerization of butylcyanoacrylate incorporated into inhalable effervescent and non-effervescent lactose micron-sized particles using spray freeze-drying. The final preparation contained nanoparticles of 140 nm providing a doxorubicin loading of 20 µg/mg of powder. Interestingly, without effervescence nanoparticle particles were not completely dispersed, showing a mean diameter close to 250 nm, while no significant difference was evidenced for the effervescent carrier powders in terms of nanoparticle diameters before and after re-dispersion. The doxorubicin-loaded powders were tested in a lung mice model. Animals treated with effervescent powders at a dose of 30 µg of doxorubicin once a week for 4 weeks showed a highly significant in survival compared to non-effervescent particles and intravenous treatments, with still 30% of mice alive at the end of the study after 140 d. Quite interestingly, in inhalable nanoparticle groups of animals, the cause of death was found to be the presence of large tumors in other parts of their body, but not in the lungs, further demonstrating nanoparticle impactful local anticancer activity. Furthermore, this treatment completely suppressed the cardiac toxicity observed in doxorubicin chemotherapy (Roa et al., Citation2011).
Considering the benefits such as increased stability and control over particle size as well as reduced complications such as pH- and osmolarity-induced bronchoconstriction as seen in nebulized formulations, DPIs seem to be a promising delivery system for loco-regional delivery of nano-encapsulated chemotherapeutic agents. However, as highlighted by a 2012 review of inhaled therapies by Zarogoulidis, a major drawback of dry powder therapies is that a large proportion of the dose is often retained within the device (Zarogoulidis et al., Citation2012a). Additionally, it is common for DPI devices to be misused leading to reduced efficacy (Dolovich et al., Citation2005). While methods to overcome such drawbacks have been explored such as modifying devices to accommodate different inspiratory flow rates, such as the Turbohaler (Labiris & Dolovich, Citation2003), dry powder platforms are in this moment not ready for the administration of chemotherapy to treat lung cancer as demonstrated by the lack of clinical trials using this approach. However, once this threshold will be crossed, the technology appears to have great potential for an anticancer treatment administration in the outpatient setting.
Intratumoral injection
While inhaled therapies look to be a promising move toward improved treatment of lung cancers, a major hindrance to their use is that the efficacy of deposition within the lung is limited by the size of the tumor. In situations of tumors larger than 5 cm where surgery is contraindicated, an alternative to surgical resection could be intratumoral injection. This particular loco-regional administration approach in addition to the benefits of minimizing systemic exposure may also address the issues of poor vascularization often observed in solid tumors, that inhibits penetration of the anticancer drug leading to low concentrations of drug within the tumor parenchyma (Kar et al., Citation2011; Jia et al., Citation2012). Some other important biological features of solid tumors that represent substantial barriers to drug absorption in inhaled administered therapies include the presence of altered interstitial properties, which may involve a stiffening of the extracellular matrix (ECM) and increased interstitial pressure (Holback & Yeo, Citation2011). Most drugs are only able to penetrate the periphery of the tumor and so the risk of metastases or relapse is high as not all malignant cells are able to be eliminated whereas intratumoral injection delivers the drug to the core of the tumor. It is important to note that this altered ECM is a particular issue for nanoparticles due to the sheer size of the systems, particularly in comparison to free drug (Holback & Yeo, Citation2011). There has been a considerable amount of work done looking at intratumoral delivery of free anticancer drugs such as docetaxel (Yoo et al., Citation2010), cisplatin (Celikoglu et al., Citation2006a,Citationb), and paclitaxel (Wang & Chen, Citation2012). Celikoglu group has produced some comprehensive reviews of the delivery of cytotoxic drugs by intratumoral injection, particularly via bronchoscopy and there appears to be a considerable opportunity to achieve even more in this treatment modality (Celikoglu et al., Citation2008, Citation2010). As discussed in these reviews, the mainstay of treatment for inoperable tumors in the lung has been external beam radiation; however, it is becoming increasingly obvious that this mode of treatment is insufficient as these patients continue to have and extremely poor prognosis. In a 2010 study (Yoo et al., Citation2010), p53 viral vectors were co-delivered with docetaxel to tumors in mice and produced an impressive and significant reduction in tumor size in treated animals compared to the control group and almost-complete tumor suppression was even observed. In this study, the animals had to receive biweekly intratumoral injections, as the drug cannot be retained within the tumor, which appears a significant drawback of almost all of the studies looking into intratumoral injection of solutions of free drug. In this context, there appears to be an opportunity for a variety of nanosystems to be administered via this delivery route in order to obtain a sustained delivery effect. Nevertheless, little research has occurred specifically looking at the role of nanoparticles in intratumoral injections. Kang and coworkers open the way to sustained release intratumoral delivery using an in situ-forming gel based on doxorubicin-poly(ethylene glycol)-β-caprolactone diblock copolymer (Dox-MP), which was able to serve as a depot for the sustained release of doxorubicin. They were able to show a sustained release of doxorubicin over a period of 20 d following an initial burst phase over the first 2 d. The implication of this was a 10.5 times reduction in tumor volume in an animal model compared to control groups after a single administration of Dox-MP. This reduction in tumor volume was similar to the reduction observed for repeated (every 5 d) injection of free doxorubicin. The invasive nature of intratumoral injections can be reduced through the application of sustained release systems and it can be considered that a combination of the in situ gel with other nanoparticle delivery systems discussed herein has considerable potential to increase their efficacy and further improves the sustained release profile, minimizing patient compliance issues, and improving safety profiles.
Despite intratumoral injection of nanoparticles loaded with chemotherapeutic agents has not been a keen area of research until now, there have been a few groups who seem to have made some important headway.
One of these groups investigated vault nanocapsule technology for the delivery of cytokines, specifically CCL21, for lung cancer immunotherapy. Vaults are endogenous ribonucleoprotein ovoid particles with the size of 40–70 nm, as such they are ideal carriers for the delivery of therapeutic peptide and proteins. Proteins can be packaged inside vaults particles by fusion with a 162 amino acids vault-targeting domain. The immune potentiating CCL21 cytokine binds to CCR7 receptors and acts as a chemoattractant to a number of immune cells including naïve and memory T cells as well as natural killer T cells to induce non-p53-mediated apoptosis (Kar et al., Citation2011). The same group had previously looked at delivery of this protein in its free form via intratumoral injection and succeeded in reduce tumor size in murine lung cancer models, but discovered that very high doses and frequent administration were required in order to overcome the rapid clearance of the drug from the tumors after injection (Sharma et al., Citation2000). They have also recently taken this protein to Phase I clinical trials using a CCL21 gene-modified dendritic cell but found the method to be prohibitively expensive and its production to be overly time consuming (Baratelli et al., Citation2008). As a result, they developed for this cytokine a delivery system based on vault nanocapsules. They found that a single trans-thoracic injection of the recombinant CCL21-vault nanocapsules was able to elicit a significant tumor regression as a consequence of the recruitment of antitumor effector cells such as T lymphocytes and dendritic cells in a murine Lewis lung carcinoma model (Kar et al., Citation2011). Immune evasion is a major contributing factor to the growth of tumors, immune stimulants such as this could be used in conjunction with other cytotoxic therapies in order to further enhance the therapeutic effect (Hanahan & Weinberg, Citation2011).
Other recent research has focused on intratumoral injection of magnetic nanoparticles in the treatment of lung cancer. One group developed an intratumoral injection that consisted of magnetic iron oxide (Fe3O4) nanoparticles and doxorubicin co-encapsulated in PLGA nanocarriers. Oleic acid-coated Fe3O4 nanoparticles (4–6 nm) and doxorubicin were encapsulated in PLGA nanoparticles (200–300 nm) by the conventional oil-in-water emulsion evaporation method. The nanoparticles were injected directly into the core of the tumor in murine Lewis lung carcinoma model. The nanoparticles were held in place by an external magnetic field, which allowed release of the drug in a controlled manner and prevented loss of the nanoparticles to systemic circulation. The magnetic field was created using a disk magnet with a magnetic field strength of 0.5 Tesla. This was fixed to the skin just above the tumor and held in place for 48 h after injection. It was possible to observe a dramatic decrease in tumor size in the group of animals treated with magnetic nanoparticles, compared to control groups treated without magnetic field application and free drug respectively. Furthermore, no signs of systemic toxicity, such as weight loss, were observed in the doxorubicin magnetic nanoparticle group (Jia et al., Citation2012).
Araya and colleagues developed a method of inductive hyperthermia for solid lung tumors via intratumoral injection of magnetic nanoparticles. In order to obtain hyperthermia a new material was used: ferucarbotran, which is composed of dextran and ultrafine (7 nm) magnetite nanoparticles. The ferucarbotran was injected as a single dose to the core of the tumor, this was immediately followed by the application of an external alternating magnetic field to increase the intratumoral temperature to 43–45 °C. A significant reduction in tumor volume was obtained for treated animals compared to the control group over the 28 d of the study. A major drawback of the design of the study identified was the application of the external magnetic field which has required to be set 2 cm from the center of the tumor, which may be applicable in mice but will not be in any tumor that is not superficial in humans (Araya et al., Citation2013). However, considering the results of these two studies, intratumorally delivered magnetic nano-therapies seem worth of further studies in order to explore their potential role in the future of lung cancer therapy, especially considering that hyperthermia treatment has recently been made clinically feasible (Thiesen & Jordan, Citation2008).
Other approaches for local delivery of anticancer drugs
Beyond the abovementioned loco-regional approaches, there has been little investigation in the direction of developing novel strategies for the administration of nanopharmaceuticals for loco-regional treatment of lung cancer. Nonetheless, a number of other loco-regional platforms, that have been investigated recently for the treatment of cancerous lesions not localized to the lung, should be cited here, considering they have the potential to be employed in future for nanopharmaceuticals application in lung cancers.
One particularly successful loco-regional approach has been the development of wafers for implantation into the surgical cavity during surgery for high grade gliomas (Westphal et al., Citation2006). Gliadel® wafers are a commercially available delivery platform for carmustine and are used as an adjunct therapy for high grade glioma (Wolinsky et al., Citation2012). A 2011 systematic review describes clinical use of this treatment modality and assesses its effectiveness (Hart et al., Citation2011). Further study of similar treatment modalities may have a future role in the loco-regional treatment of lung cancers. An interesting evolution of the classic implant systems loaded with anticancer drugs are for example polymer films (Liu et al., Citation2010) and composite materials obtained by electrospinning (Yohe et al., Citation2012a,Citationb). These delivery platforms may have significant application in future lung cancer therapies, particularly in combination with nanopharmaceuticals.
An example of this combination has been proposed by a Chinese research group of the Binzhou Medical University that incorporated docetaxel-loaded carbon nanotubes (DOX-CNT) into a composite nanofiber scaffold of PLGA by electrospinning (). Nanofibers produced were uniform and smooth with width in the order of 500–800 nm and the incorporation of DOX-CNT contributed to provide a sustained the release, with 50% doxorubicin released in 15 d, obtaining a significant antitumor efficacy in vitro is obtained against HeLa cells. Quite importantly the good mechanical properties of these nanofiber mats make them useful as therapeutic patch for local anticancer drug delivery in surgical settings (Yu et al., Citation2015).
Figure 5. Schematic illustration for the process of fabrication of electrospun composite nanofibers containing doxorubicin-loaded carbon nanotubes. Reproduced from Yu et al. (Citation2015) under CC BY 4.0.
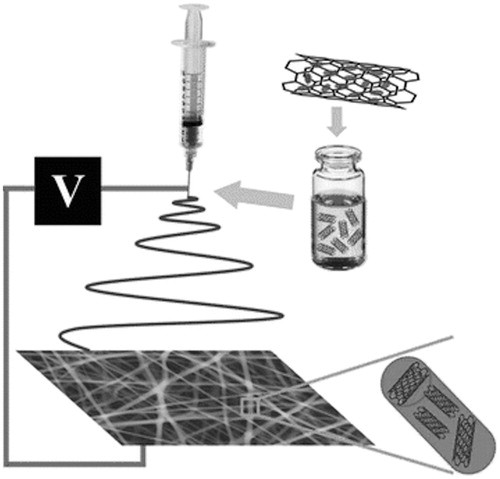
This delivery strategy could have significant application in the treatment of lung cancers, particularly those with diffuse tumors and a poor prognosis after surgical resection to treat cancers such as mesothelioma. Such a film could be implanted intrapleurally during surgical resection or debulking of tumors to provide a sustained, directed chemotherapy to prevent local recurrence. The benefits of such a formulation stem mainly from its capacity to further spatially localize and control drug release rates beyond the design of the nanoparticle.
Conclusion
Loco-regional delivery of anticancer drugs for the treatment of lung cancer is an expanding area of research and has the capacity to address many of the disadvantages of classical systemic chemotherapy including low drug accumulation within tumor parenchyma, poor aqueous solubility of chemotherapeutic agents, unacceptable high levels of systemic toxicity and high rates of tumor relapse. It appears that the combination of nanotechnology and loco-regional administration could be the most effective strategy to overcome these major drawbacks. Most recent investigation combining these two approaches have centered around inhaled therapies due to the ease of access to the tumor primary or metastatic site and non-invasive nature of the treatment modality. However, this approach is subject to the capacity of the particles to reach the site of action within the lung which may be affected by the size of the carrier particles, the size of solid tumors, altered airflow patterns in the lungs, and pulmonary co-morbidities. Production of cytotoxic aerosols is also a safety concern with this administration route; however, this is being addressed by the development of new nebulizing devices and dry powder formulations.
Beyond inhaled therapies there have been a number of successful studies investigating the potential for intratumoral injection of anticancer agent-loaded nanoparticles, especially magnetic nanoparticles. This approach may be of benefit to patients suffering inoperable lung cancer where the size of the tumor makes inhaled therapies inappropriate. This modality circumvents absorption concerns by delivering the drug to the core of the tumor. Significant reductions in tumor volume have been observed with this approach and combination of this delivery mode with nanotechnology has the potential to reduce dosing frequency, however, the invasive nature of the treatment may limit its future application.
Direct application of devices for sustained release of nanoencapsulated anticancer therapies has vast potential in the loco-regional treatment of lung cancer immediately subsequent to surgical resection of tumors. Currently, there are no data from in vivo studies of this application for lung cancer, however, considering the successes of clinical devices such as Gliadel® wafers and favorable results from devices for the delivery of nanoparticles in vitro it can be expected that this will be a keen area of research in future.
Declaration of interest
The authors report no conflicts of interest. The authors alone are responsible for the content and writing of the paper.
References
- Akbarzadeh A, Rezaei-Sadabady R, Davaran S, et al. (2013). Liposome: classification, preparation, and applications. Nanoscale Res Lett 8:102–10
- Al-Hallak KM, Azarmi S, Anwar-Mohamed A, et al. (2010). Secondary cytotoxicity mediated by alveolar macrophages: a contribution to the total efficacy of nanoparticles in lung cancer therapy? Eur J Pharm Biopharm 76:112–19
- Anderson PM, Hasz D, Dickrell L, Sencer S. (1992). Interleukin-2 in liposomes: increased venous potency and less pulmonary toxicity in the rat. Drug Dev Res 27:15–31
- Araya T, Kasagara K, Nishikawa S, et al. (2013). Antitumor effects of inductive hyperthermia using magnetic ferucarbotran. OncoTargets Ther 6:237–42
- Australian Bureau of Statistics (ABS). (2006). Cancer in Australia: a snapshot, 2004-05. Available at: http://www.abs.gov.au/ausstats/[email protected]/mf/4822.0.55.001 [last accessed 31 Aug 2015]
- Australian Bureau of Statistics (ABS). (2015). Causes of death, Australia, 2013. Available at: http://www.abs.gov.au/AUSSTATS/[email protected]/mf/3303.0/ [last accessed 31 Aug 2015]
- Azarmi S, Tao X, Chen H, et al. (2006). Formulation and cytotoxicity of doxorubicin nanoparticles carried by dry powder aerosol particles. Int J Pharm 319:155–61
- Badrzadeh F, Rahmati-Yamchi M, Badrzadeh K, et al. (2015). Drug delivery and nanodetection in lung cancer. Artif Cells Nanomed Biotechnol. [Epub ahead of print]. doi: 10.3109/21691401.2014.975237
- Baratelli F, Takedatsu H, Hazra S, et al. (2008). Pre-clinical characterization of GMP grade CCL21-gene modified dendritic cells for application in a phase I trial in Non-Small Cell Lung Cancer. J Transl Med 6:38
- Blanco E, Hsiao A, Mann AP, et al. (2011). Nanomedicine in cancer therapy: innovative trends and prospects. Cancer Sci 102:1247–52
- Byron PR. (2004). Drug delivery devices: issues in drug development. Proc Am Thorac Soc 1:321–8
- Carvalho TC, Carvalho SR, McConville JT. (2011). Formulations for pulmonary administration of anticancer agents to treat lung malignancies. J Aerosol Med Pulm Drug Deliv 24:61–80
- Celikoglu F, Celikoglu SI, York AM, Goldberg EP. (2006a). Intratumoral administration of cisplatin through a bronchoscope followed by irradiation for treatment of inoperable non-small cell obstructive lung cancer. Lung Cancer 51:225–36
- Celikoglu F, Celikoglu SI, Goldberg EP. (2008). Bronchoscopic intratumoral chemotherapy of lung cancer. Lung Cancer 61:1–12
- Celikoglu F, Celikoglu SI, Goldberg EP. (2010). Intratumoral chemotherapy of lung cancer for diagnosis and draining of lymph node metastases. J Pharm Pharmacol 62:287–93
- Celikoglu SI, Celikoglu F, Goldberg EP. (2006b). Endobronchial intratumoral chemotherapy (EITC) followed by surgery in early non-small cell lung cancer with polyploid growth causing erroneous impression of advanced disease. Lung Cancer 54:339–46
- Chang HI, Yeh MK. (2012). Clinical development of liposome-based drugs: formulation, characterization, and therapeutic efficacy. Int J Nanomedicine 7:49–60
- Chou AJ, Gupta R, Bell MD, et al. (2013). Inhaled lipid cisplatin (ILC) in the treatment of patients with relapsed/progressive osteosarcoma metastatic to the lung. Pediatr Blood Cancer 60:580–6
- Colombo P, Sonvico F, Buttini F. (2012). Nanostructures overcoming the pulmonary barrier: drug delivery strategies. In Alonso MJ, Csaba NS, eds. Nanostructured biomaterials for overcoming biological barriers. London: Royal Society of Chemistry, 273–300
- Dailey LA, Kleemann E, Wittmar M, et al. (2003). Surfactant-free, biodegradable nanoparticles for aerosol therapy based on the branched polyesters, DEAPA-PVAL-g-PLGA. Pharm Res 20:2011–20
- Dailey LA, Jekel N, Fink L, et al. (2006). Investigation of the proinflammatory potential of biodegradable nanoparticle drug delivery systems in the lung. Toxicol Appl Pharmacol 215:100–8
- Darwiche K, Zarogoulidis P, Karamanos NK, et al. (2013). Efficacy versus safety concerns for aerosol chemotherapy in non-small-cell lung cancer: a future dilemma for micro-oncology. Future Oncol 9:505–25
- De Souza R, Zahedi P, Allen CJ, Piquette-Miller M. (2010). Polymeric drug delivery systems for localized cancer chemotherapy. Drug Deliv 17:365–75
- Dolovich MB, Ahrens RC, Hess DR, et al. (2005). Device selection and outcomes of aerosol therapy: evidence-based guidelines. Chest 127:335–71
- Dufort S, Bianchi A, Henry M, et al. (2015). Nebulized gadolinium-based nanoparticles: a theranostic approach for lung tumor imaging and radiosensitization. Small 11:215–21
- El-Gendy N, Berkland C. (2009). Combination chemotherapeutic dry powder aerosols via controlled nanoparticle agglomeration. Pharm Res 26:1752–63
- Feng R, Zhang Z, Li Z, Huang G. (2014). Preparation and in vitro evaluation of etoposide-loaded PLGA microspheres for pulmonary drug delivery. Drug Deliv 21(3):185–92
- Fulzele SV, Shaik MS, Chatterjee A, Singh M. (2006). Anti-cancer effect of celecoxib and aerosolized docetaxel against human non-small cell lung cancer cell line, A549. J Pharm Pharmacol 58:327–36
- Garbuzenko OB, Mainelis G, Taratula O, Minko T. (2014a). Inhalation treatment of lung cancer: the influence of composition, size and shape of nanocarriers on their lung accumulation and retention. Cancer Biol Med 11:44–55
- Garbuzenko OB, Winkler J, Tomassone MS, Minko T. (2014b). Biodegradable Janus nanoparticles for local pulmonary delivery of hydrophilic and hydrophobic molecules to the lungs. Langmuir 30:12941–9
- Geller DE. (2002). New liquid aerosol generation devices: systems that force pressurized liquids through nozzles. Respir Care 47:1392–404
- Gill KK, Nazzal S, Kaddoumi A. (2011). Paclitaxel loaded PEG(5000)-DSPE micelles as pulmonary delivery platform: formulation characterisation, tissue distribution, plasma pharmacokinetics, and toxicological evaluation. Eur J Pharm Biopharm 79:276–84
- Hanahan D, Weinberg RA. (2011). Hallmarks of cancer: the next Generation. Cell 144:646–74
- Hart MG, Grant R, Garside R, et al. (2011). Chemotherapy wafers for high grade glioma. Cochrane Database Syst Rev 2011:CD007294
- Haynes A, Shaik MS, Chatterjee A, Singh M. (2003). Evaluation of an aerosolized selective COX-2 inhibitor as a potentiator of doxorubicin in a non-small-cell lung cancer cell line. Pharm Res 20:1485–95
- Hernández-Trejo N, Kayser O, Steckel H, Müller RH. (2005). Characterization of nebulized buparvaquone nanosuspensions – effect of nebulization technology. J Drug Target 13:499–507
- Hoet PH, Brüske-Hohlfeld I, Salata OV. (2004). Nanoparticles – known and unknown health risks. J Nanobiotechnol 2:12
- Holback H, Yeo Y. (2011). Intratumoral drug delivery with nanoparticulate carriers. Pharm Res 28:1819–30
- Ibald-Mulli A, Wichman HE, Kreyling W, Peters A. (2002). Epidemiological evidence on health effects of ultrafine particles. J Aerosol Med 15:189–201
- Jia Y, Yuan M, Yuan H, et al. (2012). Co-encapsulation of magnetic Fe3O4 nanoparticles and doxorubicin into biodegradable PLGA nano-carriers for intratumoral delivery. Int J Nanomedicine 7:1697–708
- Jinturkar KA, Anish C, Kumar MK, et al. (2012). Liposomal formulations of etoposide and docetaxel for p53 mediated enhanced cytotoxicity in lung cancer cell lines. Biomaterials 33:2492–507
- Jones MC, Jones SA, Riffo-Vasquez Y, et al. (2014). Quantitative assessment of nanoparticle surface hydrophobicity and its influence on pulmonary biocompatibility. J Control Release 183:94–104
- Joshi N, Shanmugam T, Kaviratna A, Banerjee R. (2011). Proapoptotic lipid nanovesicles: synergism with paclitaxel in human lung adenocarcinoma A549 cells. J Control Release 156:413–20
- Joshi N, Shirsath N, Singh A, et al. (2014). Endogenous lung surfactant inspired pH responsive nanovesicle aerosols: pulmonary compatible and site-specific drug delivery in lung metastases. Sci Rep 4:7085
- Jyoti K, Kaur K, Pandey RS, et al. (2015). Inhalable nanostructured lipid particles of 9-bromo-noscapine, a tubulin-binding cytotoxic agent: In vitro and in vivo studies. J Colloid Interface Sci 445:219–30
- Kang YM, Kim GH, Kim JI, et al. (2011). In vivo efficacy of an intratumorally injected in situ forming doxorubicin/poly(ethylene glycol)-β-caprolactone diblock copolymer. Biomaterials 32:4556–64
- Kar UK, Srivastava MK, Andersson A, et al. (2011). Novel CCL21-vault nanocapsule intratumoral delivery inhibits lung cancer growth. PLoS One 6:e18758
- Kaur P, Garg T, Rath G, et al. (2015). Surfactant-based drug delivery systems for treating drug-resistant lung cancer. Drug Deliv 21:1–2. doi:10.3109/10717544.2014.935530
- Khanna C, Anderson PM, Hasz DE, et al. (1997). Interleukin-2 liposome inhalation therapy is safe and effective for dogs with spontaneous pulmonary metastases. Cancer 79:1409–21
- Kleinstreuer C, Zhang Z, Donohue JF. (2008). Targeted drug-aerosol delivery in the human respiratory system. Annu Rev Biomed Eng 10:195–220
- Knight V, Koshkina NV, Waldrep JC, et al. (1999). Anticancer effect of 9-nitrocamptothecin liposome aerosol on human cancer xenografts in nude mice. Cancer Chemother Pharmacol 44:177–86
- Koshkina NV, Kleinerman ES, Waidrep C, et al. (2000). 9-Nitrocamptothecin liposome aerosol treatment of melanoma and osteosarcoma lung metastases in mice. Clin Cancer Res 6:2876–80
- Koshkina NV, Knight V, Gilbert BE, et al. (2001). Improved respiratory delivery of the anticancer drugs, camptothecin and paclitaxel, with 5% CO2 enriched air: pharmacokinetic studies. Cancer Chemother Pharmacol 47:451–6
- Koshkina NV, Waldrep JC, Knight V. (2003). Camptothecins and lung cancer: improved delivery systems by aerosol. Curr Cancer Drug Targets 3:251–64
- Labiris NR, Dolovich MB. (2003). Pulmonary drug delivery. Part II: the role of inhalant delivery devices and drug formulations in therapeutic effectiveness of aerosolized medications. J Clin Pharmacol 56:600–12
- Lebhardt T, Roesler S, Uusitalo HP, Kissel T. (2011). Surfactant-free redispersible nanoparticles in fast-dissolving composite microcarriers for dry-powder inhalation. Eur J Pharm Biopharm 78:90–6
- Liu R, Wolinsky JB, Walpole J, et al. (2010). Prevention of local tumour recurrence following surgery using low dose chemotherapeutic polymer films. Ann Surg Oncol 17:1203–13
- Long JT, Cheang TY, Zhuo SY, et al. (2014). Anticancer drug-loaded multifunctional nanoparticles to enhance the chemotherapeutic efficacy in lung cancer metastasis. J Nanobiotechnol 12:37
- Lowe SW, Bodis S, McClatchey A, et al. (1994). p53 status and the efficacy of cancer therapy in vivo. Science 266:807–10
- Lukyanov AN, Torchilin VP. (2004). Micelles from lipid derivatives of water-soluble polymers as delivery systems for poorly soluble drugs. Adv Drug Deliv Rev 56:1273–89
- Mansour HM, Rhee YS, Wu X. (2009). Nanomedicine in pulmonary delivery. Int J Nanomedicine 4:299–319
- Meenach SA, Anderson KW, Zach Hilt J, et al. (2013). Characterization and aerosol dispersion performance of advanced spray-dried chemotherapeutic PEGylated phospholipid particles for dry powder inhalation delivery in lung cancer. Eur J Pharm Sci 49:699–711
- Misra A, Jinturkar K, Patel D, et al. (2009). Recent advances in liposomal dry powder formulations: preparation and evaluation. Expert Opin Drug Deliv 6:71–89
- Muralidharan P, Malapit M, Mallory E, et al. (2015). Inhalable nanoparticulate powders for respiratory delivery. Nanomedicine 11:1189–99
- Oberdörster G, Oberdörster E, Oberdörster J. (2005). Nanotoxicology: an emerging discipline evolving from studies of ultrafine particles. Environ Health Perspect 113:823–39
- Parker DM. (2001). Global cancer statistics in the year 2000. Lancet Oncol 2:533–43
- Patlolla RR, Chougule M, Patel AR, et al. (2010). Formulation, characterization and pulmonary deposition of nebulized celecoxib encapsulated nanostructured lipid carriers. J Control Release 144:233–41
- Patton JS, Byron PR. (2007). Inhaling medicines: delivering drugs to the body through the lungs. Nat Rev Drug Discov 6:67–74
- Rao RD, Markovic SN, Anderson PM. (2003). Aerosol therapy for malignancy involving the lungs. Curr Cancer Drug Targets 3:238–50
- Roa WH, Azarmi S, Al-Hallak MH, et al. (2011). Inhalable nanoparticles, a non-invasive approach to treat lung cancer in a mouse model. J Control Release 150:49–55
- Rotoli BM, Gatti R, Movia D, et al. (2015). Identifying contact-mediated, localized toxic effects of MWCNT aggregates on epithelial monolayers: a single-cell monitoring toxicity assay. Nanotoxicology 9:230–41
- Sadhukha T, Wiedmann TS, Panyam J. (2013). Inhalable magnetic nanoparticles for targeted hyperthermia in lung cancer therapy. Biomaterials 34:5163–71
- Sharma S, Stolina M, Luo J, et al. (2000). Secondary lymphoid tissue chemokine mediates T cell-dependent antitumor responses in vivo. J Immunol 164:4558–63
- Singh J, Garg T, Rath G, Goyal AK. (2015). Advances in nanotechnology-based carrier systems for targeted delivery of bioactive drug molecules with special emphasis on immunotherapy in drug resistant tuberculosis – a critical review. Drug Deliv. [Epub ahead of print]. doi: 10.3109/10717544.2015.1074765
- Skubitz KM, Anderson PM. (2000). Inhalational interleukin-2 liposomes for pulmonary metastases: a phase I clinical trial. Anticancer Drugs 11:555–63
- Sonvico F, Dubernet C, Colombo P, Couvreur P. (2005). Metallic colloid nanotechnology, applications in diagnosis and therapeutics. Curr Pharm Des 11:2095–105
- Taratula O, Garbuzenko OB, Chen AM, Minko T. (2011). Innovative strategy for treatment of lung cancer: targeted nanotechnology-based inhalation co-delivery of anticancer drugs and siRNA. J Drug Target 19:900–14
- Taratula O, Kuzmov A, Shah M, et al. (2013). Nanostructured lipid carriers as multifunctional nanomedicine platform for pulmonary co-delivery of anticancer drugs and siRNA. J Control Release 171:349–57
- Tewes F, Ehrhardt C, Healy AM. (2014). Superparamagnetic iron oxide nanoparticles (SPIONs)-loaded Trojan microparticles for targeted aerosol delivery to the lung. Eur J Pharm Biopharm 86:98–104
- Thiesen B, Jordan A. (2008). Clinical applications of magnetic nanoparticles for hyperthermia. Int J Hyperthermia 24:467–74
- Tomoda K, Ohkoshi T, Hirota K, et al. (2009). Preparation and properties of inhalable nanocomposite particles for treatment of lung cancer. Colloids Surf B Biointerfaces 71:177–82
- Torchilin VP. (2005). Recent advances with liposomes as pharmaceutical carriers. Nat Rev Drug Discov 4:145–60
- Tseng CL, Su WY, Yen KC, et al. (2009). The use of biotinylated-EGF-modified gelatin nanoparticle carrier to enhance cisplatin accumulation in cancerous lungs via inhalation. Biomaterials 30:3476–85
- Verma NK, Crosbie-Staunton K, Satti A, et al. (2013). Magnetic core-shell nanoparticles for drug delivery by nebulisation. J Nanobiotechnol 11:1–12
- Verschraegen CF, Gilbert BE, Loyer E, et al. (2004). Clinical evaluation of the delivery and safety of aerosolised liposomal 9-nitro-20(s)-camptothecin in patients with advanced pulmonary malignancies. Clin Cancer Res 10:2319–26
- Videira M, Almeida AJ, Fabra A. (2012). Preclinical evaluation of a pulmonary delivered paclitaxel-loaded lipid nanocarrier antitumor effect. Nanomedicine 8:1208–15
- Wang C, Chen T. (2012). Intratumoral injection of Taxol in vivo suppresses A549 tumor showing cytoplasmic vacuolisation. J Cell Biochem 113:1397–406
- Westphal M, Hilt DC, Bortey E, et al. (2006). A phase 3 trial of local chemotherapy with biodegradable carmustine (BCNU) wafers (Gliadel wafers) in patients with primary malignant glioma. Neuro Oncol 5:79–88
- Willis L, Hayes D Jr, Mansour HM. (2012). Therapeutic liposomal dry powder inhalation aerosols for targeted lung delivery. Lung 190:251–62
- Wittgen BP, Kunst PW, Perkins WR, et al. (2006). Assessing a system to capture stray aerosol during inhalation of nebulized liposomal cisplatin. J Aerosol Med 19:385–91
- Wittgen BP, Kunst PW, van der Born K, et al. (2007). Phase I study of aerosolized SLIT cisplatin in the treatment of patients with carcinoma of the lung. Clin Cancer Res 13:2414–21
- Wolinsky JB, Colson YL, Grinstaff MW. (2012). Local drug delivery strategies for cancer treatment: gels, nanoparticles, polymeric films, rods, and wafers. J Control Release 159:14–26
- Yohe ST, Colson YL, Grinstaff MW. (2012a). Superhydrophobic materials for tuneable drug release: using displacement of air to control delivery rates. JACS 134:2016–19
- Yohe ST, Herrera VL, Colson YL, Grinstaff MW. (2012b). 3D superhydrophobic electrospun meshes as reinforcement materials for sustained local drug delivery against colorectal cancer cells. J Control Release 162:92–101
- Yoo GH, Subramanian G, Ezzat WH, et al. (2010). Intratumoral delivery of docetaxel enhances antitumor activity of Ad-p53 in murine head and neck cancer xenograft model. Am J Otolaryngol 31:78–83
- Yu Y, Kong L, Li L, et al. (2015). Antitumor activity of doxorubicin-loaded carbon nanotubes incorporated poly(lactic-co-glycolic acid) electrospun composite nanofibers. Nanoscale Res Lett 10:3434
- Zarogoulidis P, Chatzaki E, Porpodis K, et al. (2012a). Inhaled chemotherapy in lung cancer: future concept of nanomedicine. Int J Nanomedicine 7:1551–72
- Zarogoulidis P, Gialeli C, Karamanos NK. (2012b). Inhaled chemotherapy in lung cancer: safety concerns of nanocomplexes delivered. Ther Deliv 3:1021–23
- Zhang Z, Kleinstreuer C, Kim CS. (2008). Airflow and nanoparticle deposition in a 16-generation tracheobronchial airway model. Ann Biomed Eng 36:2095–110
- Zhang Z, Mei L, Feng SS. (2013). Paclitaxel drug delivery systems. Exp Opin Drug Deliv 10:325–40