Abstract
Context: Pterospermum acerifolium (L.) Willd (Sterculiaceae) has been traditionally used in the treatment of diabetes mellitus but no scientific data has been published supporting the claimed ethnomedical use.
Objective: The present study was designed to estimate the in silico, in vitro α-amylase inhibition potential and anti-diabetic activity of Pterospermum acerifolium bark.
Materials and methods: In silico studies were performed between human pancreatic α-amylase (HPA) and β-sitosterol by using autodock 4.2 software. In vitro α-amylase inhibition study was carried out with 50% ethanol extract of the bark (PABEE) and its various fractions. The active ethyl acetate fraction (PABEF) was sub-fractionated into three fractions (PABE1, PABE2 and PABE3). Two doses (15 and 30 mg/kg) based on acute toxicity studies, of the above fractions were subjected to antidiabetic screening in vivo by STZ-nicotinamide induced type II diabetic rats.
Results: In silico studies showed the potent inhibition of β-sitosterol on human pancreatic amylase (HPA) with an estimated inhibition constant (Ki) of 269.35 nmol and two hydrogen bond interactions. PABEF showed marked α-amylase inhibition (69.94%) compared to other fractions. Diabetic rats treated with PABE3 (30 mg/kg) reduced the levels of fasting blood glucose, HbA1c, ALT, AST, ALP, triglycerides, total cholesterol, TBARS significantly (p < 0.01) and increased the levels of HDL-C, catalase, GSH, SOD significantly (p < 0.01) as compared to that of diabetic control animals. Histological studies on PABE3 treated group showed remarkable positive changes in β-cells.
Conclusion: The present study confirmed the antihyperglycemic activity along with its status on hepatic biomarkers, antihyperlipidemic and antioxidant properties of Pterospermum acerifolium bark.
Introduction
Diabetes mellitus (DM) is characterized by chronic hyperglycemia resulting from defects in insulin secretion or action, or both, with impaired carbohydrate, lipid and protein metabolism. It is divided into insulin dependent (Type I) and non-insulin dependent (Type II) diabetes mellitus (Aylin et al., Citation2007). Its frequency worldwide is expected to continue to grow by 6% per annum and it is predicted that by 2025 India, China and the United States will have the largest number of people with diabetes and more importantly, type II diabetes that accounts for 90–95% of all diabetes (King et al., Citation1998; Muller, Citation2001). The pathogenesis of type 2 diabetes is complex involving progressive development of insulin resistance in liver and peripheral tissues accompanied by a defective insulin secretion from pancreatic β-cells leading to overt hyperglycemia (Cheng, Citation2005). Currently available therapeutic strategies for treating type II diabetes is limited with generally inadequate efficacy and a number of serious adverse effects (Srinivasan & Ramarao, Citation2007). Many traditional plant treatments for diabetes mellitus are used throughout the world, both in developing and developed countries, but few of the medicinal plant treatments for diabetes have received scientific scrutiny, for which World Health Organization (WHO) has also recommended attention (WHO, Citation1980).
Pterospermum acerifolium (L.) Willd (Sterculiaceae) is a large tree up to 24 m in height and 2.5 m in girth. It is widely distributed in the regions of sub-Himalayan tract and outer valleys from Yamuna eastwards to West Bengal, and in Assam and Manipur, up to an altitude of 1200 m (Mazumder et al., Citation2011). The plant is commonly known as Kanakchampa, Karnikara, Muchukunda and Matsakanda and has traditionally been used as a haemostatic, anti-inflammatory, anthelmintic, laxative and antihyperglycemic, as well as a treatment for blood troubles, small pox, leucorrhoea, leprosy, ulcer, tumors, ear pain and stomachache (Chopra et al., Citation1956; Kirtikar & Basu, Citation1935). A significant number of phytoconstituents have been isolated from all parts of the plant (Dixit et al., Citation2011). However, the antidiabetic potential of Pterospermum acerifolium bark has not yet been extensively studied. Hence, this work is designed to evaluate the in silico, in vitro α-amylase inhibition potential and antidiabetic activity of Pterospermum acerifolium bark.
Materials and methods
Plant material
Pterospermum acerifolium bark collected from the campus of BIT Mesra, Ranchi during August of 2011. The plant material was identified and authenticated by Dr. K. Karthikeyan, taxonomy department of Botanical Survey of India (BSI), Kolkata. A voucher specimen (CNH/48/2012/Tech II/805) was deposited in the herbarium of Department of Pharmaceutical Sciences, BIT Mesra, Ranchi for future reference.
Extraction and fractionation
Powdered bark (1 kg) was extracted with 50% ethanol for 72 h at room temperature and concentrated to yield 74.61 g of PABEE. The extract (25 g) was dissolved in distilled water and extracted successively with hexane (PABHF), chloroform (PABCF) and ethyl acetate (PABEF), and concentrated on rotavapour (Buchi Labortechnik AG, Schweiz) under reduced pressure at 40 °C to yield PABHF (3.46 g), PABCF (5.71 g) and PABEF (14.28 g) fractions.
Molecular docking studies
Amongst the various phytoconstituents reported in the bark of Pterospermum acerifolium, β-sitosterol is the common secondary metabolite which had shown various activities including type II diabetes (Gupta et al., Citation2011). Therefore, β-sitosterol was selected as the ligand for in silico studies.
The autodock 4.2 docking software was used to perform molecular docking simulation between human pancreatic amylase and β-sitosterol. The crystal structure of HPA complexed with acarviostatin (PDB CODE: 3OLE) at 1.55 Å resolution was downloaded from the RCSB Protein Data Bank (http://www.rcsb.org/pdb/home/home.do). MGLTools-1.4.6 was used to prepare protein (protein.pdbqt) and to write grid parameter file (protein.gpf) and docking parameter file (ligand.dpf). Protein preparation includes: (i) removal of water and ions and extraction of co-crystallized ligand; (ii) addition of polar hydrogens; (iii) assignment of AD4 atom type; and finally (iv) assignment of Gasteiger charges. The grid maps representing the native ligand in the actual docking target site were calculated with autogrid4 with box dimension of 60 × 60 × 60 Å and spacing of 0.375 Å by taking the centre of the ligand as the centre of the grid. Docking of the ligand was done with default parameters except keeping 50 runs.
α-Amylase inhibition assay
A modified form of the Sigma-Aldrich (St. Louis, MO) method was used (Ali et al., Citation2006). Briefly, a starch solution (0.5% w/v) was obtained by stirring 0.125 g of potato starch in 25 ml of 20 mM sodium phosphate buffer with 6.7 mM sodium chloride, pH 6.9 at 65 °C for 15 min. Enzyme (2 units/ml) solution was prepared by dissolving α-amylase in ice cold distilled water. In screw cap plastic tubes, 40 μl of plant extracts (1 mg/ml in dimethyl sulfoxide), 160 μl of distilled water and 400 μl of starch solution (0.5% w/v) were mixed. The reaction was started by the addition of 200 μl of enzyme solution. The tubes were incubated at 25 °C for 3 min. The above mixture (200 μl) was removed and added on to a separate tube containing 100 μl of dinitrosalicylic acid (DNS) color reagent solution (96 mM 3,5-dinitrosalicyclic acid, 5.31 M sodium potassium tartarate in 2 M sodium hydroxide) and kept in a water bath at 85 °C for 15 min, cooled and quantified at 540 nm. The α-amylase inhibition was expressed as 100 – % reaction, where the % reaction = (maltose in test/maltose in control) × 100.
Subfractionation of ethyl acetate fraction
The ethyl acetate fraction was further fractionated by column chromatography. Crude fraction (7 g) was mixed with silica gel (mesh 60–120) and slurry was prepared to make the fractions adsorbed with silica gel for uniform mixing. Using dichloromethane as solvent column was packed with silica gel (mesh 100–200). The column was eluted with dichloromethane, followed by methanol: dichloromethane in the regular increased proportion of methanol. Each fraction was tested on TLC plate for its homogeneity and were combined into three fractions and concentrated on a rotavapour (Buchi Labortechnik AG, Schweiz) under reduced pressure at 40 °C to yield the subfractions PABE1 (1.53 g), PABE2 (2.14 g) and PABE3 (2.87 g).
Animals
Healthy male albino rats (Wistar strain 180–200 g) were procured from the animal house of Birla Institute of Technology. They were housed in clean polypropylene cages with free access to standard laboratory pellet diet and water at room temperature with an inverted 12:12 h light–dark cycle and relative humidity of 60%. All the animals were acclimatized for 7 days prior to the experiments. The experimental protocols were approved by the Institutional Animal Ethics Committee (BIT/PH/IAEC/34/2011dt 10.12.2011).
Acute oral toxicity studies and dose fixation
Toxicity studies conducted on female mice based on OECD guidelines 423 for acute oral toxicity-acute toxic class method. Three animals were used in each step. The dose level to be used as the starting dose was selected from one of the four fixed levels, 5, 50, 300 and 2000 mg/kg body weight. For the ethyl acetate fraction (PABEF), 2000 mg/kg was fixed as the initial dose and for the subfractions (PABE1, PABE2 and PABE3) 300 mg/kg was fixed as the initial dose and the test substance was administered in a single dose by using an intragastric tube. Animals were observed individually after dosing at least once during the first 30 min, periodically during the first 24 h, with special attention given during the first 4 h, and daily thereafter, for a total of 14 days.
Induction of diabetes
Streptozotocin (STZ) was dissolved in citrate buffer (pH 4.5) and nicotinamide was dissolved in normal physiological saline. Non-insulin dependent diabetes mellitus was induced in overnight fasted rats by a single intraperitoneal injection of STZ (60 mg/kg b.w.): 15 min later, the rats were given the intraperitoneal administration of nicotinamide (120 mg/kg b.w.). Hyperglycemia was confirmed by the elevated glucose levels determined at 72 h. Animals with blood glucose concentration more than 250 mg/dL were included in the study (Pellegrino et al., Citation1998).
Experimental design
Animals were divided in to 11 groups and each group consisted of 6 animals.
Group I: Normal control (2% Tween 80; 1 ml/kg b.w.)
Group II: Diabetic control rats receiving sterile water
Group III: PABEF1; Diabetic animals treated with PABEF 200 mg/kg b.w.
Group IV: PABEF2; Diabetic animals treated with PABEF 400 mg/kg b.w.
Group V: PABE1-1; Diabetic animals treated with PABE1 15 mg/kg b.w.
Group VI: PABE1-2; Diabetic animals treated with PABE1 30 mg/kg b.w.
Group VII: PABE2-1; Diabetic animals treated with PABE2 15 mg/kg b.w.
Group VIII: PABE2-2; Diabetic animals treated with PABE2 30 mg/kg b.w.
Group IX: PABE3-1; Diabetic animals treated with PABE3 15 mg/kg b.w.
Group X: PABE3-2; Diabetic animals treated with PABE3 30 mg/kg b.w.
Group XI: STD drug; Diabetic animals treated with 600 µg/kg b.w. (Ananda Prabu et al., Citation2012) of glibenclamide.
Plant extracts, glibenclamide and vehicle were administered via an intragastric tube for 30 days.
Oral glucose tolerance test
Oral glucose tolerance test (OGTT) was performed in overnight fasted normal and drug treated rats on the 15th day of treatment. A glucose load (2 g/kg) was given to each rat orally with a feeding syringe exactly after 30 min post administration of extract, standard drug or vehicle. The blood glucose profile of each rat was determined at time 0 min (prior to the glucose load) and 30, 60 and 120 min of post-glucose administration. Food was withheld 18 h prior to administration of glucose during the course of experimentation (Gandhi et al., Citation2012).
Biochemical analysis
Fasting blood glucose was measured on days 0, 14, 21 and 28 by using a glucometer (Bayer’s blood glucose measuring kit with counter strips). On the 30th day, blood was collected in EDTA coated tubes for measuring glycosylated hemoglobin (HbA1C) by using a commercial kit (Coral Clinical Systems, Goa, India) and blood was collected in tubes without anticoagulant for serum preparations. Serum was analyzed for ALT (alanine transaminase), AST (aspartate transaminase), ALP (alkaline phosphatase) by cogent Serum-ALT, AST, ALP estimation kits (Span Diagnostics Limited, Surat, India); triglycerides, total cholesterol (TC), HDL-C was estimated by their respective kits (Span Diagnostics Limited, Surat, India). For antioxidant enzyme assays, a portion of the liver tissue was dissected out, washed with ice-cold saline immediately, and dried on tissue paper. The tissue homogenate was prepared in ice cold phosphate buffer saline (PBS) (0.1 M, pH 7.4) and centrifuged at 10 000 g at 4 °C for 20 min. The supernatant was quantified for the antioxidant enzyme parameters. TBARS (Slater & Sawyer, Citation1971), catalase (Aebi, Citation1994), superoxide dismutase (Misra & Fridovich, Citation1972) and reduced glutathione (Moron et al., Citation1979) were estimated.
Histopathology of pancreas
The pancreas from normal control, diabetic control and maximum drug dose treated animals were blotted free of mucus. The tissues were washed in normal saline, cut in to the desired size and fixed in 10% formalin for 24 h. After fixation, tissues were dehydrated and embedded in paraffin. Sections of tissue were made in microtome of 5 µm in thickness and mounted on slides. The mounted slides were stained with hematoxylin and eosin for photographic observations.
Statistical analysis
The results were expressed as mean ± standard error of the mean (SEM). Statistical analysis of all the data was evaluated according to one-way analysis of variance (ANOVA) using statistical software Graphpad prism version 6. The significance of difference was evaluated using one way ANOVA followed by Dunnett’s multiple comparison test. Probability values of aaaap < 0.0001, aaap < 0.001, aap < 0.01, ap < 0.05 were compared with normal control. Probability values of bbbbp < 0.0001, bbbp < 0.001, bbp < 0.01, bp < 0.05 were compared with disease control.
Results
Molecular modelling studies
To investigate the interaction of β-sitosterol, molecular docking simulations of the binding of β-sitosterol along with acarbose at the HPA active site was carried out using Autodock 4.2. From this study we found that acarbose forms three H-bond interactions with the active site residues Tyr2, Thr6 and Asn5 (), while the hydroxyl oxygen of β-sitosterol has one hydrogen bond with hydroxyl hydrogen of Thr6 and hydroxyl hydrogen of β-sitosterol has a hydrogen bond with backbone carbonyl oxygen of Thr6 ( and ). The estimated free energy binding of β-sitosterol was found to be −8.39 kcal mol−1 with an estimated inhibition constant (Ki) of 269.35 nmol whereas the estimated free energy binding of acarbose was −6.07 kcal mol−1 with an estimated inhibition constant (Ki) of 28.52 µmol which infers clearly that β-sitosterol is more potent than acarbose.
Figure 1. Comparison of binding mode of β-sitosterol in the active site pocket of human pancreatic α-amylase.
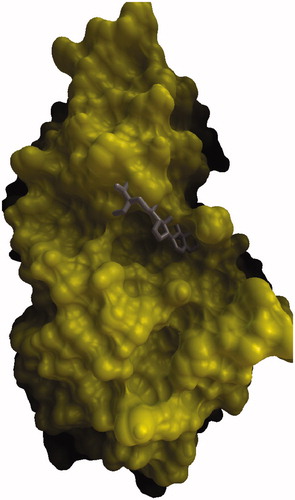
In vitro α-amylase inhibition study
The results of α-amylase inhibition studies revealed that the ethyl acetate fraction (PABEF) possessed a higher enzyme inhibition potential (69.94%) compared to all other fractions, followed by the 50% ethanolic extract (PABEE), which showed marked enzyme inhibition potential (26.51%). PABHF (−69.02%) and PABCF (−12.75%) did not show positive percentage of inhibition. Hence, for further studies, the ethyl acetate fraction (PABEF) was selected.
Acute oral toxicity
Acute oral toxicity studies revealed the nontoxic nature of bark of P. acerifolium at the dose levels tested. No lethality or toxic reactions were observed throughout the study. Mortality was not recorded during 14 days on drug treated animals. Hence, the doses were fixed as 200 and 400 mg/kg for PABEF; 15 and 30 mg/kg for subfractions (PABE1, PABE2 and PABE3).
Effect of P. acerifolium on OGTT
In the oral glucose tolerance test, the blood glucose levels of glucose treated experimental animals were increased markedly at 30 min. PABE3 (15 and 30 mg/kg) inhibited the increasing blood sugar level significantly (p < 0.05) at the 60th and 120th min when compared with disease control ().
Table 1. Effect of ethyl acetate fraction and subfractions of Pterospermum acerifolium bark on changes in blood glucose level during OGTT.
Effect of P. acerifolium on fasting blood glucose levels, body weight and HbA1c
Fasting blood glucose levels were measured in all experimental rats at time zero and on the 14th, 21st and 28th day. PABE3 (30 mg/kg) showed a significant reduction (p < 0.01) in blood glucose levels on the 14th, 21st and 28th day as compared to the disease control group (). The body weight of the diabetic control animals was reduced markedly compared to that of normal animals, PABE3 (30 mg/kg) showed a noticeable increase in body weight compared to diabetic control animals (). The levels of glycosylated hemoglobin (HbA1c) of diabetic control animals were increased significantly (p < 0.0001) compared to that of normal animals, PABE3 (30 mg/kg) decreased the level of HbA1c significantly (p < 0.01) compared to diabetic control ().
Table 2. Effect of ethyl acetate fraction and subfractions of Pterospermum acerifolium bark on changes in blood glucose level.
Table 3. Effect of ethyl acetate fraction and subfractions of Pterospermum acerifolium bark on body weight changes and glycosylated Hb.
Effect of P. acerifolium on levels of serum liver enzymes and lipid parameters
The levels of ALT, AST and ALP were found to be increased significantly in diabetic control animals (p < 0.0001) as compared to that of normal animals. Diabetic animals treated with PABE3 (30 mg/kg) showed the significant reduction (p < 0.01) on levels of ALT, AST and ALP compared to the diabetic control (). In lipid parameters, the levels of triglycerides and total cholesterol of diabetic control animals were increased significantly (p < 0.0001) compared to that of normal vehicle treated animals and the level of HDL-C (p < 0.0001) on the same animals were decreased significantly compared to that of normal vehicle treated animals. Treatment with PABE3 (30 mg/kg) decreased the level of triglycerides (p < 0.001) and total cholesterol (p < 0.01) and increased the level of HDL-C (p < 0.01) significantly when compared to that of normal control ().
Table 4. Effect of ethyl acetate fraction and subfractions of Pterospermum acerifolium bark on levels of serum liver enzymes.
Table 5. Effect of ethyl acetate fraction and subfractions of Pterospermum acerifolium bark on levels of lipid parameters.
Effect of P. acerifolium on in vivo antioxidant enzymes status
The level of thiobarbituric acid reactive substances (TBARS) were found to increase significantly (p < 0.0001) in diabetic control animals compared to that of normal vehicle treated animals and the levels of antioxidant enzymes such as catalase, SOD and GSH were found to decrease significantly (p < 0.0001) in diabetic control animals compared to that of the normal group. Diabetic animals treated with PABE3 (30 mg/kg) showed a significant reduction (p < 0.01) of the levels of TBARS compared to the diabetic control () and the same increased the levels of catalase (p < 0.05), SOD (p < 0.01) and GSH (p < 0.01) significantly compared to the disease control ().
Table 6. Effect of ethyl acetate fraction and subfractions of Pterospermum acerifolium bark on levels of antioxidant status.
Histopathological studies
In diabetic control rats, the microscopic section of pancreas showed the features of insulitis and marked evidence of degeneration of β-cells revealing cellular swelling and cytolysis with picnotic and fragmented nuclei. As compared to the diabetic control, animals treated with the ethyl acetate fraction and subfractions of Pterospermum acerifolium showed lesser features of insulitis, amplified islet cells granulation and proper islet cells arrangement along with its density ().
Figure 4. Histopathological observation of experimental rats pancreas after 30 days of treatment (A) normal control; (B) diabetic control; (C) diabetic animals treated with PABEF (400 mg/kg); (D) diabetic animals treated with PABE1 (30 mg/kg); (E) diabetic animals treated with PABE2 (30 mg/kg); (F) diabetic animals treated with PABE3 (30 mg/kg); (G) diabetic animals treated with glibenclamide (600 µg/kg).
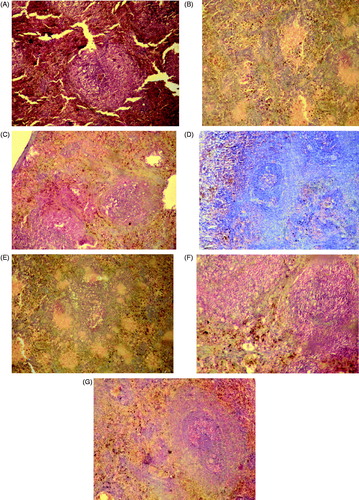
Discussion
Diabetic patients, particularly those with type II diabetes, are at a considerable risk of excessive morbidity and mortality from cardiovascular, cerebrovascular and peripheral vascular diseases leading to myocardial infarction, strokes and amputations (Watkins, Citation2003). Pterospermum acerifolium bark was traditionally used for the treatment of diabetes mellitus, but there was no in-depth proper scientific justification. Hence, the in silico antidiabetic activity of β-sitosterol was determined which was isolated and reported as a major phytoconstituent earlier from the bark of Pterospermum acerifolium (Muhit et al., Citation2010). The molecular modeling results undoubtedly revealed β-sitosterol as a potential inhibitor of HPA in comparison with acarbose. One of the most important therapeutic approaches to treat diabetes is to target the carbohydrate hydrolyzing enzymes, which are responsible for postprandial hyperglycemia (PPHG). α-Amylase is one of those most important enzymes which is responsible for breakdown of carbohydrates and inhibitors of these enzyme delay carbohydrate digestion and prolong overall carbohydrate digestion time, causing a reduction in the rate of glucose absorption and consequently blunting the postprandial plasma glucose rise (Ali et al., Citation2006). In the present study, 50% ethanol extract (PABEE) and fractions (PABHF, PABCF and PABEF) were studied for its α-amylase inhibition potential and found PABEF (ethyl acetate fraction) as active against α-amylase inhibition. Hence, PABEF was selected for further subfractionation and in vivo studies.
Postprandial hyperglycemia contributes to microvascular and macrovascular complications during diabetic conditions and effective control of postprandial hyperglycemia plays a vital role in early intervention and prevention of diabetic complications (Gandhi et al., Citation2012). In the present study, PABE3 (30 mg/kg) treated diabetic rats significantly reduced the blood glucose levels at 60th and 120th min when compared to that of diabetic control and it implies that there is further scope of the drug to be studied in detail on animal models of diabetic complications.
Administration of STZ leads to necrosis of the β-cells through the formation of alkylating free radicals. The rate of insulin synthesis was diminished and the administration of nicotinamide, a poly-ADP-ribose synthetase inhibitor, protected the function of the islets by preventing the decrease in the levels of NAD and proinsulin. This phenomenon partially reversed the inhibition of insulin secretion (Srinivasan & Pari, Citation2012). Persistent hyperglycemia, the common characteristic of diabetes, can cause most diabetic complications and in all patients. Treatment should therefore aim to lower blood glucose to near-normal levels (Shirwaikar et al., Citation2005). In the present study, PABE3 (15 and 30 mg/kg) reduced the blood glucose level at 14th, 21st and 28th days significantly and it showed clearly its antihyperglycemic effect which may be due to its increased release of insulin from existing β-cells. STZ-nicotinamide induced diabetes is characterized by the severe loss of body weight due to the destruction of structural proteins, which are known to contribute to body weight (Ananda Prabu et al., Citation2012). Treatment of diabetic animals with PABE3 (30 mg/kg) prohibited the loss of body weight when compared to that of diabetic control animals and showed its ability to reduce hyperglycaemia. HbA1C is a nonenzymatic glycated product of the hemoglobin β-chain and it is normally present at low levels in circulating red cells because of the glycosylation reaction between Hb and circulating glucose, but in the presence of excess plasma glucose this glycation is increased, thus making the HbA1C a useful index of glycemic control (Yavari, 2011). Diabetic animals treated with PABE3 (15 and 30 mg/kg) reduced the HbA1c levels significantly compared to that of diabetic control group and it could also be due to its anti-hyperglycemic property.
AST, ALT and ALP are the biomarker enzymes needed to evaluate hepatic disorders. Increase in the activities of these enzymes in diabetic rats validated hepatic damage (Navarro et al., Citation1993). Significant reductions in the activities of these enzymes in PABE3 (30 mg/kg) treated diabetic animals indicated the hepatoprotective role in preventing diabetic complications. The elevation of serum triglycerides, total cholesterol and the decline in the level of HDL-C was well documented in diabetic animals (Shirwaikar et al., Citation2005). Significant reduction in the level of triglycerides, total cholesterol and rise in the level of HDL-C was observed in PABE3 (30 mg/kg) treated diabetic animals and it may be directly attributed to improvements in insulin levels upon the treatment or indirectly to its influence on various lipid regulation systems (Shirwaikar et al., Citation2005).
A marked increase in the level of TBARS and decrease in the levels of antioxidant enzymes such as catalase, GSH and SOD were observed in diabetic animals. The increased levels of TBARS in diabetic animals were due to activation of the lipid peroxidation system in tissues and may lead to cellular infiltration and cell damage (Srinivasan & Pari, Citation2012). Superoxide dismutase is an important defense enzyme, which catalyzes the dismutation of superoxide radicals (McCord et al., Citation1976), and catalase is a hemoprotein, which catalyzes the reduction of hydrogen peroxides and protects tissues from highly reactive hydroxyl radicals (Chance et al., Citation1952). GSH is one of the essential compounds for maintaining cell integrity against ROS, as it can scavenge free radicals and reduce hydrogen peroxide and the exhaustion of GSH promotes oxidative stress with a cascade of effects on the functional and structural integrity of cells and organelle membranes (Masella et al., Citation2004). The liver plays a major role in glutathione homeostasis and is the main export organ for glutathione (Townsend et al., Citation2003). Treatment with PABE3 (30 mg/kg) reduced the TBARS levels and increased the levels of antioxidant enzymes compared to that of diabetic control and it clearly implied its role against oxidative stress. Histopathological changes showed the prominent changes in islet cell arrangement and density in Pterospermum acerifolium treated animals, which also evidenced its diabetic activity. The active subfraction PABE3 was subjected to chromatographic studies in the reported chromatographic conditions and solvent system to examine the presence of β-sitosterol (Misar et al., Citation2010). We have found two major components, but their Rf values did not match with the reported β-sitosterol Rf value. Hence, there was no correlation between β-sitosterol and in vivo antidiabetic activity shown by PABE3, but by our inference we confirm that some antidiabetic phytoconstituents other than β-sitosterol were present in that active fraction which was responsible for the activity.
Conclusion
From the present study it can be concluded that PABEF showed maximum α-amylase inhibition potential and its subfraction PABE3 showed maximum efficacy in reducing hyperglycemia. The present study demonstrated for the first time the alpha-amylase inhibitory potential and elaborated antidiabetic activity of the bark of Pterospermum acerifolium. Further studies to isolate the bioactive constituents responsible for the antidiabetic activity and detailed cellular assays to estimate the mechanism of action are in progress on the active subfraction PABE3. The above study also reveals the scope of the bark of Pterospermum acerifolium in the screening of diabetic complications.
Declaration of interest
The authors report no declarations of interest. The authors alone are responsible for the content and writing of the paper. This work was financially supported by University Grant Commission (UGC) under the scheme of major research project (MRP), New Delhi, India. (Grant No: F.39-157/2010 (SR))
Acknowledgements
The authors would like to acknowledge the Department of Pharmaceutical Sciences Birla Institute of Technology, Mesra, Ranchi, India for providing the necessary facilities to carry out the study and the University Grants Commission (UGC) for providing financial assistance. The authors are thankful to Dr. K.K. Singh, Chairman-Veterinary Pathology division, Birsa Agricultural University, Ranchi for assistance in histopathology observations.
References
- Aebi H. (1994). Catalase in vivo methods. Enzymology 105:121–6
- Ali H, Houghton PJ, Soumyanath A. (2006). α-Amylase inhibitory activity of some Malaysian plants used to treat diabetes; with particular reference to Phyllanthus amarus. J Ethnopharmacol 107:449–55
- Ananda Prabu K, Kumarappan CT, Christudas S, Kalaichelvan VK. (2012). Effect of Biophytum sensitivum on streptozotocin and nicotinamide induced diabetic rats. Asian Pacific J Trop Biomed 2:31–5
- Aylin S, Sereften A, Cemal C, et al. (2007). Effects of in vivo antioxidant enzyme activities of myrtle oil in normoglycaemic and alloxan diabetic rabbits. J Ethnopharmacol 110:498–503
- Chance B, Greenstein DS, Roughton RJW. (1952). The mechanism of catalase action steady state analysis. Arch Biochem Biophys 37:301–39
- Cheng D. (2005). Prevalence, predisposition and prevention of type II diabetes. Nutr Metab 18:2–29
- Chopra RN, Nayar SL, Chopra IC. (1956). Glossary of Indian Medicinal Plants. Council of Scientific & Industrial Research, New Delhi, India. p. 116
- Dixit P, Khan MP, Swarnkar G, et al. (2011). Osteogenic constituents from Pterospermum acerifolium Willd. flowers. Bioorg Med Chem Lett 21:4617–21
- Gandhi GR, Ignachimuthu S, Paulraj MG. (2012). Hypoglycemic and β-cells regenerative effects of Aegle marmelos (L.) Corr. bark extract in streptozotocin-induced diabetic rats. Food Chem Toxicol 50:1667–74
- Gupta R, Sharma AK, Dobhal MP, et al. (2011). Antidiabetic effect and antioxidant potential of β-sitosterol in streptozotocin-induced experimental hyperglycemia. J Diabetes 3:29–37
- King H, Aubert RE, Herman WH. (1998). Global burden of diabetes, 1995–2025: Prevalence, numerical estimates, and projections. Diabetes Care 21:1414–31
- Kirtikar KR, Basu BD. (1935). Indian medical plants. J Pharm Res 2:785–8
- Masella R, Vari R, D’Archivio M, et al. (2004). Extra virgin olive oil biophenols inhibit cell mediated oxidation of LDL by increasing the mRNA transcription of glutathione-related enzymes. J Nutr 134:785–91
- Mazumder PM, Sasmal D, Ghosh AR, Paramaguru R. (2011). Evaluation of antihyperlipidemic and antioxidant activity of Pterospermum acerifolium (L.) Willd. Pharmacol Online 3:128–46
- McCord JM, Keele BB, Fridovich I. (1976). An enzyme based theory of obligate anaerobiosis, the physiological functions of superoxide dismutase. Proc Natl Acad Sci USA 68:1024–7
- Misar A, Mujumdar AM, Ruikar A, Deshpande NR. (2010). Quantification of β-sitosterol from barks of three Acacia species by HPTLC. J Pharm Res 3:2595–6
- Misra HP, Fridovich I. (1972). The role of superoxide anion in the auto-oxidation of ephinephrine and a simple assay for superoxide dismutase. J Biol Chem 247:3170–5
- Moron ΜS, Depierre JW, Mannervik Β. (1979). Levels of glutathione, glutathione reductase, glutathione-S-transferase activities in rat lung and liver. Biochim Biophys Acta 582:67–78
- Muhit Md A, Khanam SS, Islam Md S, et al. (2010). Phytochemical and biological investigations of Pterospiermum acerifolium Willd bark. J Pharm Res 3:2643–6
- Muller D. (2001). New drug targets for type 2 diabetes and the metabolic syndrome. Nature 414:821–7
- Navarro CM, Montilla PM, Martin A, et al. (1993). Free radicals scavenger and antihepatotoxic activity of Rosmarinus tomentosus. Planta Med 59:312–14
- Pellegrino M, Broca C, Gross R, et al. (1998). Development of a new model of type 2 diabetes in adult rats administered with streptozotocin and nicotinamide. Diabetes 47:224–9
- Shirwaikar A, Rajendran K, Punitha ISR. (2005). Antidiabetic activity of alcoholic stem extract of Coscinium fenestratum in streptozotocin-nicotinamide induced type 2 diabetic rats. J Ethnopharmacol 97:36974
- Slater TF, Sawyer BC. (1971). The stimulatory effect of carbontetrachloride and other halogen alkane or peroxidative reaction in the rat liver function in-vitro. Biochem J 123:805--15
- Srinivasan K, Ramarao P. (2007). Animal models in type 2 diabetes research: An overview. Indian J Med Res 125:451–72
- Srinivasan S, Pari L. (2012). Ameliorative effect of diosmin, a citrus flavonoid against streptozotocin nicotinamide generated oxidative stress induced diabetic rats. Chem Biol Interact 195:43–51
- Townsend DM, Tew KD, Tapiero H. (2003). The importance of glutathione in human disease. Biomed Pharmacother 57:145–55
- Watkins PJ. (2003). ABC of diabetes, cardiovascular disease, hypertension and lipids. Brit Med J 10:82–4
- WHO Expert Committee on Diabetes mellitus (1980). Technical Report Series 646, Second Report. Geneva: World Health Organization
- Yavari A. (2011). Glycosylated hemoglobin: The importance in management of type 2 diabetes. J Stress Physiol Biochem 7:122–9