Abstract
Two new amperometric carbon paste enzyme electrodes including Fe3O4 nanoparticles with and without 1,4-benzoquinone were developed for glucose determination. Electron transfer properties of unmodified and Fe3O4 nanoparticles and/or 1,4-benzoquinone modified carbon paste electrodes were investigated in 0.1 M KCl support electrolyte containing Fe(CN)63−/4− as redox probe by cyclic voltammetry (CV) and electrochemical impedance spectroscopy (EIS) methods. Fe3O4 nanoparticles increased electron transfer at solution/electrode interface. The parameters affecting the analytical performance of the enzyme electrode have been investigated in detail and optimized for Fe3O4 nanoparticle modified enzyme electrode (Fe3O4–CPEE). Fe3O4 nanoparticles and 1,4-benzoquinone modified enzyme electrode (BQ‐Fe3O4‐CPEE) exhibited linear response from 1.9 × 10‐7 M to 3.7 × 10‐6 M, from 7.2 × 10‐6 M to 1.5 × 10‐4 M and from 1.3 × 10‐3 M to 1.2 × 10‐2 M with an excellent detection limit of 1.9 × 10‐8 M. BQ‐Fe3O4‐CPEE was used for determination of glucose in serum samples and results were in good agreement with those obtained by spectrophotometric method.
Introduction
Accurate, rapid, sensitive and simple determination of glucose is very important in biochemistry, clinical diagnostics, food industry and many different areas. Especially in clinical diagnostics, the concentration of glucose is used as an indicator for diabetes. Diabetes represents a serious worldwide public health problem and it leads to severe damage mainly in kidneys, heart and eyes (Lin, He, Zhao, & Zhang, 2009; Comba et al., 2010). Therefore, many techniques including colorimetry (Kabasakalian, Kalliney, & Westcott, 1974), gas chromatography-mass spectrometry (Di Gioia et al., 2004), chemiluminescence (Lv, Zhang, & Chen, 2003), voltammetry (Zhu, Lu, Li, Shao, & Zhu, 2009), liquid chromatography (Coquet, Veuthey, Haerdi, & Agosti, 1991) and spectrophotometry (Zaitoun, 2006) were reported for glucose quantification. However, these methods are usually laborious, expensive, time-consuming and/or complex to perform. An alternative method for glucose determination is the use of electrochemical enzyme electrodes, which allow direct, rapid, simple and low-cost measurement of glucose. Various types of enzyme electrodes have been reported for glucose determination since the work of Clark and Lyons in 1962 (Clark & Lyons, 1962; Saito & Watanabe, 1998; Tang et al., 2004; Cui et al., 2001).
The enzyme glucose oxidase (GOD) has been the most widely used biorecognition element in glucose enzyme electrodes. GOD catalyzes the oxidation of glucose to gluconolactone and reduces the natural mediator, oxygen, to hydrogen peroxide. H2O2 formed during this reaction can be determined with amperometric sensors (Yang, Ren, Tang, & Zhang, 2009; Ali, Nur, Willander, & Danielsson, 2010).
Unfortunately, the amperometric determination of H2O2 requires high anodic potential. In this case, other electroactive species present in the sample such as ascorbic acid acetaminophen and uric acid can also be oxidized and interfere with the analysis. This drawback can be eliminated by designing electrochemical glucose biosensors working at lower potentials. In glucose biosensors, redox-active species (mediators) can be used as artificial electron acceptors for FADH2 in place of O2. With the use of the right mediator, it is not necessary to apply a high potential to the electrode since mediators can be oxidized at potentials slightly higher than their redox potentials (Saito & Watanabe, 1998).
Carbon paste electrodes have been widely used for electroanalytical applications since their introduction by Adams in 1958. These electrodes offer the advantages of low background current, low cost of fabrication and ease of modification (Švancara, Vytas, Barek, & Zima, 2001). A number of glucose biosensors based on carbon paste matrix have been reported (Wang & Walcarius, 1996; Liu, Lu, & Wang, 1999; Wang, Mo, Li, & Porter, 2001). The use of redox mediators in carbon paste electrodes is a promising approach (Yang et al., 2004; Koyuncu, Erden, Pekyardimci, & Kiliç, 2007). Especially, quinones are one of the most commonly used mediators in mediated biosensors (Yang et al., 2004; Casero, Darder, Pariente, & Lorenzo, 2000).
Recently, applications of nanomaterials in biosensors have aroused much interest. These interesting materials exhibit desirable properties such as large surface-to-volume ratio, high surface reaction activity, high catalytic efficiency and strong adsorption ability that are useful in biosensing applications. Nanomaterials have the unique ability of promoting fast electron transfer between electrode and the active site of the enzyme. Among the various nanomaterials, magnetite nanoparticles have recently gained more interest due to biocompatibility, strong superparamagnetic property and low toxicity (Kaushik et al., 2009). Glucose biosensors based on magnetite nanoparticles have been reported (Kaushik et al., 2008; Baby & Ramaprabhu, 2010). However, preparation of a glucose biosensor based on magnetite nanoparticles and 1,4-benzoquionone, to our best knowledge have not been reported.
In this work, we developed simple and low-cost enzyme electrodes working at low potentials and performed reliable glucose analysis in real samples. We constructed two different modified carbon paste enzyme electrodes by incorporating Fe3O4 nanoparticles or Fe3O4 nanoparticles and 1, 4-benzoquinone with glucose oxidase within a carbon paste matrix. We investigated; the parameters that influence the electrode performance and the analytical characteristics for Fe3O4 nanoparticles modified enzyme electrode. Analytical characteristics, operational stability, interference effect and application of Fe3O4 nanoparticles and 1,4-benzoquinone modified enzyme electrode to real samples were also investigated.
Experimental
Equipment and reagents
The electrochemical studies were carried out with IVIUM electrochemical analyzer (www.ivium.nl) and a three- electrode cell stand (www.basinc.com). The working electrode was a modified carbon paste electrode. The counter and the reference electrodes were a Pt wire (BAS MW 1034) and Ag/AgCl (BAS MF 2052) electrode, respectively (www.basinc.com). The pH values of the buffer solutions were measured with ORION Model 720A pH/ion meter and ORION combined pH electrode (www.thermoscientific.com).
Glucose oxidase (E.C. 1.1.3.4 from Aspergillus niger sp. with a specific activity of 39 Units/mg solid), Fe3O4 nanoparticles, uric acid, ascorbic acid and glutaraldehyde were purchased from Sigma (www.sigmaaldrich.com). Sodium monohydrogenphosphate and sodium dihydrogenphosphate were supplied from Riedel-de Haën (www.riedeldehaen.com). 1,4-Benzoquinone, bovine serum albumin (BSA), graphite powder, paraffin oil and glucose were from Fluka (www.sigmaaldrich.com). All other chemicals were obtained from Merck (www.merckgroup.com). Standard solutions of glucose were prepared by dissolving glucose in phosphate buffer solution and allowed to mutarotate at room temperature for 24 hours before use. The standard glucose solutions were prepared freshly every day. All the measurements were carried out at room temperature.
Preparation of carbon paste and modified carbon paste electrodes
Carbon paste was prepared in the following proportions for unmodified electrode (UCPE): 77.64% graphite powder and 22.36% paraffin oil. Fe3O4 nanoparticles modified carbon paste electrode (Fe3O4‐CPE) was composed of 59.52% graphite powder, 18.12% Fe3O4 nanoparticles and 22.36% paraffin oil. 1,4-Benzoquinone modified electrode was composed of 64.48% graphite powder, 13.16% 1,4 benzoquinone and 22.36% paraffin oil (BQ‐CPE). Fe3O4 nanoparticles and 1,4-benzoquinone modified carbon paste (BQ‐Fe3O4‐CPE) was composed of 46.58% graphite powder, 18.12% Fe3O4 nanoparticles, 12.94% 1,4-benzoquinone and 22.36% paraffin oil. The modified electrodes were prepared by hand-mixing graphite powder with the nanoparticles and then adding paraffin oil and thoroughly mixing for approximately 20 minutes to form homogeneous modified carbon paste electrodes.
Graphite powder and Fe3O4 nanoparticles were mixed and enzyme solution (25 μL glucose oxidase (10 Unit), 1.5 mg BSA and 25 μL 1.25% glutaraldehyde) was added for Fe3O4 nanoparticles modified enzyme electrode (Fe3O4‐CPEE). Paraffin oil was added after the evaporation of water and mixed for approximately 20 minutes until a uniform paste was obtained. Fe3O4 nanoparticles and 1,4-benzoquinone modified enzyme electrode (BQ‐Fe3O4‐CPEE) was also prepared in a similar way. In all cases, the paste was placed into the bottom of the working electrode body and the electrode surface was polished with a smooth paper layer to have a smooth surface. The electrodes were washed with distillated water and working buffer between measurements. Electrodes were stored in refrigerator at +4°C when not in use.
Amperometric measurements
Electron transfer properties of unmodified and modified electrodes were determined in 0.1 M KCl solution containing 1 mM K3[(Fe(CN)6] + 1 mM K4[(Fe(CN)6] by Cyclic Voltammetry (CV) and Electrochemical Impedance Spectroscopy (EIS). The cyclic voltammograms of unmodified carbon paste electrode (UCPE) and Fe3O4‐CPE were recorded at (‐0.2)V‐(+ 0.6)V, BQ‐CPE at (‐0.25)V‐(+ 0.6)V and BQ‐Fe3O4‐CPE at (‐0.3)V‐(+ 0.6)V. EIS measurements were performed at the frequency range of 105‐ 10‐2 Hz with 5 mV amplitude under open circuit potential (EOCP) conditions.
All other amperometric measurements were performed in phosphate buffer solution (0.05 M pH 7.5). The electrochemical response of the UCPE to H2O2 was investigated first. 5.0 mL buffer solution was added to the cell. After the application of + 0.30 V potential, the background current was allowed to decay to constant value. Then an aliquot of 10‐2 M H2O2 stock solution was added to the cell and the solution was purged with nitrogen and stirred prior to each measurement. The current values against H2O2 concentrations were plotted in order to determine the sensitivity of the electrode to H2O2. The same experiment was performed with Fe3O4‐CPE and BQ‐Fe3O4‐CPE.
Determination of glucose in human serum samples
Standard addition method was used to determine the glucose concentration in human serum. Serum samples were mixed with 10% trichloroacetic acid (TCA) for deproteinization. After a 10-minute centrifugation at 3 000 rpm, supernatants were filtered and diluted. BQ‐Fe3O4‐CPEE was placed in the cell containing 5.0 mL buffer and after the stabilization of background current, 10 μL sample was added. The solution was purged and stirred. The response of the electrode against glucose was measured in oxygen-saturated medium at +0.30 V. After the current reached a steady-state value, increasing amounts of standard glucose solution were added to this solution and standard addition curve was plotted. The glucose concentration of the serum sample was calculated from this curve.
Results and discussion
Electrochemical Characterization of Unmodified and Modified CPEs
CV of the ferricyanide system is a convenient and valuable tool to monitor the characteristics of the surface modified electrodes. The electron transfer properties of UCPE, Fe3O4‐CPE, BQ‐CPE and BQ‐Fe3O4‐CPE were investigated in 0.1 M KCl solution containing 1 mM Fe(CN)63−/4− at 10 mVs‐1. The cyclic voltammograms are shown in . While the well-defined redox peaks of Fe(CN)63−/4− were observed for UCPE and Fe3O4-CPE, redox peaks of BQ/H2BQ (about ‐0.08 V and ‐0.17 V) were present for BQ‐CPE and BQ‐Fe3O4‐CPE in addition to redox peaks of Fe(CN)63−/4−. Fe3O4 nanoparticles increased current intensity and decreased peak-to-peak separation (Ep,a‐Ep,c = ΔEp) for Fe(CN)63−/4− waves. After the modification of electrode with BQ, no significant change was observed in current intensity and peak-to-peak separation compared to UCPE. The ΔEp for UCPE, Fe3O4‐CPE, BQ‐CPE and BQ‐Fe3O4‐CPE were 280 mV, 170 mV, 290 mV and 250 mV, respectively. The lower ΔEp value for Fe3O4‐CPE indicates that electron transfer of this electrode is faster than the other electrodes (Yang, Ren, Tang, & Zhang, 2009; Yao & Shiu, 2007; Lu & Chen, 2006; Lin et al., 2008). Lu and Chen revealed that nano-Fe3O4 promoted electron transfer between the electroactive Fe3+/Fe2+ species and electrode surface (Lu & Chen, 2006). Yang et al. reported that Fe3O4 nanoparticles increased the current response of Fe3+/Fe2+ redox couples, indicating the enhancing effect of the Fe3O4 nanoparticles on the electric conductivity of the electrode (Yang, Ren, Tang, & Zhang, 2009). These results confirm our findings. The electrochemical surface area of Fe3O4‐CPE was calculated from the voltammetric peak current by using Randles – Sevcik equation to support the experimental results and to investigate the effect of Fe3O4 on the electrochemical surface area of the electrode (Yao & Shiu, 2007; Wang, 2006; Ding, Chang, Wu, Lai, & Chang, 2005). According to the Randles - Sevcik equation, the peak current is directly proportional to the concentration in bulk solution and increases with the square root of the scan rate. shows the peak current versus the square root of the scan rate and cyclic voltammograms for Fe(CN)63‐/4‐ redox probe at scan rates ranging from 10 to 150 mVs −1 (inset in ) for Fe3O4‐CPE. The peak potential shifts slightly while the peak current increases with increasing the scan rate. The effective electrochemical surface area of Fe3O4‐CPE is calculated by using the slope of the curve. The effective electrochemical surface area of Fe3O4‐CPE (0.125 cm2) is much higher than the surface area of the UCPE (0.071 cm2). The curve is linear, which suggests that the mass transfer phenomenon in the double layer region of the electrode is mainly controlled by diffusion (Song et al., 2010; Du, Li, Mei, & Liu, 2009; Khan et al., 2008).
Figure 1. (A) Cyclic voltammograms of (a) UCPE, (b) Fe3O4-CPE, (c) BQ-CPE and (d) BQ-Fe3O4-CPE at 10 mVs‐1, (B) curve of the anodic peak current versus the square root of the scan rate for Fe3O4-CPE (inset: cyclic voltammograms at scan rates of 10, 25, 50, 75, 100 and 150 mVs−1) in 0.1 M KCl solution containing 1 mM Fe(CN)63−/4−.
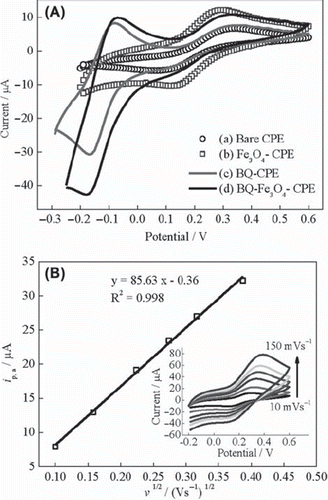
EIS is an effective method to investigate the electrical properties of modified electrodes and to determine electrochemical reaction rates (Wang, 2006). shows electrochemical impedance spectra, Nyquist curves, of UCPE, Fe3O4‐CPE, BQ‐CPE and BQ‐Fe3O4‐CPE. The Nyquist curves include a depressed semicircle portion and a linear portion. The semicircle portion at high frequencies corresponds to the electron transfer limited process, and the linear portion at low frequencies corresponds to the diffusion process. The diameter of the semicircles is equal to the electron transfer resistance (Rct) value (Wang, 2006; Liu et al., 2006). The Rct values for UCPE, Fe3O4‐CPE, BQ‐CPE and BQ‐Fe3O4‐CPE are approximately 10.8, 2.0, 11.7 and 3.1 k蒆, respectively. The results indicate that while Fe3O4 nanoparticles dramatically increase electron transfer at solution/electrode interface, BQ slightly decreases the electron transfer (Yang, Ren, Tang, & Zhang, 2009; Lu & Chen, 2006). However, Rct values of Fe3O4‐CPE and BQ‐Fe3O4‐CPE are lower than that of UCPE, so these two modified electrodes promise better results in the preparation of enzyme electrodes.
Response characteristics of CPEs and CPEEs
The quantification of glucose is based on the electrochemical detection of enzymatically generated H2O2 in oxygen-saturated medium, thus the sensor sensitivity is dependent on the electrochemical response of the electrode to H2O2. Electrodes with high catalytic efficiency to H2O2 would have high sensitivity to glucose. shows the current difference values versus H2O2 concentration obtained with UCPE, Fe3O4–CPE, BQ–CPE and BQ‐Fe3O4‐CPE in nitrogen-saturated solution. The sensitivities of the Fe3O4‐CPE, BQ‐CPE and BQ‐Fe3O4‐CPE are higher than that of unmodified carbon paste electrode to H2O2. The highest sensitivity was obtained with BQ‐Fe3O4‐CPE. This can be explained by the synergistic action of the electrocatalytic activity of 1,4-benzoquinone and Fe3O4.
Figure 3. (A) Calibration curves for H2O2: (a) BQ‐Fe3O4‐CPE, (b) Fe3O4‐CPE (c) BQ‐CPE and (d)UCPE (0.05 M pH 7.5 phosphate buffer, + 0.30 V, nitrogen-saturated solution). (B) Calibration curves for glucose: (a) BQ‐Fe3O4‐CPEE, (b) Fe3O4‐CPEE (c) BQ‐CPEE and (d) UCPEE (0.05 M pH 7.5 phosphate buffer, + 0.30 V, oxygen-saturated solution).
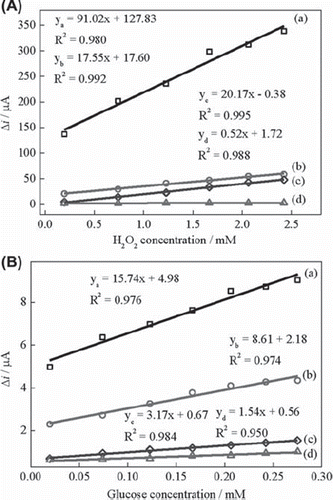
The response of UCPEE, Fe3O4‐CPEE, BQ‐CPEE and BQ‐Fe3O4‐CPEE to glucose was also determined at + 0.30 V in oxygen-saturated solution (). The sensitivities of the Fe3O4‐CPEE and BQ‐Fe3O4‐CPEE are higher than that of unmodified carbon paste electrode similar to H2O2 responses. We investigated the glucose response of different enzyme electrodes in nitrogen-saturated buffer solution and compared these results with those obtained in oxygen-saturated solution to clarify the detection mechanism of glucose. UCPEE and Fe3O4‐CPEE showed very small catalytic activity toward glucose in nitrogen-saturated solution in contrast to the response in oxygen-saturated solution. This indicates that Fe3O4 nanoparticles cannot catalyze the electron transfer between the reduced enzyme and electrode surface efficiently. BQ‐CPEE and BQ‐Fe3O4‐CPEE exhibited good response to glucose both in nitrogen and in oxygen-saturated solutions. However, the sensitivities of the electrodes were higher in oxygen-saturated solution, indicating the synergistic action of the electrocatalytic activity of 1,4-benzoquinone and oxygen. This is a clear indication of 1,4-benzoquinone acting as a mediator for glucose determination. 1,4-Benzoquinone reduces to hydroquinone (H2BQ) and hydroquinone is also reoxidized to 1,4-benzoquinone on carbon paste electrode surface at + 0.30 V. Our results are in good agreement with the data reported (Yang et al., 2004). Consequently, possible electron transfer behavior of the BQ‐Fe3O4‐CPEE may occur as following:
The detection mechanism is based on equations (1), (3) and (5) in nitrogen-saturated solution and (1), (2), (3), (4) and (5) in oxygen-saturated solution. It can be suggested that the mechanism of glucose determination is based on the electrochemical oxidation of H2O2 and H2BQ in oxygen-saturated solution. However, in nitrogen-saturated solution only electrochemical oxidation of H2BQ occurs. Furthermore, the responses of the UCPEE and Fe3O4‐CPEE in nitrogen-saturated solution shows that the detection mechanism is not based on the direct electrochemistry of glucose oxidase. In this study, the highest sensitivity was obtained with BQ‐Fe3O4‐CPEE () which may be attributed to the fact that Fe3O4 nanoparticles increases the surface area and electric conductivity of the electrodes thus enhancing the sensitivity of the electrode.
Optimum working conditions and electrode composition of Fe3O4-CPEE
The amount of enzyme loading can affect the amperometric response of an enzyme electrode. Therefore, it is important to determine the enzyme amount in carbon paste matrix. The responses of Fe3O4‐CPEE were measured at five different enzyme amounts by keeping the other conditions constant and varying the glucose oxidase amount between 4 and 12 Units. Gradual increase in the current differences was observed as the amount of glucose oxidase increased. However, over 10 Unit of enzyme, the response current tends to be saturated. The optimum enzyme loading was specified as 10 Unit because further increase of enzyme loading would be a waste of this expensive reagent.
Investigation of the effect of pH on the response of the enzyme electrode is of great importance, since the activity of the immobilized GOD is pH dependent (Wang et al., 2009). The pH dependence of the Fe3O4‐CPEE response was evaluated at 0.09 mM glucose concentration over the pH range from 6 to 9 (). The response current increases from pH 6 to 7.5 and then decreases with increasing pH. The proton is critical in the redox behavior of GOD-FAD. The decrease of the response at high pH values may be due to the decrease of proton concentration and activity of the immobilized GOD (Yang, Ren, Tang, & Zhang, 2009). The highest response was obtained at pH 7.5 as shown in . Therefore, pH 7.5 was selected as the optimum pH and all the following measurements were performed at this pH. Good responses for free enzyme have been reported in the pH range of 4–7 (Tang et al., 2004). The results obtained from pH study indicate that immobilization procedure has a little influence on the properties of glucose oxidase. Different pH values such as 6.0 (Yu, Yu, Zhao, & Zeng, 2008; Che et al., 2010), pH 6.5 (Wang, Wang, Di, & Tu, 2008), pH 7.0 (Wang et al., 2009; Xue, Xu, Zhou, & Zhu, 2006), pH 7.2 (Yang, Ren, Tang, & Zhang, 2009), pH 7.4 (Tang et al., 2004), pH 8.0 (Chuang, Wang, & Lan, 1997), pH 9.0 (Luo et al., 2010) were also reported in the literature as optimum pH for glucose enzyme electrodes. The difference of the optimum reported pH values may be attributed to different mediators, enzyme supplies, immobilization methods or electrode preparation procedures. The amperometric response of Fe3O4‐CPEE was determined at different phosphate concentrations of 0.05 M, 0.10 M, 0.15 M and 0.20 M and the best response for Fe3O4‐CPEE was obtained at 0.05 M. Above this concentration, the current difference was found to show a significant decrease. The optimized parameters were also used for the BQ‐Fe3O4‐CPEE to compare the performance of the electrodes. However, we checked the optimum working pH also for BQ‐Fe3O4‐CPEE and the maximum response was obtained at pH 7.5 similar to the Fe3O4‐CPEE.
Figure 4. The effect of buffer pH on the response of Fe3O4‐CPEE (0.05 M phosphate buffer, + 0.30 V).
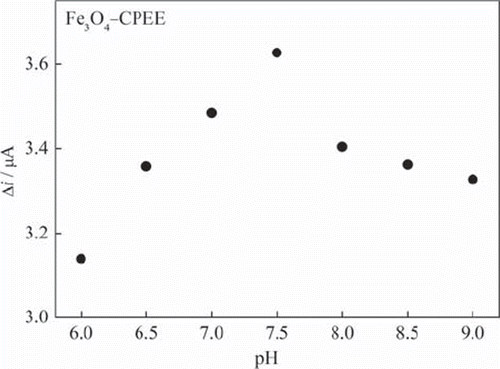
The response of Fe3O4‐CPEE to constant glucose concentration (0.09 mM) was determined at different working potentials between (0) ‐ (+ 0.70) V. Potentials higher than + 0.70 V were not investigated due to the increasing risk of interference effects. Amperometric current was increased gradually for Fe3O4‐CPEE in the range from (+ 0.20) to (+ 0.70) V. Although the maximum current difference was obtained at + 0.70 V, + 0.30 V was selected for the amperometric determination of glucose since we obtained sufficient response and calibration curves with good linearity. This working potential also could minimize the risk for interfering reactions of other oxidizable species in real samples.
Performance parameters of Fe3O4‐CPEE and BQ‐Fe3O4‐CPEE
The response time of the enzyme electrode depends on the glucose concentration. The amperometric response times of Fe3O4‐CPEE and BQ‐Fe3O4‐CPEE for glucose were determined at two different glucose concentrations. The current differences for 1.0 × 10‐5 M and 1.0 × 10‐4 M glucose versus time were plotted. The response time was shorter at lower concentrations than at higher concentrations. The current reached 95% of the steady-state current within about 120 seconds (t95) for Fe3O4‐CPEE and 100 seconds (t95) for BQ‐Fe3O4‐CPEE. The shorter response time of BQ‐Fe3O4‐CPEE may be attributed to the faster electron transfer feature of nanoparticle-mediator composite.
The repeatability of Fe3O4‐CPEE and BQ‐Fe3O4‐CPEE was also investigated. Five calibration curves were plotted by the use of the same electrodes sequentially. The relative standard deviation of the sensitivities was 2.8% for Fe3O4‐CPEE and 4.1% for BQ‐Fe3O4‐CPEE.
We checked the long-term stability of BQ‐Fe3O4‐CPEE prepared under optimum conditions. The electrode was stored in dry atmosphere at + 4°C when not in use. The BQ‐Fe3O4‐CPEE lost only 4.5% of its initial sensitivity after 21 days. This shows that Fe3O4 nanoparticles and 1,4- benzoquinone ensure the stability of the enzyme electrode. The stability of the BQ‐Fe3O4‐CPEE electrode is much better compared to the stability of another carbon material electrode with a 26.5% loss of response after 22 days. (Tang et al., 2004).
shows the amperometric response curve of the Fe3O4‐CPEE recorded as a function of glucose concentration under optimized experimental conditions. The response current increases with increasing concentration of glucose up to 7.3 × 10‐4 M. The limit of detection is 1.9 × 10‐7 M. The curve is composed of three linear parts ranging from 1.9 × 10‐7 M to 1.9 × 10−6 M, from 3.8 × 10‐6 M to 5.2 × 10‐5 M and from 8.3 × 10‐5 M to 7.3 × 10‐4 M.
Figure 5. (A) Effect of glucose concentration on the response of Fe3O4‐CPEE (B) Effect of glucose concentration on the response of BQ‐Fe3O4‐CPEE (inset: glucose response of the BQ‐Fe3O4‐CPEE at low concentrations) (0.05 M, pH 7.5 phosphate buffer, + 0.30 V, oxygen-saturated solution).
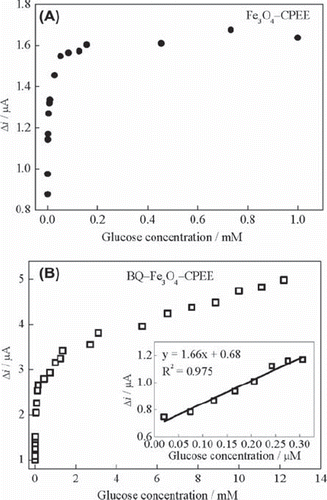
The relationship between glucose concentration and response current of the BQ‐Fe3O4‐CPEE is shown in . The BQ‐Fe3O4‐CPEE exhibited a linear response in the range from 1.9 × 10‐7 M to 3.7 × 10‐6 M, from 7.2 × 10‐6 M to 1.5 × 10‐4 M and from 1.3 × 10‐3 M to 1.2 × 10‐2 M. The limit of detection is 1.9 × 10–7 M. The linear working range of BQ‐Fe3O4‐CPEE is wider than that of Fe3O4‐CPEE. This may be attributed to the synergetic effect of Fe3O4 nanoparticles and 1,4-benzoquinone. We also investigated the response of BQ‐Fe3O4‐CPEE at lower glucose concentrations. The enzyme electrode showed linear response between 1.9 × 10‐8 M‐ 3.1 × 10‐6 M ( inset). This response at lower concentrations is important when working with dilute samples to eliminate the effects of interferences. It can be concluded that GOD (FADH2) can react with O2 and BQ at different concentrations of glucose as clearly indicated from the three ranges of linearity of the calibration curves. This study show that the use of BQ and Fe3O4 together as a modifier in CPEE's improves the detection limit and linear working range remarkably compared to other magnetite-nanoparticle-modified glucose biosensors recently reported (Yang, Ren, Tang, & Zhang, 2009; Kaushik et al., 2008; Li, Wei, & Yuan, 2009).
The oxidizable compounds such as uric acid, urea, glucose, creatinine and ascorbic acid can interfere with the amperometric measurement of enzyme electrodes. In our study, the effect of interferences was evaluated for two common interfering substances, namely ascorbic acid and uric acid, normally present in human serum and urine. Interference effect was only determined for BQ‐Fe3O4‐CPEE. Addition of 0.3 mM ascorbic acid and 0.3 mM uric acid to 0.3 mM glucose increased the response current by about 65% and 38%, respectively. However, when the concentration of ascorbic acid and uric acid decreased to 2 μM the interference effect also decreased to 2% and 7%, respectively. Therefore, it can be concluded that the response to glucose at + 0.30 V was not affected by addition of 2 μM ascorbic acid significantly and dilution reduces the effect of interferences as it was reported in the literature (Martinez‐Peréz, Ferrer, & Mateo, 2003). In this study, we performed the real sample measurements in the range of 5.4 × 10‐7 M‐ 9.8 × 10‐7 M and in this range, we did not observe a significant effect of interferences.
Determination of glucose in serum
In , results obtained from four serum samples by using BQ‐Fe3O4‐CPEE are presented together with those obtained from spectrophotometric technique. Results are in good agreement and show that BQ‐Fe3O4‐CPEE can be successfully used for the detection of glucose concentration in serum samples. We checked the accuracy of the method by t-test. The t value is 0.33 for BQ‐Fe3O4‐CPEE at 95% confidence level, for which tcritic is 3.18 (Skoog, West, Holler, & Crouch, 2004). It is clear from the t-test that there is no difference between the results of two methods at a confidence level of 95%.
Table 1. Comparison of glucose concentration in serum samples using the modified enzyme electrode and the spectrophotometric method.
Conclusion
In this study, we have presented the use of magnetite nanoparticles and 1,4-benzoquinone together in electrochemical enzyme electrodes for the first time. The BQ‐Fe3O4‐CPEE demonstrated an excellent catalytic activity toward glucose determination through the synergetic action of magnetite nanoparticles and 1,4-benzoquinone. It was shown that the mechanism of glucose determination is based on the electrochemical oxidation of H2O2 and H2BQ in oxygen-saturated medium and only electrochemical oxidation of H2BQ in nitrogen-saturated solution. 1,4-benzoquinone acted as a mediator and magnetite nanoparticles increased electric conductivity and surface area of the electrode. BQ‐Fe3O4‐CPEE provided the determination of glucose at a low potential (+ 0.30 V) and low concentration range hence reducing the risk of interference. It can be concluded that BQ‐Fe3O4‐CPEE shows promise for glucose determination in real samples. The incorporation of magnetite nanoparticles with different mediators can be extended to other enzyme-based biosensors.
Declaration of interest
The authors report no conflicts of interest. The authors alone are responsible for the content and writing of the article.
References
- Ali SMU, Nur O, Willander M, Danielsson B. 2010. A fast and sensitive potentiometric glucose microsensor based on glucose oxidase coated ZnO nanowires grown on a thin silver wire. Sensor Actuat B-Chem. 145:869–874.
- Baby TT, Ramaprabhu S. 2010. SiO2 coated Fe3O4 magnetic nanoparticle dispersed multiwalled carbon nanotubes based amperometric glucose biosensor. Talanta. 80:2016–2022.
- Casero E, Darder M, Pariente F, Lorenzo E. 2000. Peroxidase enzyme electrodes as nitric oxide biosensors. Anal Chim Acta. 403:1–9.
- Che X, Yuan R, Chai Y, Li J, Song Z, Li W. 2010. Amperometric glucose biosensor based on prussian blue-multiwall carbon nanotubes composite and hollow PtCo nanochains. Electrochim Acta. 55:5420–5427.
- Chuang C L, Wang YJ, Lan HL. 1997. Amperometric glucose sensors based on ferrocene-containing B-polyethylenimine and immobilized glucose oxidase. Anal Chim Acta. 353:37–44.
- Clark LC, Lyons JC. 1962. Electrode system for continuous monitoring in cardiovascular surgery. Ann N Y Acad Sci. 102:29–45.
- Comba FN, Rubianes MD, Cabrera L, Gutie'rrez S, Herrasti P, Rivasa GA. 2010. Highly sensitive and selective glucose biosensing at carbon paste electrodes modified with electrogenerated magnetite nanoparticles and glucose oxidase. Electroanal. 22:1566–1572.
- Coquet A, Veuthey JL, Haerdi W, Agosti RD. 1991. Applications of a post-column fluorigenic reaction in liquid chromatography for the determination of glucose and fructose in biological matrices. Anal Chim Acta. 252:173–179.
- Cui G, Yoo JH, Woo BW, Kim SS, Cha GS, Nam H. 2001. Disposable amperometric glucose sensor electrode with enzyme-immobilized nitrocellulose strip. Talanta. 54:1105–1111.
- Di Gioia ML, Leggio A, Le Pera A, Liguori A, Napoli A, Siciliano C, Sindona G. 2004. Quantitative analysis of human salivary glucose by gas chromatography–mass spectrometry. J Chromatogr. 801:355–358.
- Ding SJ, Chang BW, Wu CC, Lai MF, Chang HC. 2005. Impedance spectral studies of self-assembly of alkanethiols with different chain lengths using different immobilization strategies on Au electrodes. Anal Chim Acta. 554:43–51.
- Du P, Li H, Mei Z, Liu S. 2009. Electrochemical DNA biosensor for the detection of DNA hybridization with the amplification of Au nanoparticles and CdS nanoparticles. Bioelectrochemistry. 75:37–43.
- Kabasakalian P, Kalliney S, Westcott A. 1974. Enzymatic blood glucose determination by colorimetry of N,N-diethylaniline-4-aminoantipyrine. Clin Chem. 20:606–607.
- Kaushik A, Khan R, Solanki PR, Pandey P, Alam J, Ahmad S, Malhotra BD. 2008. Iron oxide nanoparticles-chitosan composite based glucose biosensor. Biosens Bioelectron. 24:676–683.
- Kaushik A, Solanki PR, Ansari AA, Sumana G, Ahmad S, Malhotra BD. 2009. Iron oxide-chitosan nanobiocomposite for urea sensor. Sensor Actuat B-Chem. 138:572–580.
- Khan R, Kaushik A, Solanki PR, Ansari AA, Pandey MK, Malhotra BD. 2008. Zinc oxide nanoparticle-chitosan composite film for cholesterol biosensor. Anal Chim Acta. 616:207–213.
- Koyuncu D, Erden PE, Pekyard mci Ş, Kiliç E. 2007. A new amperometric carbon paste enzyme electrode for ethanol determination. Anal Lett. 40:1904–1922.
- Li J, Wei X, Yuan Y. 2009. Synthesis of magnetic nanoparticles composed by Prussian blue and glucose oxidase for preparing highly sensitive and selective glucose biosensor. Sensor Actuat B-Chem. 139:400–406.
- Lin CC, Chen LC, Huang CH, Ding SJ, Chang CC, Chang HC. 2008. Development of the multi-functionalized gold nanoparticles with electrochemical-based immunoassay for protein A detection. J Electroanal Chem. 619–620:39–45.
- Lin J, He C, Zhao Y, Zhang S. 2009. One-step synthesis of silver nanoparticles/carbon nanotubes/chitosan film and its application in glucose biosensor. Sensor Actuat B-Chem. 137:768–773.
- Liu J, Lu F, Wang J. 1999. Metal-alloy-dispersed carbon-paste enzyme electrodes for amperometric biosensing of glucose. Electrochem Commun. 1:341–344.
- Liu Y, Yuan R, Chai Y, Tang D, Dai J, Zhong X. 2006. Direct electrochemistry of horseradish peroxidase immobilized on gold colloid/cysteine/nafion-modified platinum disk electrode. Sensor Actuat B-Chem. 115:109–115.
- Lu BW, Chen WC. 2006. A disposable glucose biosensor based on drop-coating of screen-printed carbon electrodes with magnetic nanoparticles. J Magn Magn Mater. 304:e400–e402.
- Luo L, Li Q, Xu Y, Ding Y, Wang X, Deng D, Xu Y. 2010. Amperometric glucose biosensor based on NiFe2O4 nanoparticles and chitosan. Sensor Actuat B-Chem. 145:293–298.
- Lv Y, Zhang Z, Chen F. 2003. Chemiluminescence microfluidic system sensor on a chip for determination of glucose in human serum with immobilized reagents. Talanta. 59:571–576.
- Martinez‐Peréz D, Ferrer ML, Mateo CR. 2003. A reagestless flourescent sol-gel biosensor for uric acid detection in biological fluids. Anal Biochem. 322:238–242.
- Saito T, Watanabe M. 1998. Characterization of poly(vinylferrocene-co-2-hydroxylethyl methacryate) for use as electron mediator in enzymatic glucose sensor. React Funct Polym. 37:263–269.
- Skoog DA, West DM, Holler FJ, Crouch SR. 2004. Fundamentals of analytical chemistry. Brooks/Cole, Thomson Learning Inc.: Belmont.
- Song MJ, Kim JH, Lee SK, Lee JH, Lim DS, Hwang SW, Whang D. 2010. Pt-polyaniline nanocomposite on boron-doped diamond electrode for amperometic biosensor with low detection limit. Microchim Acta. 171:249–255.
- Švancara I, Vytas K, Barek J, Zima J. 2001. Carbon paste electrodes in modern electroanalysis. Crit Rev Anal Chem. 31:311–345.
- Tang H, Chen J, Yao S, Nie L, Deng G, Kuang Y. 2004. Amperometric glucose biosensor based on adsorption of glucose oxidase at platinum nanoparticle-modified carbon nanotube electrode. Anal Biochem. 331:89–97.
- Wang H, Wang X, Zhang X, Qin X, Zhao Z, Miao Z, Huang N, et al. 2009. A novel glucose biosensor based on the immobilization of glucose oxidase onto gold nanoparticles-modified Pb nanowires. Biosens Bioelectron. 25:142–146.
- Wang J. 2006. Analytical electrochemistry. John Wiley & Sons Inc.: New Jersey.
- Wang J, Mo J W, Li S, Porter J. 2001. Comparison of oxygen-rich and mediator-based glucose-oxidase carbon-paste electrodes. Anal Chim Acta. 441:183–189.
- Wang J, Walcarius A. 1996. Zeolite containing oxidase-based carbon paste biosensors. J Electroanal Chem. 404:237–242.
- Wang J, Wang L, Di J, Tu Y. 2008. Disposable biosensor based on immobilization of glucose oxidase at gold nanoparticles electrodeposited on indium tin oxide electrode. Sensor Actuat B-Chem. 135:283–288.
- Xue MH, Xu Q, Zhou M, Zhu JJ. 2006. In situ immobilization of glucose oxidase in chitosan–gold nanoparticle hybrid film on Prussian Blue modified electrode for high-sensitivity glucose detection. Electrochem Commun. 8:1468–1474.
- Yang L, Ren X, Tang F, Zhang L. 2009. A practical glucose biosensor based on Fe3O4 nanoparticles and chitosan/nafion composite film. Biosens Bioelectron. 25:889–895.
- Yang Y, Yang M, Wang H, Tang L, Shen G, Yu R. 2004. Inhibition biosensor for determination of nicotine. Anal Chim Acta. 509:151–157.
- Yao Y, Shiu KK. 2007. Electron transfer properties of different carbon nanotube materials and their applications in glucose biosensors. Anal Bioanal Chem. 387:303–309.
- Yu J, Yu D, Zhao T, Zeng B. 2008. Development of amperometric glucose biosensor through immobilizing enzyme in a Pt nanoparticles/ mesoporous carbon matrix. Talanta. 74:1586–1591.
- Zaitoun MA. 2006. A kinetic-spectrophotometric method for the determination of glucose in solutions. J Anal Chem. 61:1010–1014.
- Zhu H, Lu X, Li M, Shao Y, Zhu Z. 2009. Nonenzymatic glucose voltammetric sensor based on gold nanoparticles/carbon nanotubes/ionic liquid nanocomposite. Talanta. 79:1446–1453.