Abstract
Context: Alpinia katsumadai (Zingiberaceae) has been identified by the National Plant Quarantine Service in Korea. The extract of Alpinia katsumadai seed (EAKS) has antioxidant activities.
Objective: We investigated the neuroprotective effects of EAKS on ischemic damage in the gerbil hippocampal CA1 region after transient cerebral ischemia.
Materials and methods: The ethanol extract of EAKS was obtained by organic solvent, collected in Kangwon province (South Korea) and orally administered using a feeding needle once a day for one week before transient cerebral ischemia in gerbils.
Result: We adapted oral administration of 25 and 50 mg/kg EAKS because there are no data about the absorption and metabolism of EKAS. We found a significant neuroprotection in the 50 mg/kg EAKS-treated ischemia group, not in the 25 mg/kg EAKS-treated ischemia group, at 4 days ischemia-reperfusion (I-R). In the 50 mg/kg EAKS-treated ischemia group, about 68% of pyramidal neurons in the CA1 region were immunostained with neuronal nuclei (NeuN) 4 days after I-R, compared to the vehicle-treated ischemia group. 8-Hydroxy-2′-deoxyguanosine (a marker for DNA damage) and 4-hydroxy-2-nonenal (a marker for lipid peroxidation) immunoreactivity in the CA1 region of the EAKS-treated ischemia group were not markedly changed compared to the vehicle-treated ischemia group. In addition, Cu,Zn- and Mn-SOD immunoreactivity in the CA1 region of the EAKS-treated ischemia group were increased compared to the vehicle-treated ischemia group.
Discussion: Repeated supplements of EAKS could protect neurons against ischemic damage, showing that DNA damage and lipid peroxidation are attenuated and SODs are increased in the ischemic CA1 region.
Introduction
Ischemic stroke is a major cause of morbidity and mortality. Reperfusion in ischemic brain tissue leads to cell death by influx of Ca++ to the intracellular region and production of large amounts of reactive oxygen species (ROS) (CitationSevanian et al., 1983; CitationAu et al., 1985; CitationMcCord et al., 1985; CitationDawson et al., 1992).
Mitochondria produce ATP by utilizing about 90% of O2 taken up by neurons. However, excessive oxygen exposure after ischemia/reperfusion amplifies the generation of mitochondrial free radicals (CitationZhang et al., 1990; CitationRadi et al., 1994). ROS involve free radicals that contain one or more unpaired electrons. ROS-induced oxidative stress has been implicated in variously pathological conditions: cancer, diabetes, ischemia/reperfusion, aging and neuropsychiatric disorders (CitationLohr, 1991; CitationSayre et al., 2001; CitationIrmak et al., 2003; CitationJenner, 2003; CitationDalle-Donne et al., 2006). Accumulation of ROS induces oxidative damage, which involves cellular proteins, lipids, nucleic acids and DNA after an ischemic insult, which leads to neuronal death (CitationRadi et al., 1993; CitationGiulivi et al., 1995).
The ROS are quenched by antioxidants and converted to nontoxic compounds by free-radical-scavenging enzymes. For example, superoxide dismutase (SOD) converts O2− to H2O2, and catalase (CAT) and glutathione peroxidase (Gpx) convert H2O2 to H2O under physiological conditions (CitationHall & Braughler, 1989; CitationCrack et al., 2003; CitationGordon et al., 2005). The physiological balance between oxidants and antioxidants is disrupted, and it leads to ischemic cell death through multiple injury mechanisms, such as Ca++ overload, mitochondrial inhibition and inflammation (CitationCoyle & Puttfarcken, 1993).
The Mongolian gerbil has been widely used as a model of transient cerebral ischemia because the animal does not have interconnections between the carotid and vertebro-basilar circulation. Transient cerebral ischemia results in neuronal death in some specific vulnerable regions such as the hippocampus, neocortex and striatum (CitationKirino, 1994; CitationHoriguchi et al., 2002; CitationJi et al., 2007).
On the other hand, in vitro and in vivo therapies for brain disorders, including ischemic stroke, Alzheimer’s disease, Parkinson’s disease and epilepsy, are still under investigation using various extracts from plants (CitationSaberi et al., 2008; CitationDhanasekaran et al., 2009; CitationRajasankar et al., 2009; CitationNade et al., 2010; CitationBora et al., 2011; CitationLi et al., 2011; CitationZheng et al., 2011). Alpinia katsumadai (Zingiberaceae), which was identified by the National Plant Quarantine Service in Korea, has been used as a traditional Chinese medicine. The plant contains the following compounds: chalcone, flavonoids, diarylheptanoids, monoterpenes, sesquiterpenoids, stilbenes, and labdanes (CitationKimura et al., 1968; CitationYushiro et al., 1968; CitationLawrence et al., 1972; CitationSaiki et al., 1978; CitationBrown & Rice-Evans, 1998; CitationKim et al., 1998; CitationYang et al., 1999). It has been reported that the extract from Alpinia katsumadai seed shows cytotoxic activity against the HepG2, MCF-7 and MAD-MB-435 cell lines, and suppresses topical pruritis, and enhances antioxidant activities (CitationLee et al., 2003; CitationChoi et al., 2009; CitationHua et al., 2009). It was also reported that Alpinia katsumadai seeds showed neuroprotective effects against glutamate-induced neurotoxicity and reactive oxygen species (ROS) generation in mouse hippocampal HT22 cells (CitationJeong et al., 2007).
Therefore, in the present study, we investigated neuroprotective effects of extract of Alpinia katsumadai seed (EAKS) in the hippocampal CA1 region using a gerbil model of transient cerebral ischemia. In addition, we observed the effects of EAKS on DNA damage, lipid peroxidation and oxidative stress in the ischemic CA1 region.
Materials and methods
Preparation of EAKS
Alpinia katsumadai seeds were collected during 1 week in Kangwon province (South Korea), in October 2010, by Hua Li (the first author). The seeds were identified and authenticated by professor J. D. Kim, and a voucher specimen was deposited at the Regional Innovation Center, Hallym University, Chuncheon, Korea. The method of preparation of EAKS was reported previously by CitationHwang et al. (2004). In brief, for the preparation of the ethanol EAKS, Alpinia katsumadai seeds were washed with distilled water and air-dried at 60°C and ground into fine powder. The powder was dispersed in 75% ethanol, and refluxed for 1 h at 50°C. This extraction procedure was repeated three times. The ethanol extract was dried under vacuum.
Animals
Male Mongolian gerbils (Meriones unguiculatus) were obtained from the Experimental Animal Center, Hallym University, Chuncheon, South Korea. The animals were used at 6 months (B.W., 65–75 g) of age. The animals were housed in a conventional state under adequate temperature (23°C) and humidity (60%) control with a 12 h light/12 h dark cycle (light on at 9 a.m. and light off at 9 p.m.), and provided with free access to water and food. The procedures for handling animals and their care conformed to the guidelines that are in compliance with current international laws and policies (NIH Guide for the Care and Use of Laboratory Animals, NIH Publication No. 85-23, 1985, revised 1996) and were approved (approval no.: Hallym-1-35) by the Hallym’s Medical Center Institutional Animal Care and Use Committee (IACUC). All of the experiments were conducted to minimize the number of animals used and suffering caused by such procedures.
Treatment with EAKS
The animals were divided into the following groups: sham-operated gerbils (sham group), vehicle-treated ischemia gerbils (vehicle-ischemia group), 25 mg/kg EAKS-treated ischemia gerbils (25 mg/kg EAKS-ischemia group), and 50 mg/kg EAKS-treated ischemia gerbils (50 mg/kg-ischemia group). The EAKS was orally administered using the feeding needle once a day for one week before transient ischemia. We adapted oral administration of 25 and 50 mg/kg EAKS, because in traditional medicine, EAKS has been taken orally and there are no data about the absorption and metabolism about EKAS (CitationYang et al., 2009). In addition, a recent study reported that pretreatment with various concentrations (5, 10, 20 and 50 µg/mL of EAKS) did not show any toxicity in RAW264.7 cells (CitationLee et al., 2012).
Induction of transient cerebral ischemia
The animals were anesthetized with a mixture of 2.5% isoflurane (Baxtor, Deerfield, IL) in 33% oxygen and 67% nitrous oxide. Bilateral common carotid arteries were isolated and occluded using non-traumatic aneurysm clips for transient cerebral ischemic damage. The complete interruption of blood flow was confirmed by observing the central artery in retina using an ophthalmoscope. After 5 min of occlusion, the aneurysm clips were removed from the common carotid arteries. The body (rectal) temperature under free-regulating or normothermic (37 ± 0.5°C) conditions was monitored with a rectal temperature probe (TR-100; Fine Science Tools, Foster City, CA) and maintained using a thermometric blanket before, during and after the surgery until the animals completely recovered from anesthesia. Thereafter, animals were kept on the thermal incubator (Mirae Medical Industry, Seoul, South Korea) to maintain the body temperature of animals until the animals were euthanized. Sham-operated animals were subjected to the same surgical procedures except that the common carotid arteries were not occluded.
Immunohistochemistry
The sham-group, vehicle-ischemia group and EAKS-ischemia group (n = 7 at each time) were scarified at 3 h, 12 h, 2 days and 4 days after the ischemic surgery for histology. The animals were anesthetized with urethane (Sigma) and perfused transcardially with 0.1 M phosphate-buffered saline (PBS, pH 7.4) followed by 4% paraformaldehyde in 0.1 M phosphate-buffer (PB, pH 7.4). The brains were removed and postfixed in the same fixative for 6 h. The brain tissues were cryoprotected by infiltration with 30% sucrose overnight. Thereafter, frozen tissues were serially sectioned on a cryostat (Leica, Wetzlar, Germany) into 30 µm coronal sections and then the sections were collected into six-well plates containing PBS.
To accurately ascertain the degree of neuroprotection, antioxidant activation and lipid peroxidation after vehicle- or EAKS treatment, immunohistochemistry was processed under the same conditions. The sections were sequentially treated with 0.3% hydrogen peroxide (H2O2) in PBS for 30 min and 10% normal goat or rabbit serum in 0.05 M PBS for 30 min. They were next incubated with neuron-specific soluble nuclear antigen (NeuN, diluted 1:800, Chemicon, international, Temecular, CA), 4-hydroxy-2-nonenal (HNE, diluted 1:1000, Alexis Biochemicals, San Diego, CA), Cu,Zn superoxide dismutase (Cu,Zn-SOD, diluted 1:1000, Calbiochem, USA), and Mn superoxide dismutase (Mn-SOD, diluted 1:1000, Calbiochem, USA) overnight at 4°C, and subsequently exposed to biotinylated horse anti-mouse IgG, goat anti-sheep IgG and streptavidin peroxidase complex (diluted 1:200, Vector, Burlingame, USA). They were visualized by staining with 3,3′-diaminobenzidine (Sigma) in 0.1 M Tris-HCl buffer (pH 7.2) and mounted on gelatin-coated slides. After dehydration the sections were mounted with Canada Balsam (Kanto).
On the other hand, to confirm the DNA damage, denaturation was conducted with the following protocol. For 8-hydroxy-2′-deoxyguanosine (8-OHdG, Chemicon, international, Temecular, CA) immunostaining to visualize 8-OHdG-labeled nuclei: 2 h incubation in 50% formamide/2X SSC (0.3 M NaCl, 0.03 M sodium citrate) at 65°C, 30-min incubation in 2 N HCl at 37°C, and 10-min rinse in 0.1 M boric acid (pH 8.5). After this, the sections were incubated in the mixture of mouse anti-8-OHdG (diluted 1:300) for 48 h at 4°C. They were then incubated with biotinylated rabbit anti-goat IgG and streptavidin peroxidase complex (diluted 1:200, Vector, Burlingame, USA). They were visualized by staining with 3,3′-diaminobenzidine (Sigma) in 0.1 M Tris-HCl buffer (pH 7.2) and mounted on gelatin-coated slides. After dehydration, the sections were mounted with Canada Balsam (Kanto).
In order to quantitatively analyse NeuN immunoreactivity, digital images of the hippocampus were captured with an AxioM1 light microscope (Carl Zeiss, Germany) equipped with a digital camera (Axiocam, Carl Zeiss, Germany) connected to a PC monitor. NeuN-immunoreactive neurons were counted in a 250 × 250 µm square applied approximately at the center of the CA1 region using an image analysing system (software: Optimas 6.5, CyberMetrics, Scottsdale, AZ). According to anatomical landmarks corresponding to AP −1.4 to −1.9 mm of the gerbil brain atlas, the studied tissue sections were selected with 300-µm interval, and cell counts were obtained by averaging the counts from the sections taken from each animal. A ratio of the count was calibrated as %.
Western blot analysis
To examine the effects of EAKS on Cu,Zn-SOD and Mn-SOD levels in the ischemic CA1 region, animals (n = 5 in each group) were used for western blot analysis at designated times (sham, 3 h, 12 h, 2 days and 4 days after the ischemic surgery) after the ischemic surgery. After sacrificing them and removing the hippocampus, it was serially and transversely cut into a thickness of 400 µm on a vibratome (Leica, Germany), and the hippocampal CA1 region was then dissected with a surgical blade. The tissues were homogenized in 50 mM PBS (pH 7.4) containing EGTA (pH 8.0), 0.2% NP-40, 10 mM EDTA (pH 8.0), 15 mM sodium pyrophosphate, 100 mM β-glycerophosphate, 50 mM NaF, 150 mM NaCl, 2 mM sodium orthvanadate, 1 mM PMSF and 1 mM DTT. After centrifugation, the protein levels in the supernatants were determined using a Micro BCA protein assay kit with bovine serum albumin as a standard (Pierce Chemical, USA). Aliquots containing 50 µg of total protein were boiled in loading buffer containing 250 mM Tris (pH 6.8), 10 mM DTT, 10% SDS, 0.5% bromophenol blue and 50% glycerol. The aliquots were then loaded onto a suitable polyacrylamide gel. After electrophoresis, the gels were transferred to nitrocellulose transfer membranes (Pall Corp, East Hills, NY, USA). To reduce background staining, the membranes were incubated with 5% non-fat dry milk in TBS containing 0.1% Tween 20 for 45 min, followed by incubation with Cu,Zn-SOD (diluted 1:1000, Calbiochem, USA) or Mn-SOD (diluted 1:1000, Calbiochem, USA) overnight at 4°C and subsequently exposed to peroxidase-conjugated horse anti-sheep IgG (Santa Cruz, USA) and an ECL kit (Amersham, UK). The result of western blot analysis was scanned, and densitometric analysis for the quantification of the bands was done using Scion Image software (Scion Corp., Frederick, MD), which was used to count relative optical density (ROD): A ratio of the ROD was calibrated as %, with sham-group designated as 100%.
Statistical analysis
Data are expressed as the mean ± SEM. Differences of the means among the groups were statistically analysed by analysis of variance (ANOVA) with a post hoc Bonferroni’s multiple comparison test in order to elucidate ischemia-related differences among experimental groups. Statistical significance was considered at P < 0.05.
Results
Neuroprotection
In the sham group, NeuN-immunoreactive neurons were easily detected in the hippocampal formation ( and ). Neuronal death was well found only in the CA1 region among hippocampal subregions in the vehicle-ischemia group at 4 days postischemia ( and ): About 12% of CA1 neurons were stained with NeuN ().
Figure 1. NeuN immunohistochemistry in the CA1 region of the sham (A, B), vehicle-ischemia (C, D), 25 mg/kg EAKS-ischemia (E, F) and 50 mg/kg EAKS-ischemia (G, H) groups at 4 days postischemia. In the vehicle-ischemia and 25 mg/kg EAKS-ischemia groups, NeuN-positive neurons (asterisk) are rare in the CA1 region (arrow). However, with the 50 mg/kg EAKS-ischemic group, NeuN-positive neurons are abundant in the CA1 region. SO, stratum oriens; SP, stratum pyramidale; SR, stratum radiatum. Scale bar = 50 µm. G: Relative analysis in the number of NeuN-positive neurons in each group 4 days postischemia (n = 7 per group; *P < 0.05, significantly different from the vehicle-ischemia group). The bars indicate the means ± SEM.
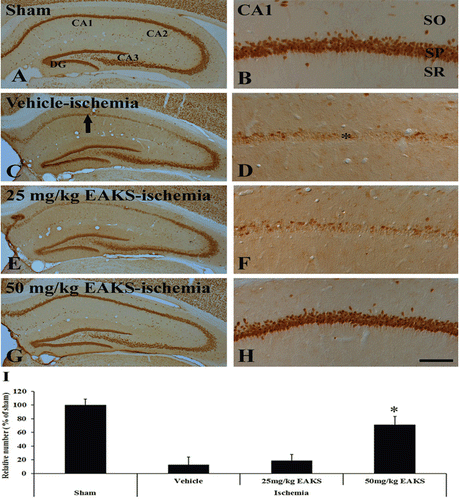
In the 25 mg/kg EAKS-ischemia group at 4 days postischemia, we could not find a significant neuroprotection compared to the 50 mg/kg EAKS-ischemia group ( and ): The number of NeuN-positive CA1 neurons was similar to the vehicle-ischemia group (). However, in the 50 mg/kg EAKS-ischemia group, many NeuN positive neurons were found 4 days after ischemia/reperfusion ( and ). About 67% of the CA1 pyramidal neurons were protected from ischemic damage by 50 mg/kg EAKS administration ().
DNA damage
To examine DNA damage, 8-OHdG immunoreactivity was evaluated (). In the vehicle-sham group, 8-OHdG immunoreactivity was easily detected in the stratum pyramidale of the CA1 region (). 8-OHdG immunoreactivity in the CA1 region in the vehicle-ischemia group was markedly changed with time. At 3 h postischemia, 8-OHdG immunoreactivity was increased compare to the sham group (). 8-OHdG immunoreactivity was highest at 2 days postischemia. At this time point, 8-OHdG immunoreactivity was expressed in non-pyramidal cells in the strata oriens and radiatum of the ischemic CA1 region (). Thereafter, 8-OHdG immunoreactivity in the ischemic CA1 region was decreased with time after ischemia/reperfusion ( and ). At 4 days postischemia, 8-OHdG immunoreactivity was decreased in the stratum pyramidale and non-pyramidal cells ().
Table 1. Semi-quantifications of 8-OHdG immunoreactivity in the CA1 region in the vehicle-ischemia and 50 mg/kg EAKS-ischemia groups.
Figure 2. Immunohistochemistry for 8-OHdG in the CA1 region in the vehicle-ischemia (A, C, E, G, I) and 50 mg/kg EAKS-ischemia (B, D, F, H, J) groups at sham (A, B), 3 h (C, D), 12 h (E, F), 2 days (G, H) and 4 days (I, J) postischemia. Weak 8-OHdG immunoreactivity is detected in the stratum pyramidale (SP, asterisk) of the CA1 region of the vehicle-sham group. In the vehicle-ischemia group, the immunoreactivity is increased at 3 h postischemia (asterisk) and highest at 12 h postischemia: At this time point, 8-OHdG immunoreactivity is expressed in non-pyramidal cells (arrows). Thereafter, 8-OHdG immunoreactivity is decreased with time after ischemia/reperfusion. In the EAKS-ischemia group, 8-OHdG immunoreactivity is not markedly changed compared to the sham group. SO, stratum oriens; SR, stratum radiatum. Scale bar = 50 µm.
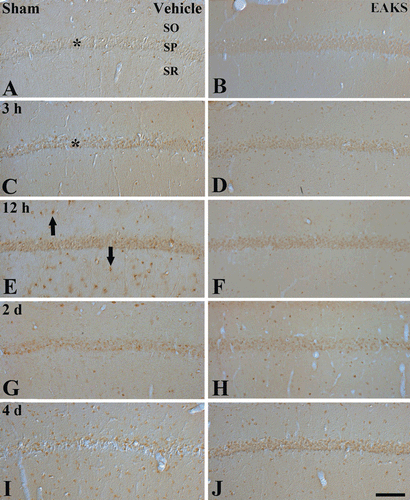
In the 50 mg/kg EAKS-sham group, 8-OHdG immunoreactivity in the stratum pyramidale was higher than the vehicle-sham group (). In the EAKS-ischemia group, 8-OHdG immunoreactivity was slightly decreased with time; however, the immunoreactivity was much higher than the vehicle-ischemia group (, and 2H). At 4 days postischemia, 8-OHdG immunoreactivity in the stratum pyramidale was similar to the sham group ().
Lipid peroxidation
To confirm lipid peroxide activity, change in HNE immunoreactivity was evaluated. Weak HNE immunoreactivity was found in the stratum pyramidale of the CA1 region of the vehicle-sham group (). In the vehicle-ischemia group, HNE immunoreactivity was distinctively altered after ischemia/reperfusion (). At 3 h postischemia, HNE immunoreactivity was increased compared to the vehicle-sham group (). At 12 h postischemia, HNE immunoreactivity was highest in the stratum pyramidale of the ischemic CA1 region (). Thereafter, HNE immunoreactivity was decreased with time after ischemic damage ( and ). Four days after ischemia/reperfusion, HNE immunoreactivity in the stratum pyramidale was marked decreased ().
Table 2. Semi-quantifications of HNE immunoreactivity in the CA1 region in the vehicle-ischemia and 50 mg/kg EAKS-ischemia groups.
Figure 3. HNE immunohistochemistry in the CA1 region in the vehicle-ischemia (A, C, E, G, I) and 50 mg/kg EAKS-ischemia (B, D, F, H, J) groups at sham (A, B), 3 h (C, D), 12 h (E, F), 2 days (G, H) and 4 days (I, J) postischemia. In the vehicle-sham group, weak HNE immunoreactivity is detected in the stratum pyramidale (SP, asterisk). In the vehicle-ischemia group, HNE immunoreactivity is increased at 3 h (asterisk) and highest at 12 h (asterisk) postischemia. Four days after ischemia/reperfusion, HNE immunoreactivity in the SP (asterisk) is very low. However, in the EAKS-ischemia group, HNE immunoreactivity is not markedly changed compared to the sham group. SO, stratum oriens; SR, stratum radiatum. Scale bar = 50 µm.
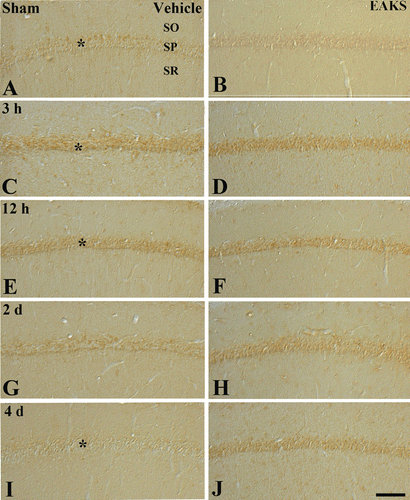
In the 50 mg/kg EAKS-sham group, HNE immunoreactivity in the stratum pyramidale was similar to the vehicle-sham group (). HNE immunoreactivity in the 50 mg/kg EAKS-ischemia group was maintained with time (, , and 3J).
Cu, Zn-SOD immunoreactivity and its protein level
Change in Cu,Zn-SOD immunoreactivity in the CA1 region was observed (). Weak Cu,Zn-SOD immunoreactivity was detected in the stratum pyramidale of the CA1 region of the vehicle-sham group (). In the vehicle-ischemia group, Cu,Zn-SOD immunoreactivity was increased and highest 12 h after ischemia/reperfusion ( and ). Thereafter, Cu,Zn-SOD immunoreactivity was decreased with time (). At 4 days postischemia, Cu,Zn-SOD immunoreactivity was weakly detected in pyramidal cells; however, strong Cu,Zn-SOD immunoreactivity was expressed in non-pyramidal cells in the strata oriens and radiatum ().
Figure 4. Immunohistochemical staining for Cu,Zn-SOD in the CA1 region in the vehicle-ischemia (A, C, E, G, I) and 50 mg/kg EAKS-ischemia (B, D, F, H, J) groups at sham (A, B), 3 h (C, D), 12 h (E, F), 2 days (G, H) and 4 days (I, J) postischemia. Cu,Zn-SOD immunoreactivity is well detected in the stratum pyramidale (SP, asterisk) in the sham group. In the vehicle-ischemia group, the immunoreactivity is increased (asterisk) at 12 h postischemia, and decreased at 2 days postischemia. Four days after ischemia/reperfusion, Cu,Zn-SOD immunoreactivity in the SP is very low, and Cu,Zn-SOD immunoreactivity is expressed in non-pyramidal cells (arrows). In the EAKS-sham group, Cu,Zn-SOD immunoreactivity is similar to the vehicle-sham group; however, in the EAKS-ischemia group, Cu,Zn-SOD immunoreactivity (asterisk) is much higher than the vehicle-ischemia group. SO, stratum oriens; SR, stratum radiatum. Scale bar = 50 µm.
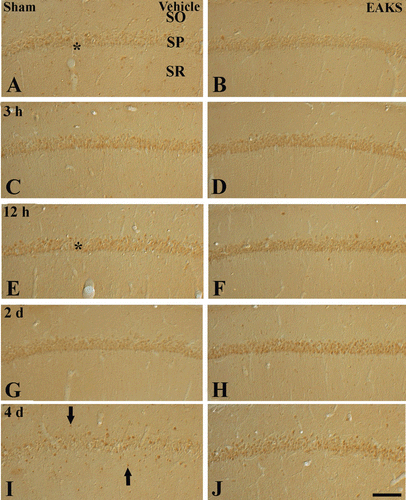
In the EAKS-sham group, Cu,Zn-SOD immunoreactivity in the CA1 region was similar to the vehicle-sham group (). In the EAKS-ischemia group, Cu,Zn-SOD immunoreactivity was also increased with time and higher than the vehicle-sham group ( and ): At 2 days postischemia, the immunoreactivity was much higher than the corresponding vehicle-ischemia group (). Four days after ischemia/reperfusion, Cu,Zn-SOD immunoreactivity was also higher than the corresponding vehicle-ischemia; however, Cu,Zn-SOD immunoreactivity was not expressed in non-pyramidal cells ().
Western blot analysis showed that changes in Cu,Zn-SOD levels were paralleled the immunohistochemical findings in both groups (). Cu,Zn-SOD levels in the EAKS-ischemia group were increased with time: At 2 days postischemia, Cu,Zn-SOD level was 1.7-fold compared to the corresponding vehicle-ischemia group. Four days after ischemia/reperfusion, Cu,Zn-SOD levels in both groups were decreased compared to both the 2 days postischemia groups.
Figure 5. Mn-SOD immunohistochemistry in the CA1 region in the vehicle-ischemia (A, C, E, G, I) and 50 mg/kg EAKS-ischemia (B, D, F, H, J) groups at sham (A, B), 3 h (C, D), 12 h (E, F), 2 days (G, H) and 4 days (I, J) postischemia. Weak Mn-SOD immunoreactivity is found in the stratum pyramidale (SP, asterisk) in the sham group. In the vehicle-ischemia group, the immunoreactivity is not changed by 12 h postischemia; however, at 2 days postischemia, Mn-SOD immunoreactivity is slightly increased in the SP (asterisk). Four days after ischemia/reperfusion, Mn-SOD immunoreactivity in the SP is very low; however, Mn-SOD immunoreactivity is increased in non-pyramidal cells (arrows). In the EAKS-sham and ischemia group, Mn-SOD immunoreactivity is similar to the vehicle-sham and ischemia group; at 2 days postischemia, the immunoreactivity (asterisk) is much higher than the vehicle-ischemia group. SO, stratum oriens; SR, stratum radiatum. Scale bar = 50 µm.
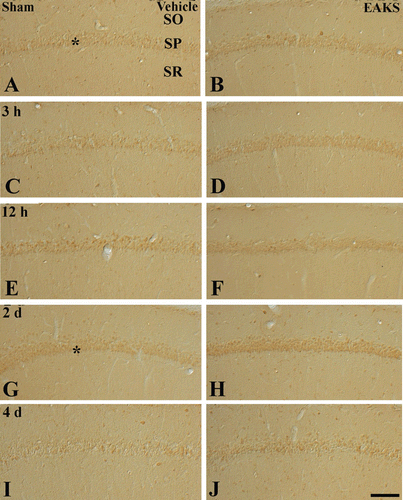
Mn-SOD immunoreactivity and its protein level
Weak Mn-SOD immunoreactivity was found in the stratum pyramidale of the CA1 region of the vehicle-sham group (). In the vehicle-ischemia group, Mn-SOD immunoreactivity was not changed by 12 h postischemia ( and ). At two days postischemia, Mn-SOD immunoreactivity was slightly increased (), and the immunoreactivity was decreased to the sham level four days after ischemia/reperfusion (). At this time point, Mn-SOD immunoreactivity was expressed in non-pyramidal cells.
Figure 6. Western blot analysis of Cu,Zn-SOD (A) and Mn-SOD (B) levels in the CA1 region of the vehicle- and EAKS-ischemia group. Relative optical density (ROD) as percentage of the immunoblot band is represented (n = 5 per group; *P < 0.05, significantly different from the vehicle-sham group, #P < 0.05, significantly different from the preceding group, †P < 0.05, significantly different from the vehicle-ischemia group). Bars indicate mean ± SEM.
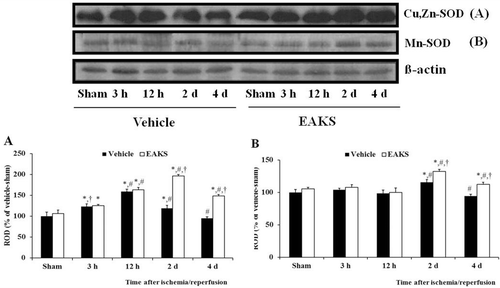
In the EAKS-sham group, Mn-SOD immunoreactivity was also similar to the vehicle-sham group (). In the EAKS-ischemia group, Mn-SOD immunoreactivity was also similar to the vehicle-sham group by 12 h post ischemia ( and ). Two days after ischemia/reperfusion, Mn-SOD immunoreactivity was markedly increased (), and the immunoreactivity was decreased at four days post ischemia ().
Changes in Mn-SOD protein levels also paralleled the immunohistochemical findings (). Mn-SOD levels in the EAKS-ischemia group were similar to the vehicle-sham group by 12 h postischemia. Two days after ischemia/reperfusion, Mn-SOD level was somewhat increased compared to the corresponding vehicle-ischemia group. At four days postischemia, Mn-SOD levels in both the groups were decreased compared to both the two days postischemia groups.
Discussion
The effects of EAKS on transient cerebral ischemia in the gerbil hippocampal CA1 region were examined. We found that 50 mg/kg EAKS protected about 68% of the CA1 neurons from ischemic damage at four days postischemia. This is the first report to show that EAKS has neuroprotective effects in a gerbil model of transient cerebral ischemia. It has been reported that EAKS extract inhibits H2O2-induced apoptosis and enhances the viability of Chinese hamster lung fibroblast (V79-4) cells (CitationLee et al., 2003).
Increased oxygen during ischemia/reperfusion induces the uncoupling of electrons, and causes massive free radical accumulation. ROS, particularly superoxide anions, play a key role in neuronal cell death after cerebral ischemia (CitationMurakami et al., 1998; CitationFujimura et al., 1999; CitationSugawara et al., 2002). Superoxide anion radical (O2−) combines with H+ to produce H2O2 (spontaneously or by superoxide dismutases). H2O2 generate hydroxyl radical (OH·) by the Fenton reaction (CitationMattson et al., 2000).
8-OHdG as a marker for oxidative DNA damage is associated with a massive increase of ROS and it can be a target of promutagenic lesions. In the present study, 8-OHdG immunoreactivity was dramatically changed in the CA1 region of the vehicle-ischemia group after ischemia/reperfusion. It has been reported that 8-OHdG levels in the gerbil hippocampus increases significantly for at least 12 h after ischemia/reperfusion (CitationWon et al., 1999). However, in the EAKS-treated group, 8-OHdG immunoreactivity was not changed compared to the vehicle-ischemia group. It was reported that the morphology and DNA profile of EAKS-treated H2O2-induced apoptotic cells were very similar to control cells (CitationLee et al., 2003).
HNE is produced during oxidation of polyunsaturated fatty acid lipid membrane; it is able to inhibit DNA, RNA and protein biosynthesis (CitationSuc et al., 1998). It was reported that NHE immunoreactivity was dramatically increased in the CA1 region of the gerbil 12 h after transient cerebral ischemia (CitationHwang et al., 2008). In our present study, we also found that NHE immunoreactivity in the vehicle-ischemia group was distinctively altered in the CA1 region after ischemic damage. However, in the EAKS-ischemia group, NHE immunoreactivity was not markedly changed in the CA1 region after ischemia/reperfusion.
To elucidate the mechanism of reduction of oxidative damage in the EAKS-ischemia group, Cu,Zn-SOD and Mn-SOD were investigated. Cu,Zn-SOD immunoreactivity and its protein levels in the EAKS-ischemia group were much higher than the vehicle-ischemia group; however, Mn-SOD immunoreactivity and protein levels in the EAKS-ischemia group were slightly increased compared to the vehicle-ischemia group. It was reported that EAKS increased antioxidant enzymes such as SOD, CAT and Gpx in V79-4 cells exposed to H2O2 (CitationLee et al., 2003).
SODs catalyze the transformation of superoxide radical into hydrogen peroxide, and Cu,Zn-SOD and Mn-SOD presents in the cytoplasm and mitochondrial matrix, respectively. Cu,Zn-SOD has been reported to prevent the early loss of DNA repair enzymes after focal cerebral ischemia in mice (CitationFujimura et al., 1999), and it attenuates DNA fragmentation after global cerebral ischemia in rats (CitationChan et al., 1998). In addition, increased Cu,Zn-SOD reduces oxidative DNA damage and subsequent DNA-fragmented cell death after photothrombotic ischemia in Cu,Zn-SOD transgenic mice (CitationKim et al., 2001). Mn-SOD is a mitochondrial superoxide dismutase that scavenges superoxide anions. Mutant mice with Mn-SOD deficiency have a significant increase in superoxide anion production under normal physiological conditions and especially after cerebral ischemia (CitationMurakami et al., 1998). Furthermore, Mn-SOD deficient mutant mice show an increased infarct size, more severe neurological deficits, enhanced cytochrome c translocation, caspase activation, and DNA fragmentation after cerebral ischemia (CitationFujimura et al., 1999).
Conclusion
The present study indicates that repeated oral administration of EAKS protects hippocampal neurons from ischemic damage in a gerbil model of transient cerebral ischemia. These effects might be strongly associated with antioxidant effects.
Acknowledgments
The authors would like to thank Mr. Sung Auk Lee and Ms. Hyun Souk Kim for their technical help in this study.
Declaration of interest
This research was supported by Basic Science Research Program through the National Research Foundation of Korea (NRF) funded by the Ministry of Education, Science and Technology (NO 2011-0022812 and 2011-0007307). The authors report no conflict of interest.
References
- Au AM, Chan PH, Fishman RA. (1985). Stimulation of phospholipase A2 activity by oxygen-derived free radicals in isolated brain capillaries. J Cell Biochem, 27, 449–453.
- Bora KS, Shri R, Monga J. (2011). Cerebroprotective effect of Ocimum gratissimum against focal ischemia and reperfusion-induced cerebral injury. Pharm Biol, 49, 175–181.
- Brown JE, Rice-Evans CA. (1998). Luteolin-rich artichoke extract protects low density lipoprotein from oxidation in vitro. Free Radic Res, 29, 247–255.
- Chan PH, Kawase M, Murakami K, Chen SF, Li Y, Calagui B, Reola L, Carlson E, Epstein CJ. (1998). Overexpression of SOD1 in transgenic rats protects vulnerable neurons against ischemic damage after global cerebral ischemia and reperfusion. J Neurosci, 18, 8292–8299.
- Choi JK, Kim KM, Kim DK, Yeom MH, Koh JY, Jung SJ, Kim HJ, Oh SH, Kim SY, Lee CH. (2009). Topical anti-inflammatory and antipruritic effects of Alpinia katsumadai extracts. J Dermatol Sci, 53, 81–84.
- Coyle JT, Puttfarcken P. (1993). Oxidative stress, glutamate, and neurodegenerative disorders. Science, 262, 689–695.
- Dalle-Donne I, Rossi R, Colombo R, Giustarini D, Milzani A. (2006). Biomarkers of oxidative damage in human disease. Clin Chem, 52, 601–623.
- Crack PJ, Taylor JM, de Haan JB, Kola I, Hertzog P, Iannello RC. (2003). Glutathione peroxidase-1 contributes to the neuroprotection seen in the superoxide dismutase-1 transgenic mouse in response to ischemia/reperfusion injury. J Cereb Blood Flow Metab, 23, 19–22.
- Dawson TM, Dawson VL, Snyder SH. (1992). A novel neuronal messenger molecule in brain: The free radical, nitric oxide. Ann Neurol, 32, 297–311.
- Dhanasekaran M, Holcomb LA, Hitt AR, Tharakan B, Porter JW, Young KA, Manyam BV. (2009). Centella asiatica extract selectively decreases amyloid beta levels in hippocampus of Alzheimer’s disease animal model. Phytother Res, 23, 14–19.
- Fujimura M, Morita-Fujimura Y, Kawase M, Copin JC, Calagui B, Epstein CJ, Chan PH. (1999). Manganese superoxide dismutase mediates the early release of mitochondrial cytochrome C and subsequent DNA fragmentation after permanent focal cerebral ischemia in mice. J Neurosci, 19, 3414–3422.
- Giulivi C, Boveris A, Cadenas E. (1995). Hydroxyl radical generation during mitochondrial electron transfer and the formation of 8-hydroxydesoxyguanosine in mitochondrial DNA. Arch Biochem Biophys, 316, 909–916.
- Gordon KB, Macrae IM, Carswell HV. (2005). Effects of 17beta-oestradiol on cerebral ischaemic damage and lipid peroxidation. Brain Res, 1036, 155–162.
- Hall ED, Braughler JM. (1989). Central nervous system trauma and stroke. II. Physiological and pharmacological evidence for involvement of oxygen radicals and lipid peroxidation. Free Radic Biol Med, 6, 303–313.
- Horiguchi T, Shima H, Suga S, Ogino M, Shimizu K, Toya S, Nagao M, Kawase T. (2002). Transient forebrain ischemia induces expression of serine/threonine protein phosphatase 1 mRNA in the vulnerable regions of gerbil brain. Neurosci Lett, 325, 115–118.
- Hua SZ, Luo JG, Wang XB, Wang JS, Kong LY. (2009). Two novel monoterpene-chalcone conjugates isolated from the seeds of Alpinia katsumadai. Bioorg Med Chem Lett, 19, 2728–2730.
- Hwang IK, Lim SS, Yoo KY, Lee YS, Kim HG, Kang IJ, Kwon HJ, Park J, Choi SY, Won MH. (2008). A phytochemically characterized extract of Cordyceps militaris and cordycepin protect hippocampal neurons from ischemic injury in gerbils. Planta Med, 74, 114–119.
- Hwang IK, Yoo KY, Kim DS, Jeong YK, Kim JD, Shin HK, Lim SS, Yoo ID, Kang TC, Kim DW, Moon WK, Won MH. (2004). Neuroprotective effects of grape seed extract on neuronal injury by inhibiting DNA damage in the gerbil hippocampus after transient forebrain ischemia. Life Sci, 75, 1989–2001.
- Irmak MK, Fadillioglu E, Sogut S, Erdogan H, Gulec M, Ozer M, Yagmurca M, Gozukara ME. (2003). Effects of caffeic acid phenethyl ester and alpha-tocopherol on reperfusion injury in rat brain. Cell Biochem Funct, 21, 283–289.
- Jeong GS, Li B, Lee DS, Byun E, Kang DG, Lee HS, Kim YC. (2007). Cytoprotective constituents of Alpinia katsumadai seeds against glutamate-induced oxidative injury in HT22 Cells. Nat Prod Sci, 13, 268–271.
- Jenner P. (2003). Oxidative stress in Parkinson’s disease. Ann Neurol, 53 Suppl 3, S26–36; discussion S36.
- Ji X, Li C, Lu Y, Chen Y, Guo L. (2007). Post-ischemic continuous administration of galantamine attenuates cognitive deficits and hippocampal neurons loss after transient global ischemia in gerbils. Neurosci Lett, 416, 92–95.
- Kim GW, Lewén A, Copin J, Watson BD, Chan PH. (2001). The cytosolic antioxidant, copper/zinc superoxide dismutase, attenuates blood-brain barrier disruption and oxidative cellular injury after photothrombotic cortical ischemia in mice. Neuroscience, 105, 1007–1018.
- Kim JH, Kim SH, Lee EJ, Gao J, Wu Z, Mar W, Chang IM. (1998). Radioprotective effect of Lifukang, a Chinese medicinal plants prescription. Nat Prod Sci, 4, 26–31.
- Kimura Y, Takahashi S, Yoshida I. (1968). Studies on the constituents of Alpinia. XII. On the constituents of the seeds of Alpinia katsumadai hayata. I. The structure of cardamomin. Yakugaku Zasshi, 88, 239–241.
- Kirino T. (1994). Cerebral ischemia and neuronal death. No To Hattatsu, 26, 130–135.
- Lawrence BM, Hogg JW, Terhune SJ. (1972). Terpenoids of two amomum species from Thailand. Phytochemistry, 11, 1534–1535.
- Lee MY, Seo CS, Lee JA, Shin IS, Kim SJ, Ha H, Shin HK. (2012). Alpinia katsumadai H(AYATA) seed extract inhibit LPS-induced inflammation by induction of heme oxygenase-1 in RAW264.7 cells. Inflammation, 35, 746–757.
- Lee SE, Shin HT, Hwang HJ, Kim JH. (2003). Antioxidant activity of extracts from Alpinia katsumadai seed. Phytother Res, 17, 1041–1047.
- Li M, Chen M, Huang H, Tao W, Cui J, Xiang H. (2011). Neuroprotective effects of active ingredients isolated from Pegasus laternarius on cultured cerebral neurons. Cell Mol Neurobiol, 31, 73–82.
- Lohr JB. (1991). Oxygen radicals and neuropsychiatric illness. Some speculations. Arch Gen Psychiatry, 48, 1097–1106.
- Mattson MP, Culmsee C, Yu ZF. (2000). Apoptotic and antiapoptotic mechanisms in stroke. Cell Tissue Res, 301, 173–187.
- McCord JM, Roy RS, Schaffer SW. (1985). Free radicals and myocardial ischemia. The role of xanthine oxidase. Adv Myocardiol, 5, 183–189.
- Murakami K, Kondo T, Kawase M, Li Y, Sato S, Chen SF, Chan PH. (1998). Mitochondrial susceptibility to oxidative stress exacerbates cerebral infarction that follows permanent focal cerebral ischemia in mutant mice with manganese superoxide dismutase deficiency. J Neurosci, 18, 205–213.
- Nade VS, Kawale LA, Dwivedi S, Yadav AV. (2010). Neuroprotective effect of Hibiscus rosa sinensis in an oxidative stress model of cerebral post-ischemic reperfusion injury in rats. Pharm Biol, 48, 822–827.
- Radi R, Rodriguez M, Castro L, Telleri R. (1994). Inhibition of mitochondrial electron transport by peroxynitrite. Arch Biochem Biophys, 308, 89–95.
- Radi R, Sims S, Cassina A, Turrens JF. (1993). Roles of catalase and cytochrome c in hydroperoxide-dependent lipid peroxidation and chemiluminescence in rat heart and kidney mitochondria. Free Radic Biol Med, 15, 653–659.
- Rajasankar S, Manivasagam T, Surendran S. (2009). Ashwagandha leaf extract: A potential agent in treating oxidative damage and physiological abnormalities seen in a mouse model of Parkinson’s disease. Neurosci Lett, 454, 11–15.
- Saberi M, Rezvanizadeh A, Bakhtiarian A. (2008). The antiepileptic activity of Vitex agnus castus extract on amygdala kindled seizures in male rats. Neurosci Lett, 441, 193–196.
- Saiki Y, Ishikawa Y, Uchida M, Fukushima S. (1978). Essential oil from Chinese drug ‘caodoukou’, the seeds of Alpinia katsumadai. Phytochemistry, 17, 808–809.
- Sayre LM, Smith MA, Perry G. (2001). Chemistry and biochemistry of oxidative stress in neurodegenerative disease. Curr Med Chem, 8, 721–738.
- Sevanian A, Muakkassah-Kelly SF, Montestruque S. (1983). The influence of phospholipase A2 and glutathione peroxidase on the elimination of membrane lipid peroxides. Arch Biochem Biophys, 223, 441–452.
- Suc I, Meilhac O, Lajoie-Mazenc I, Vandaele J, Jürgens G, Salvayre R, Nègre-Salvayre A. (1998). Activation of EGF receptor by oxidized LDL. FASEB J, 12, 665–671.
- Sugawara T, Noshita N, Lewén A, Gasche Y, Ferrand-Drake M, Fujimura M, Morita-Fujimura Y, Chan PH. (2002). Overexpression of copper/zinc superoxide dismutase in transgenic rats protects vulnerable neurons against ischemic damage by blocking the mitochondrial pathway of caspase activation. J Neurosci, 22, 209–217.
- Won MH, Kang TC, Jeon GS, Lee JC, Kim DY, Choi EM, Lee KH, Choi CD, Chung MH, Cho SS. (1999). Immunohistochemical detection of oxidative DNA damage induced by ischemia-reperfusion insults in gerbil hippocampus in vivo. Brain Res, 836, 70–78.
- Yang J, Dai Y, Xia YF, Huang WZ, Wang ZT. (2009). Alpinia katsumadai Hayata prevents mouse sepsis induced by cecal ligation and puncture through promoting bacterial clearance and downregulating systemic inflammation. Phytother Res, 23, 267–273.
- Yang Y, Kinoshita K, Koyama K, Takahashi K, Tai T, Nunoura Y, Watanabe K. (1999). Two novel anti-emetic principles of Alpinia katsumadai. J Nat Prod, 62, 1672–1674.
- Yushiro K, Shuhiti T, Ikuo Y. (1968). Studies on the constituents of the seeds of Alpinia katsumadai. Yakugaku Zasshi, 88, 239–241.
- Zhang Y, Marcillat O, Giulivi C, Ernster L, Davies KJ. (1990). The oxidative inactivation of mitochondrial electron transport chain components and ATPase. J Biol Chem, 265, 16330–16336.
- Zheng M, Liu C, Pan F, Shi D, Ma F, Zhang Y, Zhang Y. (2011). Protective effects of flavonoid extract from Apocynum venetum leaves against corticosterone-induced neurotoxicity in PC12 cells. Cell Mol Neurobiol, 31, 421–428.