Abstract
Diagnosis and therapy of brain cancer was often limited due to low permeability of delivery materials across the blood–brain barrier (BBB) and their poor penetration into the brain tissue. This study explored the possibility of utilizing theranostic d-alpha-tocopheryl polyethylene glycol 1000 succinate mono-ester (TPGS) liposomes as nanocarriers for minimally invasive brain-targeted imaging and therapy (brain theranostics). The aim of this work was to formulate transferrin conjugated TPGS coated theranostic liposomes, which contain both docetaxel and quantum dots (QDs) for imaging and therapy of brain cancer. The theranostic liposomes with and without transferrin decoration were prepared and characterized for their particle size, polydispersity, morphology, drug encapsulation efficiency, in-vitro release study and brain theranostics. The particle sizes of the non-targeted and targeted theranostic liposomes were found below 200 nm. Nearly, 71% of drug encapsulation efficiency was achieved with liposomes. The drug release from transferrin conjugated theranostic liposomes was sustained for more than 72 h with 70% of drug release. The in-vivo results indicated that transferrin receptor-targeted theranostic liposomes could be a promising carrier for brain theranostics due to nano-sized delivery and its permeability which provided an improved and prolonged brain targeting of docetaxel and QDs in comparison to the non-targeted preparations.
Introduction
Brain cancer is considered as one of the most devastating disease because it is difficult to treat and cure. Chemotherapy is the most common method for the treatment of different types of brain cancers. Unfortunately, the diagnosis and therapy of brain cancer are very unsatisfying because of the existence of the blood–brain barrier (BBB), which excludes more than 98% of small molecules and almost 100% of large molecules. Another obstacle in cancer therapy is to maintain a desired concentration of therapeutic agents at the desired tumor site which can prevent their spreading into healthy tissues (Daniels et al., Citation2012; Burgo et al., Citation2014; Zong et al., Citation2014). Therefore, a reasonable approach is needed for designing of theranostic platforms which can cross the BBB and target to tumor cells thus, improving diagnostic and therapeutic opportunities, efficiency, and patient survival while reducing the adverse effect to the normal cells (Wilson et al., Citation2008). Now, various types of nanomedicines are developed, for example, polymeric nanoparticles, solid lipid nanoparticles, liposomes, dendrimers, polymeric micelles and carbon nanotubes for effective therapy of brain cancers (Muthu & Singh, Citation2009; Muthu & Wilson, Citation2012). Liposomes are considered as an ideal candidate for theranostics because of their safety, biocompatibility and biodegradability. Recently, the marketed liposomes and stealth liposomes have made impact for cancer therapy because they can escape from reticuloendothelial system (RES) and circulate in the blood for a long period. For example, Doxil, Caelyx and Myocet are nanosized liposomes (encapsulated with doxorubicin in their aqueous core) which have been used for the treatments of Kaposi’s sarcoma, ovarian cancer and multiple myelomas (Anabousi et al., Citation2006; Li et al., Citation2009; Muthu & Feng, Citation2013). It was found that polyethylene glycol (PEG) coating of liposomes resulted in extended blood circulation half-life in combination with an increased permeability of tumor vasculature which causes passive accumulation of PEG liposomes in various types of tumors. Passive and active targeting can also be realized by enhanced permeability and retention (EPR) effect and ligand conjugations (Schnyder & Huwyler, Citation2005; Muthu et al., Citation2011).
In recent years, d-alpha-tocopheryl polyethylene glycol 1000 succinate monoester (TPGS) has emerged as an amphiphilic copolymer for developing the various drug delivery systems. TPGS is a water-soluble derivative of natural vitamin E and PEG 100, which has amphiphilic structure of lipophilic alkyl tail and hydrophilic polar head with a hydrophile–lipophile balance (HLB) value of 13.2 and critical micelle concentration (CMC) of 0.02% w/w. It has been approved by FDA as a safe pharmaceutical ingredient. (Zhang et al., Citation2012; Vijayakumar et al., Citation2013; Kutty et al., Citation2015, Muthu et al., Citation2016). Its bulky structure and large surface area make it an excellent emulsifier, solubilizer, stabilizer and absorption enhancer of hydrophobic drugs (Feng, Citation2006; Zhao & Feng, Citation2010; Muthu et al., Citation2012a, 2012b). Nano drug delivery systems formulated with TPGS such as liposomes and micelles have exhibited higher drug encapsulation efficiency, cellular uptake, and cell cytotoxicity on cancer cells as well as an extended circulation time up to 360 h and enhanced therapeutic efficiency. One of the major advantages of TPGS is that it has shown great potential in overcoming multi-drug resistance (MDR) as a kind of p-glycoprotein (p-gp) inhibitor via inhibition of the activity of the ATPase part of p-gp (Bao et al., Citation2014; Song et al., Citation2014). In one study, Muthu et al. (Citation2011) prepared TPGS-coated theranostic liposomes containing docetaxel (DTX) and quantum dots (QDs) with and without targeting moieties. Folic acid was used as targeting probe to target folate receptor overexpressing MCF-7 breast cancer cell lines. Higher cellular uptake and cytotoxicity of the drug by the targeting theranostic liposomes were observed in comparison with those by the non-targeting liposomal formulation. In another study, Muthu et al. (Citation2012a) have prepared DTX-loaded TPGS micelles, which showed three times increase of cytotoxicity than control DTX in brain cancer cells. Biodistribution study in normal rats shows that micelles of DTX were found to be present longer time in brain, lungs and blood in comparison to control DTX.
Theranostic liposomes are generally designed to facilitate simultaneous imaging and therapy i.e., to track whether drugs are specifically delivered to target organs. The imaging agents are often incorporated with liposomes to monitor liposomal distributions. QDs, the semiconductor nanocrystals have recently emerged as an imaging reagent which can overcome the drawbacks of conventional organic fluorophores. It shows a better photostability (100–10 000 times greater) than conventional organic dyes. The QDs have narrow band emissions together with large ultraviolet absorption spectra, which enable multiplex imaging under a single light. However, QDs contain heavy metals which have potential toxicities in vitro and in vivo (Muthu et al., Citation2012a, 2015; Wen et al., Citation2013). But, the encapsulation of QDs into liposomes can greatly improve biocompatibility because they provide sufficient space for entrapping a large number of drug molecules. They are ideal nanocarriers for theranostic applications as they protect the cargos against degradation, prolong half-life, control drug delivery and minimize adverse effects. Incorporation of QDs within the hydrophobic membrane or linked covalently to the surface of the liposomes with the therapeutic agents and targeting ligand, makes it a feasible and novel strategy for cellular and in vivo tumor imaging with targeting and therapeutic modalities. These multifunctional liposomes are integrating QDs, drug and targeting ligand which will become effective platforms for synchronous diagnosis and treatment (Weng et al., Citation2008, Citation2012; Ni et al., Citation2014; Muthu et al., Citation2014a,Citationb).
Recently, the performance of liposomal drug delivery systems is improved by active targeting, e.g. by using a ligand coupled to the surface which recognizes specific marker molecules or receptors on tumor cells. Among others, the transferrin receptor has been found as one of the successful target molecules. Transferrin (ß-1 glycopeptide) is a hydrophilic transport vector, controls the extracellular iron level in the body fluid by binding and sequestering. It is known to be internalized into the cells by means of transferrin-receptor (Tf-R)-mediated endocytosis (Kobayashi et al., Citation2007; Daniels et al., Citation2012). Transferrin receptor overexpression has been targeted for improving the BBB transport of drugs by numerous researchers (Kuo et al., Citation2011; Li et al., Citation2012). Many studies have indicated, transferrin receptor expression on cancer cells can be up to 100-fold higher than the average expression in normal cells owing to their rapid proliferation rate and iron demand, thereby transferrin has been explored as a targeting ligand for nanocarriers to deliver diagnostic and therapeutic agent into cancer cells (Jones & Shusta, Citation2007; Ulbrich et al., Citation2009; Xu et al., Citation2012).
Here, we are using DTX (N-debenzoyl-N-tert-butoxycarbonyl-10-deacetyl-paclitaxel) as a model drug which is a semi-synthetic derivative of the taxoid family of anti-neoplastic agents. It has been found more effective than paclitaxel against breast, ovarian, lung and neck cancers (Bissery et al., Citation1991). However, the efficacy of systemic treatment with DTX against brain cancer is limited. Probably a major cause of this lack of efficacy is the BBB, which restricts the penetration of drugs into the brain tumor tissue. An important component of the BBB is P-glycoprotein (P-gp), which acts as a drug efflux pump. There is need to develop a better adjuvant formulation which can inhibit the P-gp to increase the penetration of drug as well as can target the brain cancer cells to increase its therapeutic outcome while reducing adverse effects (Kemper et al., Citation2004; Liu et al., Citation2010; Gan & Feng, Citation2010).
We aim in this research to develop theranostic TPGS liposomes for transferrin receptor targeting co-delivery of DTX and QDs, and to prove their theranostic effects for brain cancer applications. Firstly, acid functionalized D-α-tocopheryl polyethylene glycol 1000 succinate (TPGS-COOH) was conjugated covalently to the amino ends of transferrin (i.e., TPGS-Tf). Secondly, conjugate (TPGS-Tf) was assembled on the surface of the liposomes and a therapeutic and imaging agent (i.e., DTX and QDs) was encapsulated in the lipophilic portion of the liposomes by solvent injection method. The liposomes were then characterized for their size and size distribution, surface morphology, drug encapsulation efficiency and drug release profile. Further, liposomes were tested in vivo for brain theranostics after intravenous (i.v). administration to prove the transport efficiency of DTX and QDs across the BBB and its targeted delivery into the brain.
Materials and methods
The drug of choice in the present investigation, docetaxel (DTX) was obtained from Neon Laboratories Ltd, Mumbai, India, as gift sample. d-Alpha-tocopheryl polyethylene glycol 1000 succinate (TPGS) was obtained from Antares Health Products., St. Charles, IL, as gift sample. Transferrin was purchased from Sigma–Aldrich Chemicals, St. Louis, MO. Lipoid PC (DPPC) was obtained from Lipoid GmbH, Ludwigshafen, Germany, as gift sample. Cholesterol was purchased from Loba Chemie Pvt. Ltd, Mumbai, India. CdSe/ZnS quantum dots (QDs) were purchased from Reinste, New Delhi, India. Dialysis membrane (Spectra/Por7®) of 1 KDa molecular weight cutoff was purchased from Spectrum Laboratories Inc., Rancho Dominguez, CA. Docel™ was purchased from RPG Life Sciences Limited, Mumbai, India. Dialysis membrane with a 12 KDa molecular weight cutoff was purchased from Sigma-Aldrich Chemicals, France. All other chemicals were of analytical grade.
Synthesis of TPGS-COOH (activation of TPGS) and its conjugate
TPGS was activated (i.e., synthesis of TPGS-COOH) by succinic anhydride through ring-opening polymerisation (ROP) reaction in the presence of DMAP () (Raju et al., Citation2013; Muthu et al., Citation2015). In brief, TPGS (0.77 g, 0.5 mM), succinic anhydride (0.10 g, 1 mM) and DMAP (0.12 g, 1 mM) were mixed and heated at 100 °C under the nitrogen atmosphere for 24 h. The mixture was cooled to room temperature, dissolved in 5 ml of cold dichloromethane, filtered to remove excessive succinic anhydride and then precipitated in 100 ml of diethyl ether at −10 °C overnight. The white TPGS-COOH precipitate was filtered and dried in vacuum.
Figure 1. Schematic diagram for (A) synthesis of (i) TPGS-COOH, (ii) TPGS-Tf and (B) preparation of (i) non-targeted (DTX-QD-TPGS) and (ii) transferrin receptor targeted (DTX-QD-TPGS-Tf) liposomes. DTX-QD-TPGS: Non-targeted DTX and QDs-loaded theranostic TPGS Liposomes. DTX-QD-TPGS-Tf: Transferrin receptor targeted DTX and QDs-loaded theranostic TPGS liposomes.
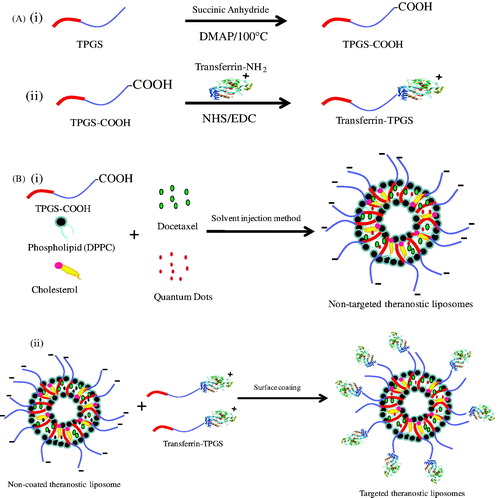
Conjugation of transferrin to TPGS-COOH was achieved by carbodiimide chemistry with the help of EDC and NHS in phosphate buffer saline (pH 5.5) (Muthu et al., Citation2015). In brief, for conjugation of transferrin to TPGS-COOH, 1-ethyl-3-(3-dimethylaminopropyl) carbodiimide (EDC) and N-hydroxysuccinimide (NHS) (both are catalyst) were added into TPGS-COOH with a molar ratio of 1:5 (TPGS-COOH: EDC or NHS) in phosphate buffer saline (pH 5.5). In brief, TPGS-COOH (200 mg), EDC (96 mg) and NHS (74 mg) were mixed in 2 ml of phosphate buffer saline (pH 5.5) at 25 °C for 5 h, stored in a refrigerator at 4 °C over 24 h, mixed with 1 ml of 0.2% (w/v) transferrin and stirred at 4 °C over 8 h. The resulted product was then dialyzed using a dialyzing membrane (MWCO: 12 kDa) against phosphate buffer saline (pH 5.5) for 48 h in order to remove excess TPGS-COOH, NHS and EDC. The dialyzed product (i.e., TPGS-Tf) was freeze-dried and used for liposome formation.
Characterization of TPGS-COOH and its conjugate
Fourier transform infrared (FTIR) spectroscopy
FTIR spectrophotometer (Perkin Elmer Spectrum Two, Waltham, MA) was used to investigate the molecular structure of activated TPGS (TPGS-COOH) and transferrin conjugated TPGS (TPGS-Tf). The scanning range was 400–4000 cm−1 and the resolution used was 2 cm−1 (Raju et al., Citation2013; Sonali et al., Citation2015).
Proton nuclear magnetic resonance (1H NMR) spectrophotometery
The structure of synthesized TPGS-COOH and TPGS-Tf were confirmed by 1H NMR spectrophotometer (JEOL FX Fourier Transform Multinuclear NMR Spectrometer, Tokyo, Japan). For nuclear magnetic resonance (NMR) analysis, TPGS-COOH and TPGS-Tf were solubilized in CDCl3 and D2O respectively before analysis (Sonali et al., Citation2015).
Preparation of TPGS liposomes
TPGS coated theranostic liposomes with or without transferrin targeting were prepared according to the solvent injection method. In brief, DTX, DPPC, cholesterol, and TPGS-COOH were dissolved in 0.3 ml of ethanol at 60 °C, according to the formulae (). In addition, 100 μl of QDs (dispersed in THF as 1 mg/ml concentration) were also added in the ethanol system for preparation of theranostic liposomes. The mixture was then injected into 2.7 ml of 1 mM phosphate buffered saline (PBS), pH 7.4 isothermally at 60 °C. The suspension formed was kept at 60 °C under stirring for 60 min to form multilamellar vesicles (MLV). The small unilamellar vesicles were prepared from the MLV suspension by ultrasonication (Heilscher UP200H, Teltow, Germany) for 5 min followed by filtration through 0.22 μm filter while keeping the lipids above the phase transition temperature (Tm). Finally, liposomes were centrifuged at 11 000 rpm for 15 min to remove the excess non-incorporated drug and QDs, and stored at 4 °C. For the preparation of targeted theranostic liposomes, TPGS-Tf was added into the prepared liposomes and incubated for 1 h in water bath shaker for surface coating of TPGS-Tf by hydrophobic interaction of TPGS and electrostatic interaction of transferrin ( and ) (Muthu et al., Citation2012a). Liposomes formulae were established to get higher drug encapsulation, controlled drug release and stability.
Table 1. Formulae of preparations.
Table 2. Particle size, polydispersity index, zeta potential and encapsulation efficiency.
Liposome characterization
Particle size, polydispersity, zeta potential and surface morphology
Particle sizes, polydispersity and zeta potential of liposomes (both non-targeted and targeted) were measured at 25 °C by dynamic light scattering (DLS) technique (Delsa Nano C-Beckmann Coulter, Beckman Coulter India Pvt Ltd, Mumbai, India). Samples were scattered (658 nm) at an angle of 165°. Data were fitted by the method of inverse ‘‘Laplace transformation’’ and CONTIN. The liposome samples were analyzed after 50 times dilution with deionized water. Each value reported is the average of three measurements.
Surface morphology (particle size and the shape) was studied by a transmission electron microscopy (TEM) system (Philips CM-12., Fullerton, CA). Samples of liposomes were prepared by placing one drop on a copper grid and dried under vacuum pressure. The dried liposomes being stained with 1% phosphotungstic acid for 30 s were finally examined.
Additionally, surface morphology of liposomes was visualized by atomic force microscopy (AFM) under normal atmospheric conditions. The Explorer™ atomic force microscope (SOLVER NEXT, NT-MDT Co., Moscow, Russia) was in tapping mode, using high resonant frequency (F0 = 346 kHz) pyramidal cantilevers with silicon probes having force constants of 41 N/m. Scan speeds were set at 1 Hz. The samples were diluted twice with distilled water and then dropped onto glass slides, followed by vacuum drying during 24 h at 25 °C. The measurements were obtained using AFM image analysis software (NOVA-Px-3.1.0-Rev 3880).
Determination of encapsulation efficiency of liposomes
The encapsulation efficiency of liposomes was determined by UV spectrophotometer (Shimadzu 1800, Tokyo, Japan). A 0.2 ml aliquot of liposomes was taken in round bottom flask and evaporated to dryness by rotary evaporator under reduced pressure at 40 °C, and then it was dissolved in 5 ml of methanol and sonicated to disrupt the vesicles. From this sample, 1 ml of aliquot was withdrawn and diluted to 10 ml with methanol. The solution was filtered through 0.45 μm syringe filter. Then light absorption was measured at 229 nm using spectrophotometer. The drug encapsulation efficiency was defined as the ratio between the amount of DTX encapsulated in the liposomes and that added in the liposomes preparation process (Sonali et al., Citation2015; Singh et al., Citation2016). The QDs encapsulated in the theranostic liposomes was measured by fluorescence spectrometer (JY HORIBA, Horiba Jobin Yvon Inc, Longjumeau Cedex, France). Briefly, 0.2 ml of liposomes was freeze-dried and re-dissolved in 1 ml of dichloromethane (DCM) before emission analysis. The emission at 654 nm under excitation at 458 nm was measured to determine the QDs content in the DCM solution using a previously established calibration curve of QDs in DCM. The QDs encapsulation efficiency was defined as the ratio between the amount of QDs encapsulated in the liposomes and that added in the liposomes preparation process. All samples were done in triplicate (Muthu et al., Citation2012a).
In-vitro drug release studies
The dialysis bag diffusion technique was used to study the in vitro drug release from the DTX-loaded liposomes. The drug-loaded liposomes of a volume equivalent to 150 μg (0.3 ml) of DTX were placed in the dialysis bag (cellulose membrane, molecular weight cut-off 1000 Da), hermetically sealed and immersed into 20 ml of phosphate buffered saline (pH 7.4) (PBS). The entire system was kept at 37 ± 0.5 °C with continuous shaking at 100 rpm/min. Samples were withdrawn from the receptor compartment at predetermined time intervals and replaced with fresh medium. The samples were filtered through 0.45 μm syringe filter and then absorbance was measured by UV spectrophotometer (Sonali et al., Citation2015).
In-vivo study – brain theranostic evaluation of liposomes in rats
Charles Foster (CF) rats (200 ± 20 g) of Institute of Medical Sciences, Banaras Hindu University, India, were used to study the brain theranostic effect of liposomes in rats. Before experimentation they were kept in groups of six and fed with standard diet with water ad libitum. All experimental procedures were reviewed and approved by the animal and ethics review committee of Banaras Hindu University, India (Dean/2015/CAEC/1263).
The rats were randomly distributed into 13 groups with four rats in each group (n = 4). Groups 1–3 received an i.v. injection (via lateral tail vein) of saline control, Group 4 received an i.v. injection of free QDs (only for 0.5 h), Groups 5–7 received an i.v. injection of marketed formulation Docel™ (as control) while Groups 8–10 and Groups 11–13 received i.v. injection of the DTX-loaded non-targeted and brain-targeted theranostic liposomal formulations, respectively. Totally 52 rats were used for this study. The formulations were administered through the lateral tail vein at a DTX dose of 1 mg/kg and/or QDs dose of 100 μg/kg of body weight.
All animals were regularly monitored for their general health condition, clinical signs, stress, movement and activity or mortality. At different time points after the treatment (0.5 h, 2 h and 4 h), rats were given with anesthesia (Urethane – 1.5 g/kg of rat, intraperitonealy) and brains were harvested after decapitation from different treatment groups. The brain samples were stored at −80 °C until further analysis. Then, the samples were freeze-dried and homogenized into powder. For brain distribution analysis, liquid–liquid extraction using ethyl acetate was performed to extract the drug from the brain samples. Subsequently, 100 mg of the powder was extracted with 1 ml ethyl acetate. The mixture was vigorously stirred on vortex shaker for 3 min. and extracted the drug. After that, the mixture was centrifuged at 11 000 rpm for 15 min. The organic layer was transferred to a new tube for drying. Then, the drug was reconstituted with 200 μl of high performance liquid chromatography (HPLC) mobile phase (acetonitrile: water 50:50 v/v). Then, 90 μl solution was transferred into HPLC insert for analysis and 20 μl was injected into the column at a flow rate 1.0 ml/min. Total run time was 15 min. The DTX content of the brain samples (obtained from control, non-targeted and targeted liposomes) was then calculated based on the pre-established calibration curve. The column effluent was detected with a UV-Visible detector at 229 nm (Gan et al., Citation2010; Muthu et al., Citation2012b). Simultaneously, brain uptake of QDs from theranostic liposome formulations (both non-targeted and targeted) were done to analyze the brain targeting effect. After 0.5 h, brain samples were collected for sections from Groups 4, 8 and 11. Before sectioning the brain samples were frozen at −80 °C. The frozen sections of 10 μm thickness were prepared with a Cryotome and mounted on gelatine-coated glass plates. The nucleus was stained with 10 μg/ml Hoechst 33342 for 4 min at room temperature. After PBS washing, images were observed and captured by a fluorescence microscope (EVOS® FL Cell Imaging System, Life Technologies, Carlsbad, CA).
Statistical analysis
Statistical analysis was performed by Student’s t-test. All results are given as mean ± standard deviation (S.D). Differences are considered statistically significant at p < 0.05.
Results and discussion
Characterization of TPGS-COOH and TPGS-Tf by FTIR and 1H NMR spectrophotometer
In FTIR spectra of TPGS-COOH, intensity of peak for –C = O stretching vibration in ester group was increased and observed at 1739.46 cm−1 that confirmed the formation of carboxylic group. In transferrin conjugated TPGS, a characteristic peak at 1633.08 cm−1 appeared which corresponds to the bonded amide stretching vibrations, thus confirming the successful conjugation of transferrin to the TPGS-COOH. The above results indicate the formation of TPGS-Tf conjugate. In 1H NMR spectra of TPGS-COOH, the triplet signals at 2.793, 2.815 and 2.910 ppm were observed which belong to –CH2 protons (poly ethyleneoxide) part of TPGS-COOH. The succinoyl methylene (–CH2) gave signals at 1.968 and 2.007 ppm, which confirm the formation TPGS-COOH. The 1H NMR spectra of TPGS-Tf demonstrated a signal at 4.238 ppm, which confirms the conjugation between free amino group of Tf and carboxylic group of TPGS-COOH (Sonali et al., Citation2015).
Particle size, polydispersity, zeta potential and surface morphology of liposomes
The mean particle size and polydispersity of the DTX-loaded theranostic liposomes (non-targeted and targeted) are listed in . DLS measurements were undertaken in multimodel analysis to get a true reflection of particle size distribution. The particle size distribution curves for all the samples were unimodal ( and ). The particle size and its population standard deviation of the non-targeted liposomes (DTX-TPGS), i.e. before conjugation of transferrin, were found to be 164.7 ± 1.50 nm. The sizes of the corresponding QD-loaded liposomes prepared by the solvent injection method and its population standard deviation were 168.2 ± 1.62 nm and 182.5 ± 1.80 nm for batches of non-targeted liposomes (DTX-QD-TPGS) and targeted liposomes (DTX-QD-TPGS-Tf), respectively. The particle size results indicate that incorporation of targeting ligand (transferrin) on the liposomes surface leads to a significant increase (p < 0.05) in the size in comparison to non-targeted liposomes (DTX-TPGS, DTX-QD-TPGS). The higher number of Tf molecules available to the surrounding of the liposomes leads to the formation of larger liposomes (). The polydispersity of all the batches showed quite narrow size distribution, which is nearer to 0.2–0.4 (). It was reported that liposomes in the sizes less than 200 nm are suitable for cancer drug delivery. Liposomes in such size enhance EPR effect to tumor by passive targeting (Muthu & Singh, Citation2009; Muthu et al., Citation2012a). The zeta potential showed that all the theranostic liposomes are negatively charged. The coating of transferrin (TPGS-Tf) on the liposome surface showed less negative charge in comparison with TPGS-coated liposomes (). This may be attributed to the presence of positive charged functional groups of transferrin.
Figure 2. Particle size results of DTX-TPGS, DTX-QD-TPGS and DTX-QD-TPGS-Tf. DTX-TPGS: Non-targeted DTX-loaded TPGS liposomes. DTX-QD-TPGS: Non-targeted DTX and QDs-loaded theranostic TPGS Liposomes. DTX-QD-TPGS-Tf: Transferrin receptor targeted DTX and QDs-loaded theranostic TPGS liposomes.
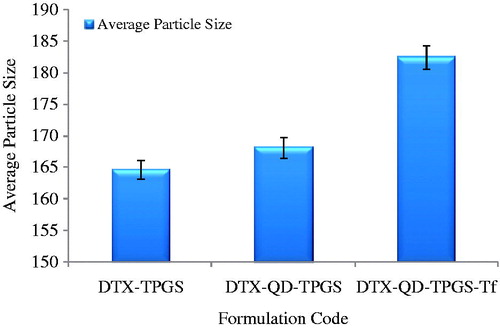
Figure 3. Histogram showing particle size and size distribution of (A) DTX-TPGS, (B) DTX-QD-TPGS, (C) DTX-QD-TPGS-Tf. DTX-TPGS: Non-targeted DTX-loaded TPGS liposomes. DTX-QD-TPGS: Non-targeted DTX and QDs-loaded theranostic TPGS liposomes. DTX-QD-TPGS-Tf: Transferrin receptor targeted DTX and QDs-loaded theranostic TPGS liposomes.
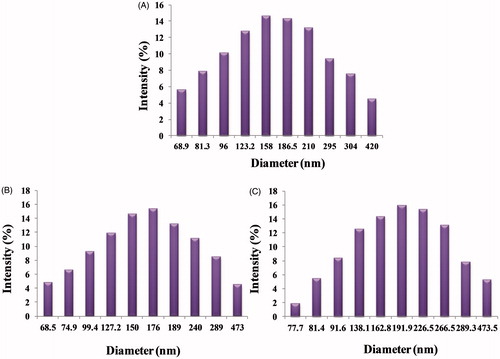
showed TEM images of (A) non-targeted theranostic liposomes (DTX-QD-TPGS) in 100 nm scale, (B) targeted theranostic liposomes (DTX-QD-TPGS-Tf) in 100 nm scale, and which revealed that the liposomes were spherical in shape (). The liposomes size as observed by TEM correlated, which also agreed well with that measured by DLS. Additionally, TEM images also confirmed the presence of transferrin coating on the liposomes surface (). The AFM images (2D and 3D images) of liposomes were shown in . Images show the smooth surface without any noticeable pinholes or cracks. AFM also revealed that the liposomes were spherical in shape of size below 200 nm, which agreed quite well with the result measured by DLS and TEM ().
Figure 4. Transmission electron microscope (TEM) image of (A) Theranostic non-targeted liposomes (DTX-QD-TPGS) in 100 nm scale, (B) Theranostic targeted liposomes (DTX-QD-TPGS-Tf) in 100 nm. DTX-QD-TPGS: Non-targeted DTX and QDs-loaded theranostic TPGS liposomes. DTX-QD-TPGS-Tf: Transferrin receptor targeted DTX and QDs-loaded theranostic TPGS liposomes.
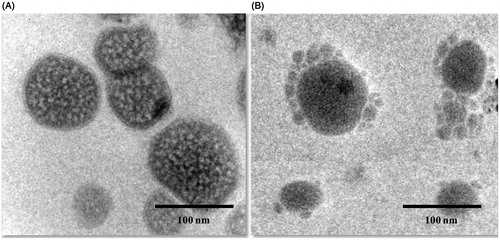
Drug encapsulation efficiency of liposomes
The drug encapsulation efficiency of the non-targeted DTX-loaded TPGS liposomes (DTX-TPGS) was 71.27 ± 2.30%. The drug encapsulation efficiency of non-targeted theranostic liposomes (DTX-QD-TPGS) and targeted theranostic liposomes (DTX-QD-TPGS-Tf) were found to be 69.75 ± 0.80 and 64.10 ± 0.88%, respectively ().The targeted liposomes showed significant decrease (p < 0.05) in the drug encapsulation efficiency. This may be because of influence of TPGS-Tf (conjugate) in the liposomes formation that leads to the disturbance in the hydrophobic membrane which resulted in drug loss during preparation process (Muthu et al., Citation2015) (). Further, QDs encapsulation efficiency of non-targeted theranostic liposomes and targeted theranostic liposomes were found to be 50.45 ± 1.20 and 51.19 ± 1.44%, respectively. Here, less encapsulation efficiency of QDs may be due to its larger size than DTX and thus likely to be lost in the preparation process of the liposomes (Muthu et al., Citation2012a).
In-vitro drug release studies
shows the cumulative percentage release of drug from the non-targeted liposomes (DTX-TPGS), non-targeted theranostic liposomes (DTX-QD-TPGS) and targeted theranostic liposomes (DTX-QD-TPGS-Tf) in phosphate buffered saline (pH 7.4). Non-targeted theranostic liposomes (DTX-QD-TPGS) and the targeted theranostic liposomes (DTX-QD-TPGS-Tf) showed slow and sustained release for more than 24 h without any burst release. After 72 h of dialysis in phosphate buffered saline (pH 7.4), the percentage of drug released from the liposomes was found to be 87.23% for non-targeted liposomes (DTX-TPGS), 72.41% for non-targeted theranostic liposomes (DTX-QD-TPGS) and 62.55% for targeted theranostic liposomes (DTX-QD-TPGS-Tf), respectively (). It was found that coating of transferrin on the surface of liposomes leads to much slower release kinetic of drug in comparison to non-targeted theranostic liposomes. The t50% (time at which 50% of the drug was released in medium) of the formulations were about 16, 24, and 48 h for the DTX-TPGS, DTX–QD-TPGS, and DTX-QD-TPGS-Tf, respectively ().
Figure 6. In-vitro drug release study of non-targeted TPGS liposomes (DTX-TPGS) and non-targetd theranostic (DTX-QD-TPGS) and targeted theranostic (DTX-QD-TPGS-Tf) TPGS liposomes in phosphate buffered saline (pH 7.4) (n = 3). DTX-TPGS: Non-targeted DTX-loaded TPGS liposomes. DTX-QD-TPGS: Non-targeted DTX and QDs-loaded theranostic TPGS liposomes. DTX-QD-TPGS-Tf: Transferrin receptor targeted DTX and QDs-loaded theranostic TPGS liposomes.
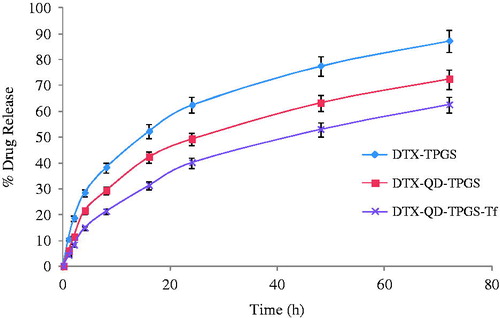
In-vivo brain theranostic study in rats
The brain distribution of DTX formulated in the non-targeted (DTX-QD-TPGS) and targeted (DTX-QD-TPGS-Tf) theranostic liposomes were investigated in comparison with marketed formulation Docel™ as control preparation and free QDs after i.v. administration ( and ). The key brain distribution pharmacokinetic parameters were analyzed using Phoenix WinNonlin 6.4 software (Princeton, NJ) and results are listed in , which include Tmax, the time at which drug present in maximum concentration, Cmax, the peak concentration of DTX and AUC (0–4 h), the total area under the curve which represents the brain DTX concentration. From results, it was found that the mean concentration of DTX in the brain tissue at 0.5, 2 and 4 h after the i.v. administration was significantly higher for targeted theranostic liposomes (DTX-QD-TPGS-Tf) than non-targeted theranostic liposomes (DTX-QD-TPGS) and Docel™ (p < 0.05). It was observed that the mean concentration of DTX in brain tissue at 0.5, 2 and 4 h after the i.v. administration was in the order of DTX-QD-TPGS-Tf > DTX-QD-TPGS > Docel™. It is worthy note that the non-targeted theranostic liposomes (DTX-QD-TPGS) achieved a higher concentration in brain compared to Docel™. This might be due to enhanced cellular uptake of nano-sized liposomes and sustained release manner of DTX from the liposomes. Further, the targeted theranostic liposomes (DTX-QD-TPGS-Tf) resulted in much higher concentration in brain tissue compared to non-targeted theranostic liposomes (DTX-QD-TPGS) and Docel™. The drug concentrations in brain for each of three preparations, namely Docel™, DTX-QD-TPGS and DTX-QD-TPGS-Tf were 24.7 ± 2.4 ng/g, 99.7 ± 6.87 ng/g and 385 ± 12.52 ng/g, respectively, which imply that drug formulated in the non-targeted and targeted theranostic liposomes could be 3.125 and 14.58 fold more efficient than Docel™ after 0.5 h of treatment, respectively. The drug concentrations in brain for each of the three preparations were 123 ± 14.41 ng/g, 674 ± 22.56 ng/g and 1220 ± 52.51 ng/g, respectively which imply that drug formulated in the non-targeted and targeted theranostic liposomes could be 4.47 and 8.91 fold more efficient than Docel™ after 2 h of treatment, respectively. The drug concentrations in brain for each of the three preparations were 39.5 ± 6.8 ng/g, 211 ± 19.47 ng/g and 457 ± 27.32 ng/g, respectively, which imply that drug formulated in the non-targeted and targeted liposomes could be 4.34 and 10.56 fold more efficient than Docel™ after 4 h of treatment, respectively (). It is straightforward to understand that targeted theranostic liposomes showed 2.86, 0.81 and 1.16 fold more drug concentration in brain tissue after 0.5, 2 and 4 h of treatment in comparison with non-targeted formulation (), which is mainly because of internalization of liposomes into brain by transferrin receptor mediated endocytic mechanism (Sonali et al., Citation2015; Muthu et al., Citation2015). The results show the controlled release and targeting effect of the targeted theranostic liposomes for extended duration due to increased brain uptake. The Cmax in brain for each of three preparations, namely Docel™, DTX-QD-TPGS and DTX-QD-TPGS-Tf liposomes were 123 ± 6.02 ng/g, 674 ± 16.12 ng/g and 1220 ± 26.10 ng/g respectively. Brain distribution kinetics of DTX in brain shows that maximum fold of drug concentrations in brain tissue were achieved at 2 h (Tmax) after the treatment with all the preparations (). Moreover, the AUC(0–4 h) for targeted theranostic liposomes was 2997.64 ± 42.50 ng.h/g which is 1.01 folds of non-targeted theranostic liposomes (1491.25 ± 33.60 ng.h/g) and 9.72-fold of Docel™ (279.45 ± 15.23 ng.h/g) ().
Figure 7. Brain distribution of DTX formulated in DocelTM, non-targeted theranostic TPGS (DTX-QD-TPGS) and targeted theranostic TPGS liposomes (DTX-QD-TPGS-Tf) after 0.5, 2 and 4 h of i.v. administration at the dose of 1 mg/Kg (n = 4). DTX-QD-TPGS: Non-targeted DTX and QDs-loaded theranostic TPGS liposomes. DTX-QD-TPGS-Tf: Transferrin receptor targeted DTX and QDs-loaded theranostic TPGS liposomes.
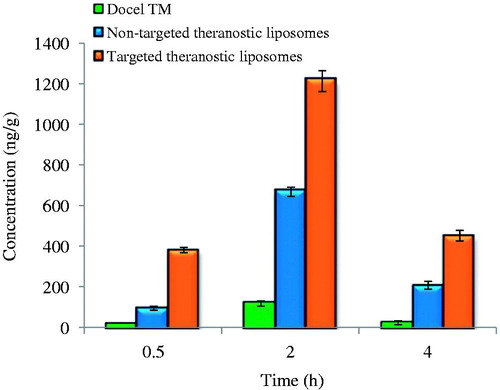
Figure 8. Fluorescence microscopic images of brain sections after 0.5 h i.v. injections of (A) free QDs (control) (from Group 4), (B) non-targeted theranostic (DTX-QD-TPGS) (from Group 8), and (C) targeted theranostic (DTX-QD-TPGS-Tf) TPGS liposomes (from Group 11). DAPI channels showing the blue fluorescence from Hoechst stained nuclei (left), QDs showing red fluorescence (middle), and Merged channels showing QDs and DAPI (right) (yellow circle is showing the presence of QDs in brain cells). Scale bar is 200 μm.
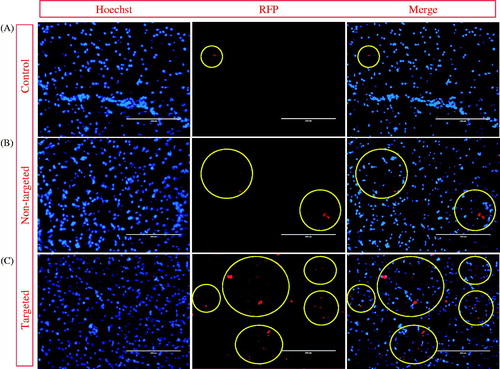
Table 3. Brain distribution kinetics of Docel™, non-targeted theranostic liposomes (DTX-QD-TPGS) and targeted theranostic liposomes (DTX-QD-TPGS-Tf) after i.v. injection at an equivalent dose of 1 mg/kg.
The results suggest that the transferrin coating on liposomes could significantly increase the BBB transport of DTX as active targeting (delivery). The higher expression of transferrin receptors on BBB enabled much greater proportion of targeted theranostic liposomes to be entered into brain via endocytosis mechanism relative to non-targeted theranostic liposomes or Docel™. Non-targeted theranostic liposomes also showed significant higher mean concentration of DTX in brain tissue in comparison to Docel™. It was thus demonstrated that the BBB might be crossed by liposomes with a size of approximately <200 nm and cause nonselective accumulation of DTX in brain. Further, the TPGS liposomes were able to inhibit p-glycoprotein (p-gp) based drug efflux in BBB that leads to enhancement in brain DTX concentrations with improved half-life (Zhao et al., Citation2013; Song et al., Citation2014). While, the Docel™ showed the lowest concentration of DTX in the brain as a result of the resistance from the BBB as well as the enhanced elimination rate from the body.
The brain biodistribution of fluorescent QDs formulated in the non-targeted (DTX-QD-TPGS) and targeted (DTX-QD-TPGS-Tf) liposomes was also investigated in comparison with the free QDs as control preparation after 0.5 h of i.v. administration of liposomal formulation simultaneously. It was observed that no fluorescence was detected in the control-treated images. Fluorescence intensity (more red color spots) was increased with non-targeted theranostic liposomes (DTX-QD-TPGS), while targeted theranostic liposomes (DTX-QD-TPGS-Tf) showed higher fluorescence intensity in comparison to control and non-targeted theranostic liposomes. The results confirm the higher brain uptake of the QDs formulated in the non-targeted and targeted theranostic liposomes. This was probably because of the much higher transportation of the QDs across the blood brain barrier by liposome formulations. Also, EPR effect observed for QDs formulated in the liposomes was higher in comparison to the free QDs that thus leads to increase concentration of QDs in the brain cells. Further, the higher fluorescent intensity of the QDs observed for targeted liposomes in comparison to non-targeted liposomes confirms transferrin receptor mediated delivery of QDs ().
Conclusion
In this work, we developed transferrin conjugated theranostic liposomes for targeted co-delivery of DTX and QDs into brain cancer cells. We have synthesized TPGS-COOH from TPGS then it was conjugated with transferrin. The characteristics of TPGS-COOH and conjugate (TPGS-Tf) were analyzed by FTIR spectrophotometer and NMR spectrophotometer. FTIR spectra and NMR spectra have revealed the characteristic peaks associated with the formation TPGS-COOH and TPGS-Tf. Firstly, we have prepared both non-targeted and targeted theranostic liposomes by solvent injection method. Further, non-targeted and targeted liposomes were evaluated for particle size, surface morphology, polydispersity index, drug encapsulation efficiency, in-vitro study and theranostic effect on brain. Particle size and polydispersity of both non-targeted and targeted liposomes were found to be satisfactory. The drug encapsulation efficiency was achieved up to 71% for both types of formulations. In-vitro release of DTX from the targeted formulation and non-targeted formulations showed slow and sustain release. Here, TPGS has been proven as an efficient spacer for targeted nano drug delivery. Also, we performed in vivo study to confirm the brain targeted theranostic potential of transferrin receptor targeted liposomes. In comparison with the marketed formulation Docel™ and non-targeting theranostic liposomes, the transferrin receptor targeted theranostic liposomes showed significantly higher delivery of DTX along with QDs into brain tissue due to the targeting effect of theranostic liposomes. As maximum non-targeted and targeted liposomes could be 4.41 and 8.91 fold more efficient when compared with Docel™ after 2 h treatment, respectively.
Declaration of interest
The authors report no conflicts of interest. The authors alone are responsible for the content and writing of the paper.
Author Dr. M.S. Muthu acknowledges the Science & Engineering Research Board (SERB), New Delhi, India, for a research project under Fast track scheme for young scientists (OYS). Project file number is SR/FT/LS-132/2011. Author Ms. Sonali has received Junior and Senior Research Fellowship from University Grants Commission (UGC), New Delhi, India, under the scheme of Rajiv Gandhi National Fellowship (RGNF) (RGNF-2012-13-SC-UTT-17607) to her doctoral research. We acknowledge Interdisciplinary School of Life Sciences (ISLS), Institute of Science, Banaras Hindu University, Varanasi, India for providing HPLC facility. We also acknowledge Prof. O.N. Srivastava, Department of Physics, Institute of Science, Banaras Hindu University, Varanasi, India in carrying out TEM analysis of samples.
References
- Anabousi S, Bakowsky U, Schneider M, et al. (2006). In vitro assessment of transferrin-conjugated liposomes as drug delivery systems for inhalation therapy of lung cancer. Eur J Pharm Sci 29:367–74
- Bao Y, Guo Y, Zhuang X, et al. (2014). D-α-Tocopherol polyethylene glycol succinate-based redox sensitive paclitaxel prodrug for overcoming multidrug resistance in cancer cells. Mol Pharm 11:3196–209
- Bissery MC, Guenard D, Gueritte-Voegelein F, et al. (1991). Experimental antitumor activity of taxotere (RP 56976, NSC 628503), a taxol analogue. Cancer Res 51:4845–52
- Burgo LSD, Hernández RM, Orive G, et al. (2014). Nanotherapeutic approaches for brain cancer management. Nanomedicine 10:905–19
- Daniels TR, Bernabeu E, Rodríguez JA, et al. (2012). The transferrin receptor and the targeted delivery of therapeutic agents against cancer. Biochim Biophys Acta 1820:291–317
- Feng SS. (2006). New-concept chemotherapy by nanoparticles of biodegradable polymers: where are we now? Nanomedicine (Lond) 1:297–309
- Gan CW, Feng SS. (2010). Transferrin-conjugated nanoparticles of Poly (lactide)-D-α-tocopheryl polyethylene glycol succinate diblock copolymer for targeted drug delivery across the blood brain barrier. Biomaterials 31:7748–57
- Gan CW, Chien S, Feng SS. (2010). Nanomedicine: enhancement of chemotherapeutic efficacy of docetaxel by using a biodegradable nanoparticle formulation. Curr Pharm Des 16:2308–20
- Jones AR, Shusta EV. (2007). Blood–brain barrier transport of therapeutics via receptor mediation. Pharm Res 24:1759–71
- Kemper EM, Verheij M, Boogerd W, et al. (2004). Improved penetration of docetaxel into the brain by co-administration of inhibitors of P-glycoprotein. Eur J Cancer 40:1269–74
- Kobayashi T, Ishida T, Okada Y, et al. (2007). Effect of transferrin receptor-targeted liposomal doxorubicin in P-glycoprotein-mediated drug resistant tumor cells. Int J Pharm 329:94–102
- Kuo YC, Lin PI, Wang CC. (2011). Targeting nevirapine delivery across human brain microvascular endothelial cells using transferrin-grafted poly(lactide-co-glycolide) nanoparticles. Nanomedicine (Lond) 6:1011–26
- Kutty RV, Chia SL, Setyawati MI, et al. (2015). In vivo and ex vivo proofs of concept that cetuximab conjugated vitamin E TPGS micelles increases efficacy of delivered docetaxel against triple negative breast cancer. Biomaterials 63:58–69
- Li XM, Ding LY, Xu Y, et al. (2009). Targeted delivery of doxorubicin using stealth liposomes modified with transferrin. Int J Pharm 373:116–23
- Li Y, He H, Jia X, et al. (2012). A dual-targeting nanocarrier based on poly(amidoamine) dendrimers conjugated with transferrin and tamoxifen for treating brain gliomas. Biomaterials 33:3899–908
- Liu Y, Li K, Pan J, et al. (2010). Folic acid conjugated nanoparticles of mixed lipid monolayer shell and biodegradable polymer core for targeted delivery of docetaxel. Biomaterials 31:330–8
- Muthu MS, Singh S. (2009). Targeted nanomedicines: effective treatment modalities for cancer, AIDS and brain disorders. Nanomedicine (Lond) 4:105–18
- Muthu MS, Kulkarni SA, Xiong J, Feng SS. (2011). Vitamin E TPGS coated liposomes enhanced cellular uptake and cytotoxicity of docetaxel in brain cancer cells. Int J Pharm 421:332–40
- Muthu MS, Wilson B. (2012). Challenges posed by the scale-up of nanomedicines. Nanomedicine 7:307–9
- Muthu MS, Kulkarni SA, Raju A, Feng SS. (2012a). Theranostic liposomes of TPGS coating for targeted co-delivery of docetaxel and quantum dots. Biomaterials 33:3494–501
- Muthu MS, Kulkarni SA, Liu Y, Feng SS. (2012b). Development of docetaxel-loaded vitamin E TPGS micelles: formulation optimization, effects on brain cancer cells and biodistribution in rats. Nanomedicine (Lond) 7:353–64
- Muthu MS, Feng SS. (2013). Theranostic liposomes for cancer diagnosis and treatment: current development and pre-clinical success Expert Opin. Drug Deliv 10:151–5
- Muthu MS, Leong DT, Mei L, Feng SS. (2014a). Nanotheranostics - application and further development of nanomedicine strategies for advanced theranostics. Theranostics 4:660–77
- Muthu MS, Mei L, Feng SS. (2014b). Nanotheranostics: advanced nanomedicine for the integration of diagnosis and therapy. Nanomedicine 9:1277–80
- Muthu MS, Kutty RV, Luo Z, et al. (2015). Theranostic vitamin E TPGS micelles of transferrin conjugation for targeted co-delivery of docetaxel and ultra bright gold nanoclusters. Biomaterials 39:234–48
- Muthu MS, Sahu AK. Sonali, et al. (2016). Solubilized delivery of paliperidone palmitate by d-alpha-tocopheryl polyethylene glycol 1000 succinate micelles for improved short-term psychotic management. Drug Deliv 23:230–7
- Ni D, Zhang J, Bu W, et al. (2014). Dual-targeting upconversion nanoprobes across the blood-brain barrier for magnetic resonance/fluorescence imaging of intracranial glioblastoma. ACS Nano 8:1231–42
- Raju A, Muthu MS, Feng SS. (2013). Trastuzumab-conjugated Vitamin E TPGS liposomes for sustained and targeted delivery of docetaxel. Expert Opin Drug Deliv 10:747–60
- Schnyder A, Huwyler J. (2005). Drug transport to brain with targeted liposomes. NeuroRx 2:99–107
- Singh RP, Sharma G. Sonali, et al. (2016). Transferrin receptor targeted PLA-TPGS micelles improved efficacy and safety in docetaxel delivery. Int J Biol Macromol 83:335–44
- Sonali, Aggrawal P, Singh RP, et al. (2015). Transferrin receptor targeted Vitamin E TPGS micelles for brain cancer therapy: preparation, characterization and brain distribution in rats. Drug Deliv. [Epub ahead of print]. DOI: 10.3109/10717544.2015.1094681
- Song Q, Tan S, Zhuang X, et al. (2014). Nitric oxide releasing D-α-tocopheryl polyethylene glycol succinate for enhancing antitumor activity of doxorubicin. Mol Pharm 11:4118–29
- Ulbrich K, Hekmatara T, Herbert E, Kreuter J. (2009). Transferrin- and transferrin-receptor-antibody-modified nanoparticles enable drug delivery across the blood–brain barrier (BBB). Eur J Pharm Biopharm 71:251–6
- Vijayakumar MR, Muthu MS, Singh S. (2013). Co-polymers of poly (lactic acid) and D-α-tocopheryl polyethylene glycol 1000 succinate based nanomedicines: versatile multifunctional platforms for cancer diagnosis and therapy. Expert Opinion on Drug Delivery 10:529–43
- Weng KC, Noble CO, Sternberg BP, et al. (2008). Targeted tumor cell internalization and imaging of multifunctional quantum dot-conjugated immunoliposomes in vitro and in vivo. Nano Lett 8:2851–7
- Wen CJ, Zhang LW, Al-Suwayeh SA, et al. (2012). Theranostic liposomes loaded with quantum dots and apomorphine for brain targeting and bioimaging. Int J Nanomedicine 7:1599–611
- Wen CJ, Sung CT, Aljuffali IA, et al. (2013). Nanocomposite liposomes containing quantum dots and anticancer drugs for bioimaging and therapeutic delivery: a comparison of cationic, PEGylated and deformable liposomes. Nanotechnology 24:1–13
- Wilson B, Samanta MK, Santhi K, et al. (2008). Targeted delivery of tacrine into the brain with polysorbate 80-coated poly(n-butylcyanoacrylate) nanoparticles. Eur J Pharm Biopharm 70:75–84
- Xu Q, Liu Y, Su S, et al. (2012). Anti-tumor activity of paclitaxel through dual-targeting carrier of cyclic RGD and transferrin conjugated hyperbranched copolymer nanoparticles. Biomaterials 33:1627–39
- Zhang Z, Tan S, Feng SS. (2012). Vitamin E TPGS as a molecular biomaterial for drug delivery. Biomaterials 33:4889–906
- Zhao J, Mi Y, Feng SS. (2013). Targeted co-delivery of docetaxel and siPlk1 by herceptin-conjugated vitamin E TPGS based immunomicelles. Biomaterials 34:3411–21
- Zhao L, Feng SS. (2010). Enhanced oral bioavailability of paclitaxel formulated in vitamin E-TPGS emulsified nanoparticles of biodegradable polymers: in vitro and in vivo studies. J Pharm Sci 99:3552–60
- Zong T, Mei L, Gao H, et al. (2014). Synergistic dual-ligand doxorubicin liposomesImprove targeting and therapeutic efficacy of brain glioma in animals. Mol Pharm 11:2346–57