ABSTRACT
To study the effect of myoendothelial communication on vascular reactivity, we integrated detailed mathematical models of Ca2+ dynamics and membrane electrophysiology in arteriolar smooth muscle (SMC) and endothelial (EC) cells. Cells are coupled through the exchange of Ca2+, Cl−, K+, and Na+ ions, inositol 1,4,5-triphosphate (IP3), and the paracrine diffusion of nitric oxide (NO). EC stimulation reduces intracellular Ca2+ ([Ca2+]i) in the SMC by transmitting a hyperpolarizing current carried primarily by K+. The NO-independent endothelium-derived hyperpolarization was abolished in a synergistic-like manner by inhibition of EC SKCa and IKCa channels. During NE stimulation, IP3 diffusing from the SMC induces EC Ca2+ release, which, in turn, moderates SMC depolarization and [Ca2+]i elevation. On the contrary, SMC [Ca2+]i was not affected by EC-derived IP3. Myoendothelial Ca2+ fluxes had no effect in either cell. The EC exerts a stabilizing effect on calcium-induced calcium release–dependent SMC Ca2+ oscillations by increasing the norepinephrine concentration window for oscillations. We conclude that a model based on independent data for subcellular components can capture major features of the integrated vessel behavior. This study provides a tissue-specific approach for analyzing complex signaling mechanisms in the vasculature.
ACKNOWLEDGEMENTS
This work was supported, in part, by the American Heart Association (grant NSDG 0435067N), the National Institutes of Health (grant SC1HL095101; Bethesda, Maryland, USA), and the Patras University Program (Karathoedori project no. C.177).
Declaration of interest: The authors report no financial conflicts of interest. The authors alone are responsible for the content and writing of this article.
This paper was first published online on iFirst on 4 September 2009.
SUPPLEMENTARY MATERIAL
S1. Dynamics of cell coupling
shows the predicted time course of EC and SMC Vm after instantaneous gap-junction uncoupling (t=10 minutes) and coupling (t=70 minutes). At steady state, the net influx of K+, Na+, Cl−, and Ca2+ through plasma membrane in isolated EC and SMC is zero. The intracellular ionic concentrations are maintained by active membrane pumps, and they return to their stable steady-state values after small perturbations. After gap-junction coupling (t=70 minutes), myoendothelial current is generated instantaneously, according to electrochemical gradients, for each ion across the junction. Since the ionic concentrations in EC and SMC are similar (but not the same), the potential difference will dominate and drive the flow of net positive charge from the EC to SMC. As a result, the SMC will depolarize and EC hyperpolarize until the myoendothelial current is compensated by new transmembrane currents in each cell. This process is fast because of the small RC time constant of the system (τ˜10−11 F * 109 Ω = 10−2 seconds) and small time constants of voltage-dependent activation/deactivation of channels. Ionic concentration changes are negligible in this initial phase. The gap junctional current will be mediated mostly by K+, because [K+]i is significantly larger than the cytosolic concentrations of the other ions. The amount of extra ions (ΔN) necessary to charge the membrane capacitance to the new Vm is very small, compared to the total amount of ions (N) in the cytoplasm, and does not affect significantly the ionic concentrations: ΔN=ΔQ/F=ΔVm*Cm/F; (i.e., for a 20-mV change in Vm, movement of 4.1 * 10−18 mol of K+ ion is required). In comparison, the total amount of K+ in each cell is ˜1.2 * 10−13 mol. However, this process disturbs the ionic mass balance, and the net flux of individual ionic species into each cell will not be zero anymore. Therefore, following this initial change in Vm, the ionic concentrations will drift, causing further changes in Vm through the modification of Nernst potentials, until a true steady state is reached. The magnitude and direction of the concentration and Vm changes are difficult to predict, but they are significant if the isolated cells exhibit differences before coupling. The time course of the drift is relatively slow () and depends on cell volumes and ionic buffering. Although the concentrations of individual ionic species drift significantly, they compensate each other so that the total amount of ions (and electric charge) is constant. It follows from the simulations, that unless the isolated cells have identical Vm and intracellular ionic concentrations, there will be, upon coupling, electrochemical gradients and ionic fluxes across gap junctions sustained by transmembrane currents.
Figure S1. Predicted time course of EC Vm (dashed-dotted line) and SMC Vm (solid line) after instantaneous gap-junction uncoupling (t=10 minutes) and coupling (t=70 minutes).
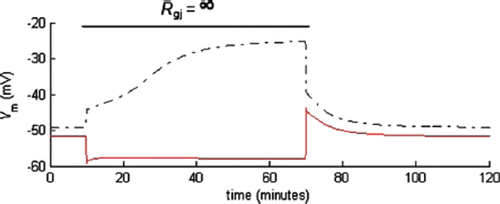
During gap-junction uncoupling, the reverse process takes place. Following an instantaneous termination of the gap-junction current (t=10 minutes), transmembrane currents depolarize the EC and hyperpolarize the SMC.
The myoendothelial ionic exchange during agonist stimulation resembles ionic exchange after myoendothelial coupling, but the time course of Vm change also depends on the kinetics of the signaling pathway that causes the opening of ion channels. For example, the activation of KCa channels after ACh stimulation hyperpolarizes EC and SMC (through the gap junctions) toward the Nernst potential for EC K+ until new electric-current balance in EC and SMC is reached. This response is fast and is associated with negligible changes in ionic concentrations. However, because of the new transmembrane and gap-junction currents, the balance of K+, Na+, Ca2+, and Cl− in EC and SMC is disturbed. If the agonist stimulation continues, ionic concentrations will drift slowly, affecting Vm through the modification of Nernst potentials. When the true steady state is reached, myoendothelial ionic fluxes are compensated by new fluxes through membrane channels and pumps. For example, prolonged ACh stimulation modified endothelial Nernst potentials for K+ from −80 to −76.4 mV, for Na+ from 51.2 to 41 mV, for Cl− from −26 to 32.4 mV, and for Ca2+ from 122 to 113 mV. The magnitude and direction of these drifts depend on membrane channels and pumps.
In the model, SMC and EC coupling depolarizes SMC, but in the literature, the endothelium has typically a hyperpolarizing effect on the smooth muscle layer [Citation1]. However, the removal of endothelium had no effect on SM Vm in L-NNA hypertensive rats [Citation1], and endothelial removal hyperpolarized SM in RMA incubated with L-NAME Citation9]. These observations suggest that the activity of the NO pathway, under basal conditions, may be more significant than predicted in the model. Unfortunately, NO release by the endothelium has not been well characterized, under any conditions, and published production rates differ by three orders of magnitude.
Table S1. Resting membrane potentials and ionic concentrations in isolated and integrated SMC and EC.
S2. K+-induced oscillations
A shows model simulations for the effect of extracellular K+ on SMC Ca2+. Increasing [K+]o depolarized the SMC, opened VOCCs, and raised SMC [Ca2+]i. When the Ca2+ elevation exceeded the threshold value of ˜160 nM, sustained Ca2+ oscillations in the SMC were generated through the CICR mechanism. We have predicted similar oscillations in the isolated SMC model [Citation5]. The period (21–30 seconds) and amplitude of the oscillations were not affected significantly by the integration of the SMC with the EC. Relative to the isolated SMC, the minimum [K+]o initiating the oscillations was reduced from 25 to 20 mM as a result of the basal SMC depolarization and Ca2+ elevation in the integrated model. Model results are shown next to corresponding experimental data reproduced from [Citation7]. Changes in diameter and SM [Ca2+]i were recorded in isolated RMA by using fluorescence and video microscopy. As [K+]o increases above 20 mM, oscillations appear in [Ca2+]i and the vessel's diameter with a period of 25 seconds. The amplitude of the oscillations was not explicitly quantified by calibrating the fluorescence signal, but it increases with increasing [K+]o and appears smaller than the theoretical predictions.
Figure S2. KCl-induced Ca2+ oscillations in SM. (A) In the model, increasing [K+]o depolarized the SMC, opened VOCCs, and raised SMC [Ca2+]i. When the mean Ca2+ exceeded the threshold value of ˜160 nM, sustained Ca2+ oscillations in the SMC were generated through the CICR mechanism. (B) Experimental data reproduced from 7. Changes in diameter and SM [Ca2+]i were recorded in an isolated second-order RMA (350±40 µm in external diameter), using fluorescence and video microscopy. As [K+]o increases above 20 mM, oscillations appear in [Ca2+]i and the vessel's diameter.
![Figure S2. KCl-induced Ca2+ oscillations in SM. (A) In the model, increasing [K+]o depolarized the SMC, opened VOCCs, and raised SMC [Ca2+]i. When the mean Ca2+ exceeded the threshold value of ˜160 nM, sustained Ca2+ oscillations in the SMC were generated through the CICR mechanism. (B) Experimental data reproduced from 7. Changes in diameter and SM [Ca2+]i were recorded in an isolated second-order RMA (350±40 µm in external diameter), using fluorescence and video microscopy. As [K+]o increases above 20 mM, oscillations appear in [Ca2+]i and the vessel's diameter.](/cms/asset/6b1d7b06-9e14-4a80-a20e-b362ddc59694/imic_a_417926_f0020_b.gif)
S3. Relative contribution of SKCa and IKCa channels to EDHF
In guinea-pig coronary arteries [Citation2] and some studies on RMA [Citation4], apamine (a selective inhibitor of SKCa) had a limited effect on EDHF. However, in other RMA studies, apamine was more effective than selective blockers of IKCa channels, and the effect may depend on SM stimulation (e.g., in [Citation3,Citation2]. According to the model, the differences can result from different expression levels and Ca2+ sensitivities of SKCa and IKCa channels in different vessel types and from various EC Ca2+ levels during EC and SMC stimulation in the same vessel. depicts the effect of EC [Ca2+]i on the ratio of SKCa and IKCa currents with parameters mostly from porcine coronary and mouse aortic endothelial cells. At high (i.e., saturating) [Ca2+]i, the two channel types are maximally activated and the current ratio is determined only by the maximum whole-cell channel conductances (i.e., GSKCa:GIKCa=1:2.8). At low [Ca2+]i, the ratio depends on several parameters determining the Ca2+-dependent activation of SKCa and IKCa channels (, caption). In particular, at [Ca2+]i=200 nM, SKCa current is significantly larger than IKCa current, in spite of the smaller whole-cell conductance.
Figure S3. The ratio of SKCa and IKCa currents as a function of EC [Ca2+]i calculated from the following formula:
GIKCa=1.72 nS, KSKCa,Cai=237 nM, KIKCa,Cai=740 nM, nSKCa=5, and nIKCa=4 [Citation8]. At saturating [Ca2+]i>KIKCa,Cai, the two channel types are maximally activated and the current ratio is determined only by the maximum whole-cell channel conductances (i.e., GSKCa:GIKCa=1:2.8). At low [Ca2+]i<KIKCa,Cai, the ratio depends on EC [Ca2+]i, the maximum whole-cell conductances, and the parameters describing Ca2+-dependent activation of the channels.


![Figure S3. The ratio of SKCa and IKCa currents as a function of EC [Ca2+]i calculated from the following formula: Display full size Display full sizeGIKCa=1.72 nS, KSKCa,Cai=237 nM, KIKCa,Cai=740 nM, nSKCa=5, and nIKCa=4 [Citation8]. At saturating [Ca2+]i>KIKCa,Cai, the two channel types are maximally activated and the current ratio is determined only by the maximum whole-cell channel conductances (i.e., GSKCa:GIKCa=1:2.8). At low [Ca2+]i<KIKCa,Cai, the ratio depends on EC [Ca2+]i, the maximum whole-cell conductances, and the parameters describing Ca2+-dependent activation of the channels.](/cms/asset/3c0b5eee-4028-4f11-96c3-cad07553fbf7/imic_a_417926_ilg0017.gif)
S4. Multiple layers of SMCs
To investigate the effect of multiple SMC layers in the vascular wall, we performed a simulation with three SMCs connected in series and the first SMC coupled to the EC. The neighboring SMCs were coupled according to Equations 1, 2, and 6. We assumed a SMC-to-SMC gap-junction resistance (Rgj,SM) of 84.7 MΩ [Citation6] and an inversely proportional IP3 permeability between SMCs (i.e., pIP3,SM=900/84.7*0.05 s−1). NO concentration was assumed to be the same in all SMCs. [A relative flat concentration gradient in the vascular wall is predicted in the absence of significant extravascular NO scavenging (i.e., by myoglobin or nearby capillaries) [Citation10]].
,, show the predicted changes in Vm and [Ca2+]i induced by the agonist stimulations of the EC and three SMCs. When the cells are coupled by ionic, IP3, and NO fluxes, small differences are observed between the single and the multilayer model (compare vs. ). After the blockade of the NO, the EC-induced hyperpolarization of SM (preconstricted with NE) was significantly compromised in the multilayer, as compared to the single-layer, model ( vs. ). The reduction of myoendothelial resistance from 900 to 35 MΩ reversed this difference and restored EDHF-dependent relaxation in the multilayered model (). Thus, the model predicts a different requirement for gap-junction density in single vs. multilayered arterioles for an effective role of ionic coupling in EDHF.
Figure S4. NE (1 µM)- and ACh (1 a.u.)-induced changes of Vm and [Ca2+]i in the model with three SMCs (solid lines) and one EC (dashed-dotted lines) in the presence of the ionic (EC-SMC Rgj=900 MΩ), IP3, and NO couplings. Predicted responses are similar to responses in the model with single SMC (Figure 5).
![Figure S4. NE (1 µM)- and ACh (1 a.u.)-induced changes of Vm and [Ca2+]i in the model with three SMCs (solid lines) and one EC (dashed-dotted lines) in the presence of the ionic (EC-SMC Rgj=900 MΩ), IP3, and NO couplings. Predicted responses are similar to responses in the model with single SMC (Figure 5).](/cms/asset/d6cf47e9-b367-4d77-af4f-e6b4d85979e8/imic_a_417926_f0021_b.gif)
Figure S5. Predicted NE (1 µM)-induced and ACh (1 a.u.)-induced changes of of Vm and [Ca2+]i in SMCs (solid lines) and EC (dashed-dotted lines) coupled by the ionic (EC-SMC Rgj=900 MΩ) and IP3 fluxes (NO blocked). The EC-induced hyperpolarization of SMCs preconstricted with NE was significantly compromised, as compared to the single-layer model (Figure 4).
![Figure S5. Predicted NE (1 µM)-induced and ACh (1 a.u.)-induced changes of of Vm and [Ca2+]i in SMCs (solid lines) and EC (dashed-dotted lines) coupled by the ionic (EC-SMC Rgj=900 MΩ) and IP3 fluxes (NO blocked). The EC-induced hyperpolarization of SMCs preconstricted with NE was significantly compromised, as compared to the single-layer model (Figure 4).](/cms/asset/91920916-6ffd-4f11-8602-3ba900754713/imic_a_417926_f0022_b.gif)
Figure S6. Predicted NE (1 µM)-induced and Ach (1 a.u.)-induced changes of of Vm and [Ca2+]i in SMCs (solid lines) and EC (dashed-dotted lines) coupled by the ionic and IP3 fluxes (NO blocked) after myoendothelial resistance reduction from 900 to 35 MΩ. The reduction of EC-SMC Rgj compensated the increased electrical load from SMCs and restored the EDHF.
![Figure S6. Predicted NE (1 µM)-induced and Ach (1 a.u.)-induced changes of of Vm and [Ca2+]i in SMCs (solid lines) and EC (dashed-dotted lines) coupled by the ionic and IP3 fluxes (NO blocked) after myoendothelial resistance reduction from 900 to 35 MΩ. The reduction of EC-SMC Rgj compensated the increased electrical load from SMCs and restored the EDHF.](/cms/asset/e3f00998-d148-42c4-9811-0905faeffc5c/imic_a_417926_f0023_b.gif)
Initial conditions for the coupled system
SMC: Vm= − 52.7 mV; [Ca]i=95.9 nM; [Ca]u=0.772 mM; [Ca]r=0.729 mM; [Na]i=9.39 mM; [K]i=121 mM; [Cl]i=42.0 mM; RyR R01=0.0012; R10=0.003; R11=3.62 · 10−6; [IP3]=[DAG]=[cGMP] = 0; VcGMP=0. Adrenoceptor phosphorylation rate kp,G=0. EC: Vm= − 49.8 mV; [Ca2+]i=131 nM; [Ca2+]IS=3.3 mM; [Na]i=18.7 mM; [K]i=116 mM; [Cl]i=46.3 mM; [IP3] = 0; QGIP3=0.