Abstract
Successful heart preservation is critical for clinical heart transplantation, but even the most successful heart preservation method can only preserve the heart in a time range of approximately 4 h. In the present study, diazoxide (DE)-containing cardioplegia solution was used as the hypothermic heart preservation solution, and hearts were preserved for different time periods before reperfusion. Five groups were randomly divided into the control group, DE groups with different concentrations of DE (15, 30, and 45 μM), and the 5-HD + DE group containing 30 μM DE and 100 μM of 5-hydroxydecanoate (5-HD). Each group was further divided according to the storage time: 3 h and 8 h subgroups. Hemodynamic parameters were monitored continually and myocardial injury markers lactate dehydrogenase (LDH) and creatine kinase (CK) were measured before arrest and during reperfusion. After reperfusion, myocardium water content, myocardium apoptosis, and myocardial mitochondrial superoxide dismutase (SOD) activity and malondialdehyde (MDA) content were measured. All data were analyzed by independent samples t-test. Our results showed that diazoxide treatment improved impaired hemodynamics parameters and decreased the leakage of myocardial enzymes during reperfusion, compared to the control group. Moreover, diazoxide treatment significantly alleviated myocardial edema, decreased the number of TUNEL-positive cardiomyocytes, prevented the loss of mitochondrial SOD activity, and depressed the mitochondrial MDA content. This study indicated that mitoKATP channel opener diazoxide could significantly enhance myocardial protection during long-term hypothermic preservation, and the inhibition of oxidative stress-induced myocardium apoptosis seems to be the mechanism involved in the protective action on the mitoKATP channel.
Introduction
As the ultimate solution for end-term heart diseases, heart transplantation has now been widely accepted. But the major obstacle confronting clinical surgeons is the lack of a dependable organ preservation method which can preserve the donor heart as long as possible while the heart is still recovering to a clinically satisfactory extent after transplantation (CitationSadala & Stolf, 2008). Unfortunately, the hypothermia preservation method in practice can only preserve the donor time in a range of about 4 h (CitationWittwer & Wahlers, 2008), and as the preservation time increases from 2 to 4 h, the death rate also increases from 9.8 to 17.6%. Recent research indicates that myocardium ischemia–reperfusion injury is the major reason responsible for the high incidence of complications and the high mortality rate after heart transplantation, and, thus, reducing ischemia–reperfusion injury has become the primary concern for research on heart preservation.
CitationNoma (1983) initially reported the existence of the adenosine triphosphate (ATP)-sensitive potassium channel, and the term “ATP-sensitive potassium channel” (KATP) indicates that the activity of this channel is dependent on the intracellular ATP level. CitationInoue et al. (1991) discovered another type of KATP in the inner mitochondrial membrane of the rat hepatocyte, and other scientists (CitationGhosh et al., 2000) verified the existence of such a potassium-selective channel in the inner mitochondrial membrane of the rat cardiomyocyte by using electron microscopy and fluorescence staining techniques. Because the potassium ion flow through this channel is inhibited by ATP, this newly found channel was named the mitochondrial ATP-sensitive potassium channel #(mitoKATP). Subsequently, this channel has also been found in skeletal muscle, smooth muscle, β-cells of the pancreas, brain, and kidney, etc. The mitoKATP is attracting increasing interest after the discovery of its role in mediating the so called ischemic preconditioning effect, a phenomenon which can be elicited by multiple factors such as ischemia, hypoxia, calcium, adenosine, opium peptide, acetylcholine, and inducible nitric oxide synthase, believed to mediate the cardioprotective effect after reperfusion of the heart (CitationGross, 2004; CitationKuzelova et al., 2006; CitationXie et al., 2006; CitationZhang et al., 2005). Activation of this channel seems to be able to initiate an endogenous signal pathway which can protect the heart against ischemic injury. All these factors inspired us to determine whether we could gain additional protective effect if we added the selective mitoKATP opener diazoxide (DE) into the standard hypothermia preservation solution, and to explore the mechanism behind this.
Materials and methods
Materials
Diazoxide (DE), 5-hydroxydecanoate (5-HD), and dimethylsulfoxide (DMSO) were from Sigma Co. (USA). The terminal deoxynucleotidyl transferase-mediated dUTP nick-end labeling (TUNEL) kit was from Roche Molecular Biochemicals Co. (Germany). The diaminobenidine (DAB) kit was from Boster Co. (Wuhan, China). Superoxide dismutase (SOD), malondialdehyde (MDA), and Coomassie Brilliant Blue kits were from Jiancheng Bioengineering Institute (Nanjing, China). Male Sprague-Dawley (SD) rats (220–280 g) were purchased from the Experimental Animal Center of Zhejiang University.
Animal grouping
Five groups were randomly divided: (1) control group: the isolated heart was stored in Celsior solution after balancing; (2) DE group: the isolated heart was stored in Celsior solution containing different concentrations of DE (15, 30, and 45 μM); (3) 5-HD + DE group: the isolated heart was stored in Celsior solution containing 30 μM DE and 100 μM 5-hydroxydecanoate (5-HD). Each group was further divided according to the storage time: 3 h and 8 h subgroups. All procedures were conducted with the approval of the local animal care committee.
In vitro hypothermia heart preservation model
Male SD rats were executed by cervical joint dislocation and the hearts were quickly removed from the chest, washed in 4°C Krebs–Henseleit (K–H) solution to get rid of blood, fixed to the Langendorff perfusion apparatus, and perfused reversely with K–H solution (mM: NaCl 118.0, KCl 4.7, KH2PO4 1.2, MgSO4 1.2, NaHCO3 25.0, CaCl2 1.25, glucose 10.0; pH 7.4) at constant pressure (76 mmHg). During the whole perfusion period, the perfusion temperature was maintained at 37°C and the K–H solution was saturated with 95% O2–5% CO2 air. After balancing for 30 min, the hemodynamics parameters of the ventricle were recorded as the basal values. After that, 4°C Celsior solution (mM: NaOH 100, KCl 15, MgCl2 13, CaCl2 0.25, mannitol 60, lactobionate 80, histidine 30, glutamate 20; pH 7.4) was perfused into the aorta to induce cardiac arrest and the perfusion pressure was maintained at 76 mmHg. The perfusion time was less than 3 min and the heart surface was cooled down simultaneously. After preservation for certain time periods, the hearts were reloaded onto the Langendorff apparatus and reperfused for another 60 min. Hemodynamics parameters including left ventricular developed pressure (LVDP) and coronary flow (CF) were recorded 10 min after the beginning of reperfusion.
LDH and CK content in the coronary outflow
The coronary outflow was collected during the balancing and reperfusion process and its lactate dehydrogenase (LDH) and creatine kinase (CK) content was measured by an autonomous biochemical analyzer (CX-4; Beckman Co., USA).
Myocardium edema
After reperfusion was finished, a piece of myocardium tissue was cut from the anterior wall of the left ventricle and weighed (wet weight). The same specimen was baked in an 80°C oven and weighed again (dry weight). The myocardium water content = (wet weight – dry weight)/wet weight × 100%.
TUNEL assessment of cell apoptosis
After reperfusion, the cardiac apex of the left ventricle was cut off, fixed in 10% formaldehyde, routinely dehydrated, paraffin embedded, and cut into sections. The TUNEL method was applied to assess cardiomyocyte apoptosis. Slides were dewaxed using dimethylbenzene, grade-ethanol hydrated, protein K treated for 30 min at 37°C, washed with phosphate buffered saline (PBS), 0.3% H2O2 methanol solution-treated for 30 min at room temperature, washed with PBS, incubated with a fluorescein isothiocyanate (FITC)-ligated nucleotide and terminal deoxynucleotidyl transferase (TdT) mixture for 60 min at 37°C, washed with PBS, incubated with horseradish peroxidase (HRP) solution for 30min at 37°C, washed with PBS, DAB color developed, hematoxylin redyed, dehydrated, and observed under an optical microscope (×400 magnification). Ten visual fields were randomly selected on each slide (total of about 500 cells), and cells with brown staining of the nucleus were regarded as apoptotic. The apoptosis index (AI) = apoptotic cell number/total cell number × 100%.
Mitochondrial SOD and MDA content in cardiomyocytes
The ventricle parts of the hearts were weighed, moved to cold saline, chopped into pieces, and homogenized into 10% tissue homogenate (homogenized for 3 × 10 s with 30 s intervals on ice). Some 5–10 mL of 10% tissue homogenate was centrifuged at 1000 rpm for 10 min (4°C) and the supernatant was centrifuged at 10,000 rpm for 20 min (4°C). The mitochondria pellet was resuspended in cold saline at the concentration of 0.1 g/mL. According to the kit instructions, myocardial mitochondrial SOD content (U/mg protein) was determined by the xanthine oxidase method and the mitochondrial MDA content (nmol/mg protein) was determined by the thiomalonylurea method. The Coomassie Brilliant Blue method was used to measure the protein concentration.
Statistical analysis
Data are all expressed as mean ± SD and were analyzed by Student’s t-test (two-group data) and one way analysis of variance (ANOVA) followed by the Student–Newman–Keuls (data from more than three groups) method.
Results
Cardiac function recovery after hypothermic ischemic preservation
LVDP of the left ventricle
There was no significant difference in LVDP among the groups before the experiment (p > 0.05). The LVDP value at balancing was taken as 100%, and the LVDP recovery rate was observed after the hypothermic ischemic preservation. When compared with the control, DE addition significantly enhanced the LVDP recovery rate in both the 3 h and 8 h groups (p < 0.05). There was no significant difference between the 5-HD + DE group and the control group in LVDP recovery rate (p > 0.05).
Three different DE concentrations (15, 30, and 45 μM) could all improve the LVDP recovery rate in the 8 h long-term hypothermic ischemic preservation (p < 0.05); the effect of 30 and 45 μM DE was better than that of 15 μM, while no difference existed between the 30 and 45 μM DE groups (p > 0.05) ().
Figure 1. Time changes of left ventricular developed pressure (LVDP) (% of equilibrium values) during 60 min of reperfusion after hypothermic preservation. (A) 3 h of preservation; (B) 8 h of preservation. Data are expressed as mean ± SD, n = 8. *p < 0.05, **p < 0.01 vs. control group; #p < 0.05, ##p < 0.01 vs. 30 μM DE group; +p < 0.05, ++p < 0.01 vs. 15 μM DE group.
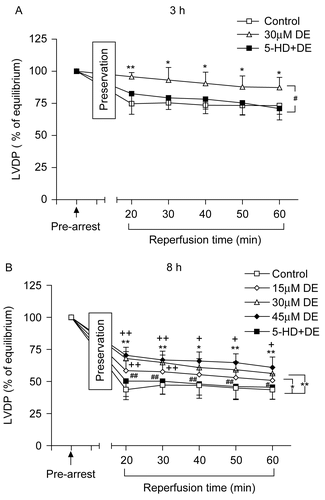
Coronary flow recovery after hypothermic ischemic preservation
Coronary flow values showed no significant difference among the groups before the experiment (p > 0.05). The CF value at balancing was taken as 100% and the CF recovery rate of every group after hypothermic ischemic preservation was observed. There was no significant difference among the groups in CF recovery rate when the hearts were stored in vitro for 3 h (p > 0.05). When compared with the control, 30 μM DE could improve the CF recovery rate in the 8 h subgroup (p < 0.05), while the 5-HD + DE group showed no significant difference compared with the control group (p > 0.05).
In the long-term hypothermic ischemic preservation group (8 h), 15 μM DE had no significant difference compared with the control group in CF recovery rate (p > 0.05), while 30 and 45 μM DE could significantly improve the CF recovery rate (p < 0.05). There was no significant difference between the 30 and 45 μM DE groups (p > 0.05) ().
Figure 2. Recovery of coronary flow (CF) (% of equilibrium values) at the end of reperfusion after hypothermic preservation. Data are expressed as mean ± SD, n = 8. *p < 0.05, **p < 0.01 vs. control group; #p < 0.05, +p < 0.05 vs. 15 μM DE group.
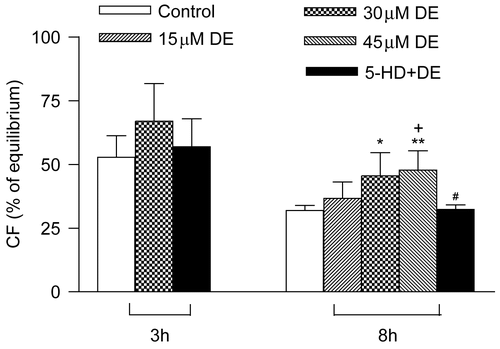
Because 30 μM DE could maximally preserve the cardiac contractile function and coronary flow in the long-term hypothermic ischemic preservation, as a result this concentration was adopted in the following experiments.
Myocardium enzyme changes
The coronary outflow collected during balancing showed no significant difference in LDH and CK content among the different groups (p > 0.05). After preservation for certain times, the LDH content in all groups showed a peak level at 2–4 min after reperfusion and then declined (). When compared with the control, the 30 μM DE supplement could significantly decrease the LDH and CK levels in the 8 h subgroup (p > 0.05). DE also could lower the LDH and CK leakage at certain time points (2 min and 4 min) in the 3 h subgroup (p > 0.05). 5-HD could partially eliminate this effect ( and ).
Figure 3. Release of myocardial lactate dehydrogenase (LDH) in coronary effluent before arrest and during reperfusion after hypothermic preservation. (A) 3 h of preservation; (B) 8 h of preservation. Data are expressed as mean ± SD, n = 8. *p < 0.05, **p < 0.01 vs. control group; ##p < 0.01 vs. 30 μM DE group.
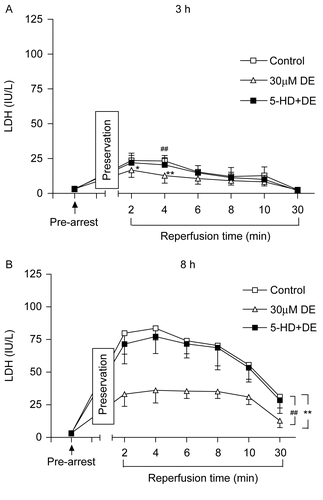
Cardiomyocyte apoptosis
After preservation for 8 h, cardiomyocyte apoptosis could be observed in all groups. When compared with the control, the 30 μM DE addition could significantly decrease the AI in the 8 h subgroup (p < 0.05). However, the 5-HD + DE group showed no significant difference compared with the control (p > 0.05) ( and ).
Figure 5. Evaluation of cardiomyocyte apoptosis using terminal dUTP nick-end labeling (TUNEL) after 8 h of hypothermic preservation. Data are expressed as mean ± SD, n = 7–8. *p < 0.05 vs. control group; #p < 0.05 vs. 30 μM DE group.
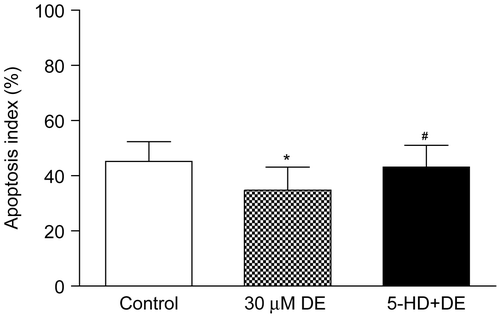
Figure 6. Cardiomyocyte apoptosis detected by TUNEL after 8 h hypothermic preservation (×200). Brown staining (TUNEL positive) indicates apoptotic cardiomyocytes (arrow). (A) Normal group: myocardium simply perfused with K–H solution for 30 min; (B) control group: myocardium preserved in Celsior solution for 8 h; (C) DE group: myocardium preserved in Celsior solution supplemented with 30 μM DE for 8 h; (D) 5-HD + DE group: myocardium preserved in Celsior solution supplemented with 30 μM DE and 100 μM 5-HD for 8 h.
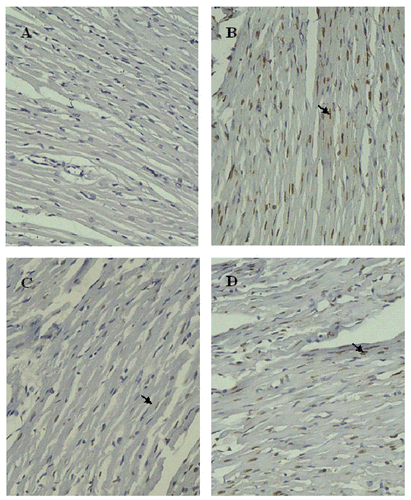
Mitochondrial SOD and MDA levels after reperfusion
When compared with the control, the 30 μM DE addition could significantly preserve the cardiomyocyte mitochondrial SOD activity after 8 h in vitro hypothermic ischemic preservation (p < 0.05), but in the 30 min reperfusion groups, no significant difference existed among control, DE, and 5-HD + DE in SOD activity (p < 0.05) ().
Figure 7. Activities of mitochondrial superoxide dismutase (SOD) in preserved hearts and reperfused hearts. Data are expressed as mean ± SD, n = 8. **p < 0.01 vs. control group; #p < 0.05 vs. 30 μM DE group.Compared with the control, the 30 μM DE addition could significantly inhibit the rise in cardiomyocyte mitochondrial MDA level after 8 h in vitro hypothermic ischemic preservation and 8 h ischemic preservation plus 30 min reperfusion (p < 0.05). The 5-HD + DE group showed no significant difference compared with the control (p > 0.05) ().
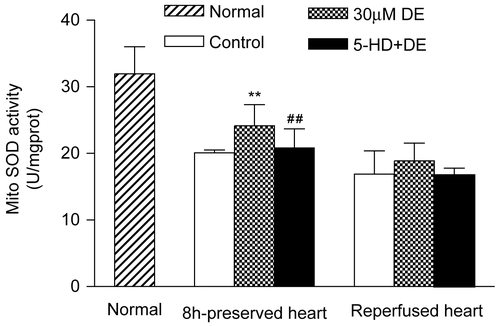
Discussion
Successful organ preservation is the premise for clinical organ transplantation. However, hypothermic preservation of the heart has a limit of about 4 h, which is much lower than that for the liver, kidney, and pancreas (CitationSadala & Stolf, 2008). Moreover, recent studies indicate that the myocardium ischemia–reperfusion injury is the major player mediating the high mortality and high complication rates after heart transplantation. The mitoKATP is an important channel that links the myocardial metabolism to cell membrane electrical activity. Recent advances indicate that mitoKATP has a cardioprotective role by mimicking the ischemia preconditioning phenomenon. Diazoxide, the specific opener of mitoKATP, could decrease the myocardial infarct size, promote the recovery of heart function, attenuate vascular endothelial injury, and thus protect the heart from ischemia–reperfusion injury (CitationGuo et al., 2004; CitationKorotkov et al., 2007). Inspired by the discoveries mentioned above, we turned our attention to applying DE in hypothermic preservation of the heart. In our study, we added DE directly into the standard Celsior solution and found that the DE supplement could significantly preserve the function of the left ventricle, promote recovery of the coronary flow, decrease enzyme leakage during reperfusion, and inhibit myocardial edema in long-term (8 h) hypothermic ischemic preservation. The above protective effects of DE were eliminated by 5-HD, the specific antagonist of mitoKATP, which further verified that the opening of the mitoKATP is the reason for these cardioprotective effects.
In our study, enzyme leakage reached a peak level at 2–4 min after reperfusion and declined thereafter in all groups, indicating that the early phase of reperfusion is the major period mediating the ischemia–reperfusion injury. DE addition could significantly lower the LDH and CK leakage, which was reversed by 5-HD. These results indicate that opening of the mitoKATP during in vitro ischemic preservation could stabilize the myocardial membrane and thus inhibit the reperfusion. We also discovered that DE treatment had no obvious effects on heart function, coronary recovery, and myocardial edema in the 3 h preservation subgroup, while improving all these parameters in the 8 h subgroup. This may be because the 3 h in vitro effect is still within the preservation capacity of the Celsior solution (CitationBoggi et al., 2005), which contains protective agents such as mannitol, reduced glutathione, and histidine buffering system. These ingredients could alleviate the oxidative injury, supply energy to cardiomyocytes under ischemic conditions, and improve the vascular endothelium (CitationMohara et al., 2001; CitationPietri et al., 1994), all of which could contribute to the preservation of heart function and coronary flow in the 3 h subgroup. Previous studies showed that 8–12 h is the phase where the myocardial energy store and function degenerate dramatically (CitationKu et al., 1997), which is in accordance with our results. Mitochondria structural change is the early marker for cell injury (CitationLatchoumycandane et al., 2006), and research has already verified that DE could protect the myocardial mitochondrial membrane function and integrity (CitationAkao et al., 2003). Based on this evidence, we hypothesize that DE might preserve the mitochondrial function or integrity by opening of the mitoKATP, and the integrity of the mitochondria is critical for the long-term hypothermic ischemic preservation of the heart.
Although it was reported that the EC50 of DE-induced mitoKATP opening is 27 μM, other researchers reported that the DE-mediated cardioprotective effect is dose dependent in the 1–30 μM range while showing the same effect at 30–100 μM (CitationLiu et al., 1998). Because a high dose of DE also has an effect on the vascular smooth muscle (CitationPirotte et al., 2000), there is still no agreement on the specific concentration at which DE could elicit the cardioprotective effect. In our own study, of the three concentrations used, we proved that 30 and 45 μM DE were more effective than 15 μM DE and there was no significant difference between 30 and 45 μM DE. This result may supply a certain basis for further clinical usage.
Although the idea that opening of the mitoKATP could alleviate the myocardial ischemia–reperfusion injury is recognized, the specific mechanism is still obscure. Possible mechanisms involve inhibition of calcium overload, regulation of mitochondrial matrix volume, promotion of oxidative metabolism and mitochondrial respiration, and inhibition of apoptosis induced by oxidative stress (CitationAkao et al., 2001). Research has indicated that oxidative stress is the major mechanism mediating myocardial ischemia–reperfusion injury (CitationDas & Maulik, 2004). Oxidative stress could generate a large amount of free radicals which attack the lipid membrane of both the cells and the mitochondria, leading to mitochondrial swelling and rupture, inner calcium release and calcium overload, caspase family enzyme activation, and cell apoptosis finally (CitationHan et al., 2004). Our results showed that DE addition could preserve the mitochondrial SOD activity, inhibit the rise in MDA level, and decrease myocardial apoptosis in the long-term preservation group, suggesting that activation of mitoKATP during heart hypothermic ischemic preservation could improve the antioxidative capacity of the cells and contribute to myocardium survival.
To summarize, the supplement of DE in standard Celsior solution could significantly improve the long-term (8 h) heart preservation effect, and opening of the mitoKATP during hypothermic ischemic preservation could stabilize the myocardial membrane as well as the mitochondrial membrane, promote the antioxidative capacity, and inhibit cell apoptosis, all of which are beneficial to the long-term preservation of the heart. Further efforts are needed to explore the specific mechanism involved.
Acknowledgements
This study was supported by National Natural Science Foundation grant No. 30470635 and Science and Technology Department of Zhejiang Province grants No. 2005C23033 and No. 2007C33026.
References
- Akao M, O’Rourke B, Kusuoka H, Teshima Y, Jones SP, Marban E (2003): Differential actions of cardioprotective agents on the mitochondrial death pathway. Circ Res 92: 195–202.
- Akao M, Ohler A, O’Rourke B, Marban E (2001): Mitochondrial ATP-sensitive potassium channels inhibit apoptosis induced by oxidative stress in cardiac cells. Circ Res 88: 1267–1275.
- Boggi U, Signori S, Vistoli F, Del Chiaro M, Pietrabissa A, Croce C, Barsotti M, Bartolo TV, Amorese G, Capocasale E, Della Valle R, Mazzoni MP, Mosca F (2005): University of Wisconsin solution versus Celsior solution in clinical pancreas transplantation. Transplant Proc 37: 1262–1264.
- Das DK, Maulik N (2004): Conversion of death signal into survival signal by redox signaling. Biochemistry (Mosc) 69: 10–17.
- Ghosh S, Standen NB, Galinanes M (2000): Evidence for mitochondrial K ATP channels as effectors of human myocardial preconditioning. Cardiovasc Res 45: 934–940.
- Gross M (2004): Flavonoids and cardiovascular disease. Pharm Biol 42: 21–35.
- Guo W, Shen YL, Chen YY, Hu ZB, Yan ZK, Xia Q (2004): [The protective effect of diazoxide on long-term heart preservation]. Sheng Li Xue Bao 56: 632–638.
- Han H, Long H, Wang H, Wang J, Zhang Y, Wang Z (2004): Progressive apoptotic cell death triggered by transient oxidative insult in H9c2 rat ventricular cells: A novel pattern of apoptosis and the mechanisms. Am J Physiol Heart Circ Physiol 286: H2169–H2182.
- Inoue I, Nagase H, Kishi K, Higuti T (1991): ATP-sensitive K+ channel in the mitochondrial inner membrane. Nature 352: 244–247.
- Korotkov SM, Nesterov VP, Demina IN, Ryabchikov NN (2007): Effect of diazoxide and Ca2+ on rat heart mitochondria loaded with Na+. Dokl Biochem Biophys 414: 141–145.
- Ku K, Oku H, Alam MS, Saitoh Y, Nosaka S, Nakayama K (1997): Prolonged hypothermic cardiac storage with histidine-tryptophan-ketoglutarate solution: Comparison with glucose-insulin-potassium and University of Wisconsin solutions. Transplantation 64: 971–975.
- Kuzelova M, Mladonicka M, Bukovsky M, Dubnickova M, Adameova A, Svec P (2006): NO-synthase inhibitors provide influence on protective effect of modified endotoxine diphosphoryl lipid A in a rat heart model of ischemic-reperfusion injury. Pharmazie 61: 568–570.
- Latchoumycandane C, Seah QM, Tan RC, Sattabongkot J, Beerheide W, Boelsterli UA (2006): Leflunomide or A77 1726 protect from acetaminophen-induced cell injury through inhibition of JNK-mediated mitochondrial permeability transition in immortalized human hepatocytes. Toxicol Appl Pharmacol 217: 125–133.
- Liu Y, Sato T, O’Rourke B, Marban E (1998): Mitochondrial ATP-dependent potassium channels: novel effectors of cardioprotection? Circulation 97: 2463–2469.
- Mohara J, Takahashi T, Oshima K, Aiba M, Yamagishi T, Takeyoshi I, Matsumoto K, Morishita Y (2001): The effect of Celsior solution on 12-hour cardiac preservation in comparison with University of Wisconsin solution. J Cardiovasc Surg (Torino) 42: 187–192.
- Noma A (1983): ATP-regulated K+ channels in cardiac muscle. Nature 305: 147–148.
- Pietri S, Culcasi M, Albat B, Alberici G, Menasche P (1994): Direct assessment of the antioxidant effects of a new heart preservation solution, Celsior. A hemodynamic and electron spin resonance study. Transplantation 58: 739–742.
- Pirotte B, Ouedraogo R, de Tullio P, Khelili S, Somers F, Boverie S, Dupont L, Fontaine J, Damas J, Lebrun P (2000): 3-Alkylamino-4H-pyrido[2,3-e]-1,2,4-thiadiazine 1,1-dioxides structurally related to diazoxide and pinacidil as potassium channel openers acting on vascular smooth muscle cells: design, synthesis, and pharmacological evaluation. J Med Chem 43: 1456–1466.
- Sadala ML, Stolf NA (2008): Heart transplantation experiences: a phenomenological approach. J Clin Nurs 17: 217–225.
- Wittwer T, Wahlers T (2008): Marginal donor grafts in heart transplantation: lessons learned from 25 years of experience. Transpl Int 21: 113–125.
- Xie J, Zeng Q, Wang L (2006): The protective effect of L-carnitine on ischemia-reperfusion heart. J Huazhong Univ Sci Technolog Med Sci 26: 188–191.
- Zhang XM, Shen F, Xv ZY, Yan ZY, Han S (2005): Expression changes of thrombospondin-1 and neuropeptide Y in myocardium of STZ-induced rats. Int J Cardiol 105: 192–197.