Abstract
Context: Acetaminophen (APAP) leads to severe hepatic and renal necrosis and thus causes significant clinical problems. Artemisia pallens Walls ex D.C. (Asteraceae) possesses various pharmacological properties such as antidiabetic, antioxidant, analgesic, and anti-inflammatory activity.
Objective: The objective was to evaluate the protective effects of Artemisia pallens methanol extract (APME) in APAP-induced hepatic and nephro-toxicity.
Materials and methods: The methanolic extract of aerial parts of Artemisia pallens (APME) was prepared. Toxicity was induced in male Wistar rats (180–220 g) by administration of APAP (700 mg/kg, p.o., 14 d). APME (100, 200, and 400 mg/kg, p.o.) was administered to rats 2 h before APAP oral administration. Various biochemical and molecular parameters along with histopathological aberration were studied in the kidney and liver of rats.
Results: Pretreatment with APME (200 and 400 mg/kg, p.o.) significantly (p < 0.01 and p < 0.001) decreased aspartate transaminase (AST), alanine transaminase (ALT), bilirubin, blood urea nitrogen (BUN), and serum creatinine as compared with APAP-treated rat. Decreased level of serum albumin, serum uric acid, and HDL were significantly (p < 0.01 and p < 0.001) restored by APME (200 and 400 mg/kg, p.o.) pre-treatment. Administration of APME (200 and 400 mg/kg, p.o.) significantly (p < 0.01 and p < 0.001) reduced the elevated level of cholesterol, LDL, LDH, triglyceride, and VLDL. It also significantly (p < 0.01 and p < 0.001) restored the altered level of hepatic and renal antioxidant enzymes (superoxide dismutase (SOD) and glutathione (GSH)). The increased level of malondialdehyde (MDA) and nitric oxide (NO) in hepatic as well as renal tissue was significantly (p < 0.01 and p < 0.001) decreased by APME (200 and 400 mg/kg, p.o.) administration. Histological alternation induced by APAP in liver and kidney was also reduced by the APME (200 and 400 mg/kg, p.o.) pre-treatment.
Conclusion: It is concluded that the methanol extract of Artemisia pallens alleviates APAP induced in rats toxicity through its antioxidative and anti-inflammatory actions.
Introduction
Acetaminophen (APAP), i.e., paracetamol, has been the preferred medication for the treatment of pain especially in children suggesting that it is safer and perhaps more efficacious than aspirin (Ahmad et al., Citation2012). APAP exerts its analgesic potential via the formation of its conjugated metabolite, i.e., p-aminophenol with arachidonic acid by fatty acid amide hydrolase to form archidonic acid metabolite which exerts its effect through cannabinoid receptors (Anderson et al., Citation1999). APAP does not produce any gastrointestinal complication or affects blood coagulation or impair renal functions within the recommended doses. However, in cancer patients who receive chemotherapy anticancer drugs are usually treated with higher doses of APAP to relieve pain (Nassar et al., Citation2009). Therefore, APAP toxicity at higher doses is very common and is often associated with hepatic (Nelson, Citation1990) as well as renal damage (Ghosh & Sil, Citation2007) in clinical settings.
Moreover, APAP-induced toxicity is a well-established animal model to screen various hepatoprotective agents (Kandhare et al., Citation2011b). It has been reported that administration APAP results in hepatic and renal damage in humans as well as in experimental animals, although nephrotoxicity is less common than hepatotoxicity (Larson et al., Citation2005). The hepatic and renal damage by the administration of APAP has complex events. Primarily APAP is converted into water-soluble metabolites in the liver via glucuronidation as well as sulfuration reactions and the metabolites are excreted via the kidney. The microsomal CYP-450 enzyme system (cytochrome P450 (CYP) 2E1 and CYP 3A4) plays a vital role in the metabolism of APAP. During the biotransformation of APAP, a highly reactive intermediate N-acetyl-p-benzoquinoneimine (NAPQI) is produced (Mitchell et al., Citation1973). NAPQI reacts with intracellular glutathione (GSH) and causes depletion of GSH. Moreover, NAPQI also binds to cellular proteins and initiates lipid peroxidation, leading to renal (Hart et al., Citation1994) and hepatic injury (Mitchell et al., Citation1977). In a recent study, involvement of various mechanisms besides GSH depletion by NAPQI in hepatic and renal tissue by APAP has been reported. The formation of reactive oxygen species (ROS), nitrogen species, and macrophages have been identified as being involved in the development of APAP toxicity (Michael et al., Citation1999).
Pharmacotherapy for the management of APAP induced toxicity is multivariate. Aminoguanidine, zinc sulfate, lobenzarit, and N-acetylcysteine are treatment options for APAP-induced toxicity (Michael et al., Citation1999; Prescott, Citation1979). However, these synthetic moieties are associated with severe undesirable adverse effects which limit their use. Nowadays, orthotopic liver transplantation (OLT) is a therapeutic option for liver failure caused by APAP (Starzl et al., Citation1987). OLT also depends on defined prognostic factors for the effective management of APAP toxicity. Therefore, a search for novel agents such as plant-derived products and their bioactive compound has been carried out for the management of APAP toxicity.
In Ayurveda, the Indian system of medicine, a number of medicinal plants have been recommended as a treatment option for liver and kidney diseases (Subramoniam & Pushpangadan, Citation1999). At present, for the treatment of liver cirrhosis and alcoholic liver diseases, extensively used plant-derived phytoconstituent is silymarin which is a polyphenolic flavanoid isolated from the fruits and seeds of Silybum marianum (L.) Gaertn. (Asteraceae) which acts via its antioxidant potential by inhibiting lipid peroxidation (Valenzuela & Garrido, Citation1993). Hence, in the present investigation, silymarin was used as a standard hepatoprotective drug (positive control).
Artemisia pallens Walls ex D.C. (Davana) (Asteraceae) is an aromatic medicinal herb native in the southern part of India, especially in the states of Karnataka, Tamil Nadu, Andhra Pradesh, and Maharashtra. A number of researchers have reported chemical composition of oil obtained from A. pallens plant (Pujar et al., Citation2000; Rojatkar et al., Citation1996; Sipma & Van der Wal, Citation1968). The oil of A. pallens is used as a flavoring agent for cakes, pastries, tobacco, and in some costly beverages. Reported pharmacological properties of the plant are anthelmintic, tonic, antipyretic, antidiabetic, antifungal, antibacterial, antimicrobial, antioxidant, analgesic, and anti-inflammatory activity (Ashok & Upadhaya, Citation2010; Ruikar et al., Citation2011). However, the effectiveness of Artemisia pallens in APAP-induced toxicity has not been yet reported. The objective of the present investigation was to evaluate the protective effect of a methanol extract of Artemisia pallens in APAP-induced toxicity by studying various biochemical and molecular parameters along with histopathological aberration in the kidney and liver of rats.
Materials and methods
Animals
Adult male Wistar rats (180–220 g) were obtained from the National Institute of Biosciences, Pune, India. They were housed in cages in a facility maintained at 24 ± 1 °C, with a relative humidity of 45–55% and 12:12 h dark/light cycle. The animals had free access to standard pellet chow (Pranav Agro Industries Ltd., Sangli, India) and filtered water throughout the experimental period. All experiments were carried out between 09:00 and 17:00 h. The experimental protocol was approved by the Institutional Animal Ethics Committee (IAEC) of Poona College of Pharmacy, Pune, India, and performed in accordance with the guidelines of Committee for Control and Supervision of Experimentation on Animals (CPCSEA), Government of India. Animals were transferred to testing laboratory 1 h before the experiment for adaptation.
Chemicals
1,1′,3,3′-Tetraethoxypropane, crystalline beef liver catalase, reduced GSH, and 5,5′-dithiobis (2-nitrobenzoic acid) were purchased from S.D. Fine Chemicals, Mumbai, India. Sulphanilamides, naphthalamine diamine HCl, and phosphoric acid were obtained from LobaChemi Pvt. Ltd., Mumbai, India. APAP and silymarin were obtained as gift samples from Symed Pharmaceutical Pvt. Ltd, Hyderabad, India.
Preparation of extract
The arial parts of Artemisia pallens plant were collected from Jejuri, Maharashtra state, India, in the month of December 2011. The plant was authenticated by Dr. Mrs. Upadhey, Department of Botany, Agharkar Research Institute, Pune, India. The voucher specimen of plant material was maintained under the reference number WP-091. The methanol extract was prepared by macerating air-dried and course powdered plant material (500 g) with methanol (5 L) for 24 h at room temperature. The extract was filtered and the filtrate was concentrated in vacuo at 40 °C. This process was repeated three times. The yield of methanol extract was 52.50 g. The aqueous solution of methanol extract of Artemisia pallens in DMSO was prepared for the pharmacological evaluation.
Induction of APAP induced toxicity and drug treatment schedule
The selection of dose for APAP was based on the studies carried out previously (Ahmad et al., Citation2012). Silymarin was administered to rats orally at a dose of 25 mg/kg for 14 d (Hegde & Joshi, Citation2010). APAP (1% aqueous solution of gum acacia) was administered to rats orally at a dose of 700 mg/kg (Ahmad et al., Citation2012). Fasted rats were randomly divided into six groups of six rats as follows:
Group I: Normal control: rats were administered single daily dose of DMSO (10 mg/kg), p.o. for 14 d.
Group II: APAP: rats that served as the model control was administered single daily dose of DMSO (10 mg/kg, p.o.) 2 h before oral administration APAP suspension (700 mg/kg) for 14 d.
Group III: Silymarin (25): rats were administered single daily dose of silymarin (25 mg/kg, p.o.) in distilled water, 2 h before oral administration APAP suspension (700 mg/kg) for 14 d.
Group IV: Artemisia pallens methanol extract (APME) (100): rats were administered single daily dose of APME (100 mg/kg, p.o.) 2 h before oral administration APAP suspension (700 mg/kg) for 14 d.
Group V: APME (200): rats were administered single daily dose of APME (200 mg/kg, p.o.) 2 h before oral administration APAP suspension (700 mg/kg) for 14 d.
Group VI: APME (400): rats were administered single daily dose of APME (400 mg/kg, p.o.) 2 h before oral administration APAP suspension (700 mg/kg) for 14 d.
Serum biochemistry
The serum was separated by centrifugation using an Eppendorf cryocentrifuge (model no. 5810, Eppendorf, Hamburg, Germany), maintained at 4 °C and run at a speed of 7000 rpm for 15 min. The levels of serum albumin, alkaline phosphatase (ALP), blood urea nitrogen (BUN), cholesterol, creatinin, direct bilirubin, high-density lipoprotein (HDL), lactate dehydrogenase (LDH), low-density lipoprotein (LDL), aspartate transaminase (AST), alanine transaminase (ALT), triglyceride, total bilirubin, and uric acid were measured by a spectrophotometer (UV–visible spectrophotometer, Jasco V-530, Tokyo, Japan) using commercially available reagent kits according to procedure provided by manufacturer (Accurex Biomedical Pvt. Ltd., Mumbai, India).
The biochemical estimations
Preparation of tissue homogenate
For liver and kidney homogenization, tissue segments were mixed with 0.1 M phosphate buffer and homogenized on ice for 60 s at 10 000 rpm in a homogenizer (Remi Equipment Pvt. Ltd., Remi Motors Ltd., Mumbai, India). Supernatant of tissue homogenates was employed to estimate superoxide dismutase (SOD), reduced GSH, lipid peroxidation (malondialdehyde (MDA) content), and nitric oxide (NO content).
Determination of total protein, SOD, GSH, MDA, and NO
The level of total protein, SOD, GSH, MDA, and NO in liver and kidney homogenate was determined according to earlier reported methods (Gosavi et al., Citation2012; Kandhare et al., Citation2011a, Citation2013a–c).
Histopathological examination
Liver and kidney tissues were stored in 10% formalin for 24 h. The specimen was dehydrated and placed in xylene for 1 h (three times) and later in ethyl alcohol (70, 90, and 100%) for 2 h. The infiltration and impregnation were carried out by treating with paraffin wax twice, each time for 1 h. Tissue specimens were cut into sections of 3–5 µm thickness and were stained with hematoxylin and eosin (H&E). The specimen was mounted on slide by use of distrene pthalate xylene (DPX) as a mounting medium. Sections were examined under a light microscope for the inspection of the histopathology features of specimen and infiltration of cells. The various changes in histological features were graded as Grade 0 (not present or very slight), Grade 1 (mild), Grade 2 (moderate), and Grade 3 (severe) as described earlier (Yamasaki et al., Citation2001).
Statistical analysis
Data were expressed as mean ± standard error mean (SEM). Data analysis was performed using software (v 5.0, Graph Pad, San Diego, CA). Data of biochemical parameters were analyzed using a one-way analysis of variance (ANOVA) and Dunnett's test was applied for post hoc analysis. A value of p < 0.05 was considered to be statistically significant.
Results
Effect of treatment of APME on APAP-induced alteration in body weight and relative organ weight in rats
There was a significant (p < 0.001) decrease in the body weight of APAP rats as compared with normal rats, whereas relative organ weights of liver and spleen were significantly (p < 0.001) increased in APAP rats as compared with normal rats. Treatment with APME (200 and 400 mg/kg, p.o.) did not show any significant change in the body weight as compared with APAP rats but, relative liver weight as well as relative spleen weight were significantly (p < 0.001) decreased as compared with APAP rats. Administration of silymarin (25 mg/kg, p.o.) for 14 d significantly (p < 0.01) increased the body weight as compared with APAP rats whereas relative liver weight and spleen weight were significantly (p < 0.001) decreased as compared with APAP rats ().
Table 1. Effect of treatment of APME on body weight, liver weight, and spleen weight in paracetamol-induced toxicity in rats.
Effect of treatment of APME on APAP-induced alteration in serum albumin, BUN, serum creatinine and serum uric acid in rats
The levels of serum albumin and serum uric acid were significantly (p < 0.01 and p < 0.001) decreased in APAP rats as compared with normal rats whereas BUN and serum creatinine levels were significantly (p < 0.001) increased in the APAP rats as compared with normal rats. Chronic administration of APME (100, 200, and 400 mg/kg, p.o.) for 14 d significantly increased serum albumin (p < 0.01, p < 0.05, and p < 0.01, respectively) and serum uric acid (p < 0.01, p < 0.01, and p < 0.001, respectively) levels whereas the levels of BUN and serum creatinine were significantly (p < 0.01 and p < 0.001) decreased by the treatment of APME (400 mg/kg, p.o.) as compared with APAP rats. When compared with APAP rats, the serum uric acid levels were significantly (p < 0.001) increased in silymarin (25 mg/kg, p.o.) treated rats whereas the levels of BUN and serum creatinine were significantly (p < 0.01 and p < 0.001) decreased. However, silymarin (25 mg/kg, p.o.) failed to produce any significant change in serum albumin level as compared with serum albumin level of APAP rats ().
Figure 1. Effect of treatment of APME on albumin (A), blood urea nitrogen (B), serum creatinine (C), and uric acid (D) in paracetamol-induced toxicity in rats. Data are expressed as mean ± SEM (n = 6) and analyzed by one-way ANOVA followed by Dunnett's test for each parameter separately. *p < 0.05, **p < 0.01, and ***p < 0.001 as compared with the APAP group and ##p < 0.01 and ###p < 0.001 as compared with the normal group.
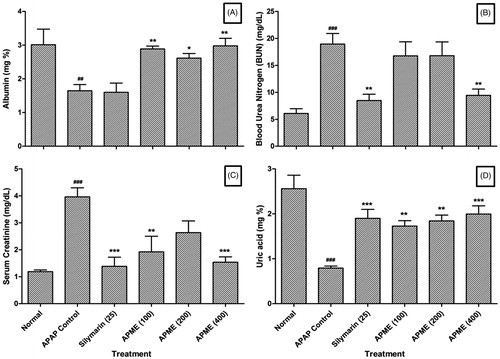
Effect of treatment of APME on APAP-induced alteration in ALP, total bilirubin, direct bilirubin, AST, and ALT in rats
Chronic administration of APAP caused a significant (p < 0.001) increase in the level of ALP, total bilirubin, direct bilirubin, AST, and ALT in APAP rats as compared with normal rats. When compared with APAP rats, there was a significant (p < 0.01 and p < 0.001) and dose-dependant decrease in the level of total bilirubin, direct bilirubin after chronic treatment of APME (200 and 400 mg/kg, p.o). However, administration of APME (200 and 400 mg/kg, p.o.) significantly (p < 0.001) decreased elevated levels of AST and ALT as compared with APAP rats. Administration of APME (100, 200, and 400 mg/kg, p.o.) failed to produce any significant reduction in the elevated level of ALP as compared with APAP rats. Silymarin (25 mg/kg, p.o.) administration for 14 d significantly reduced these elevated levels of ALP (p < 0.05), total bilirubin (p < 0.001), direct bilirubin (p < 0.05), AST (p < 0.001), and ALT (p < 0.001) when compared with APAP rats ().
Table 2. Effect of treatment of APME on alkaline phosphatase, total bilirubin, direct bilirubin, SGOT, and SGPT in paracetamol-induced toxicity in rats.
Effect of treatment of APME on APAP-induced alteration in serum cholesterol, HDL, LDL, LDH, triglyceride, and VLDL in rats
There were significant (p < 0.001) increases in the levels of serum cholesterol, LDL, LDH, triglyceride, and VLDL in APAP rats as compared with normal rats. Chronic administration of APAP for 14 d significantly (p < 0.001) decreased the level of HDL in APAP rats as compared with normal rats. The level of serum HDL was significantly (p < 0.05, p < 0.01, and p < 0.001) and dose dependently increased by the chronic treatment with APME (100, 200, and 400 mg/kg, p.o.) when compared with APAP rats. Moreover, administration of APME (200 and 400 mg/kg, p.o.) for 14 d significantly and dose dependently prevented APAP caused increase in the level of LDH (p < 0.01, p < 0.001 and p < 0.001), triglyceride (p < 0.01 and p < 0.001), and VLDL (p < 0.01 and p < 0.001) as compared with APAP rats. The increased levels of serum cholesterol and LDL after APAP administration were significantly (p < 0.01) decreased by chronic administration of APME (400 mg/kg, p.o., 14 d) as compared with APAP rats. Silymarin (25 mg/kg, p.o.) significantly (p < 0.001) decreased the elevated levels of serum cholesterol, LDL, triglyceride, and VLDL as compared with APAP rats. However, administration of silymarin (25 mg/kg, p.o.) did not produce any significant change in the level of HDL and LDH as compared with APAP rats ().
Table 3. Effect of treatment of APME on cholesterol, HDL, LDL, LDH, triglyceride, and VLDL in paracetamol-induced toxicity in rats.
Effect of treatment of APME on APAP-induced alteration in hepatic as well as renal SOD and GSH in rats
Chronic administration of APAP for 14 d resulted in a significant (p < 0.001) decrease in the levels of SOD and GSH in hepatic as well as renal tissue in APAP rats as compared with normal rats. Treatment with APME (200 and 400 mg/kg, p.o.) showed a significant elevation in the level of hepatic SOD (p < 0.001) and renal SOD (p < 0.05) as well as hepatic GSH (p < 0.01) and renal GSH (p < 0.001) as compared with APAP rats. However, administration APME (100 mg/kg, p.o.) for 14 d did not show any significant increase in the level of SOD and GSH in both liver and kidney when compared with APAP rats. Silymarin (25 mg/kg, p.o.) showed a significant (p < 0.001) increase in the level of hepatic SOD and renal GSH as compared with APAP rats but, it failed to produce any significant increase in the level of hepatic GSH and renal SOD as compared with APAP rats ().
Figure 2. Effect of treatment of APME on hepatic and renal SOD (A), GSH (B), MDA (C), and NO (D) in paracetamol-induced toxicity in rats. Data are expressed as mean ± SEM (n = 6) and analyzed by a one-way ANOVA followed by Dunnett's test for each parameter separately. *p < 0.05, **p < 0.01, and ***p < 0.001 as compared with the APAP group and ##p < 0.01 and ###p < 0.001 as compared with normal group.
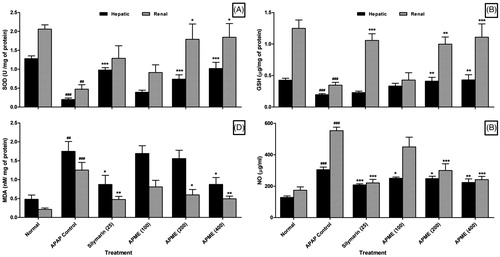
Effect of treatment of APME on APAP-induced alteration in hepatic as well as renal MDA and NO in rats
There was a significant increase in the levels of hepatic MDA (p < 0.01) and NO (p < 0.001) as well as renal MDA (p < 0.001) and NO (p < 0.001) in APAP rats as compared with normal rats. Treatment with APME (400 mg/kg, p.o.) showed a significant decrease in hepatic as well as renal MDA (p < 0.05) and NO (p < 0.01) whereas levels of hepatic NO (p < 0.05) and renal MDA (p < 0.05) as well as renal NO (p < 0.001) were significantly decreased in APME (200 mg/kg, p.o.) treated rats as compared with APAP rats. However, APME (100 mg/kg, p.o.) showed a significant (p < 0.05) attenuation in hepatic NO as compared with APAP rats. Treatment with APME (200 mg/kg, p.o.) did not show any significant decrease in the level of hepatic MDA as compared with APAP rats. When compared with APAP rats, silymarin (25 mg/kg, p.o.) treated rats showed significant decrease in the level of hepatic MDA (p < 0.05) and NO (p < 0.001) as well as renal MDA (p < 0.01) and NO (p < 0.001) ().
Effect of treatment of APME on APAP induced pathological alteration in rat liver and kidney
In the histopathological studies, normal control animals showed normal central vein in liver parenchymal cells and without any signs of inflammation as well as necrosis in cells (). However, there was evidence of congestion (grade 1) and vacuolization (grade 1). The histopathological examination of liver of APAP-administered rats showed inflammatory cells (grade 4) and shrunken hepatocytes with chromatin condensation. Administration of APAP caused hepatocellular injury reflected by the presence of diffuse cytoplasmic vacuolation (grade 3), centrilobular necrosis (grade 4), vascular congestion (grade 3), and nuclear pyknosis (grade 3) of the hepatocytes. Macrovesicular fatty changes (grade 2) were also evident in liver histological observation of APAP rats (). However, silymarin (25 mg/kg) showed moderate histopathological changes in liver marked by sinusoidal congestion (grade 2), cytoplasmic vacuolation (grade 1), and the presence of only few inflammatory cells (grade 1) along with few fatty globules (grade 1) (). In the APME (400 mg/kg) treated rats, the histology of liver showed mild degree of vacuolization (grade 1), necrosis (grade 1), and congestion (grade 1) around central vein. Moderate inflammatory cells (grade 2) were present in APME (400 mg/kg) treated rats () ().
Figure 3. Effect of treatment of APME on paracetamol-induced pathological alteration in rat liver. Photomicrograph of sections of liver of normal (A), APAP (B), silymarin (25 mg/kg) treated (C), and APME (400 mg/kg) treated (D) rats. Inflammatory infiltration (black arrow), edema (yellow arrow), congestion (green arrow), and necrosis (red arrow). H&E staining at 40 × and 100 × (inset).
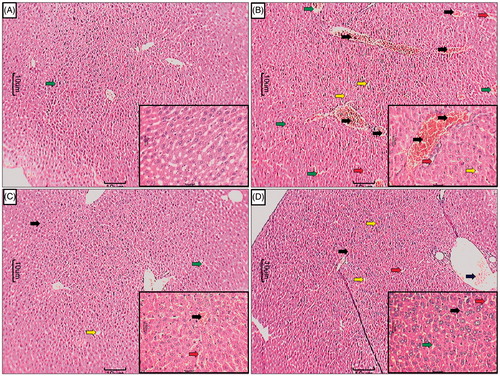
Table 4. Effect of treatment of APME on paracetamol-induced pathological alteration in rat liver.
shows normal architecture of the kidney. Chronic administration of APAP caused renal damage evident by glomerular structural disruption (grade 4) and partial endothelial rupture in capsule. APAP animals showed the presence of intraluminal cell debris, karyorrhexis, and pyknosis (grade 2) indicating cell death (). Treatment with silymarin (25 mg/kg) as well as APME (400 mg/kg) showed a moderate number of inflammatory (grade 2) and apoptotic cells (). APME (400 mg/kg) treated rats showed mild architectural damage with few intraluminal cell debris (grade 1) and infiltration of inflammatory cells (grade 1) as compared with APAP () ().
Figure 4. Effect of treatment of APME on paracetamol-induced pathological alteration in rat kidney. Photomicrograph of sections of kidney of normal (A), APAP (B), silymarin (25 mg/kg) treated (C), and APME (400 mg/kg) treated (D) rats. Glomerular hypertrophy (white arrow), inflammatory infiltration (black arrow), edema (yellow arrow), congestion (green arrow), and necrosis (red arrow). H&E staining at 40 × and 100 × (inset).
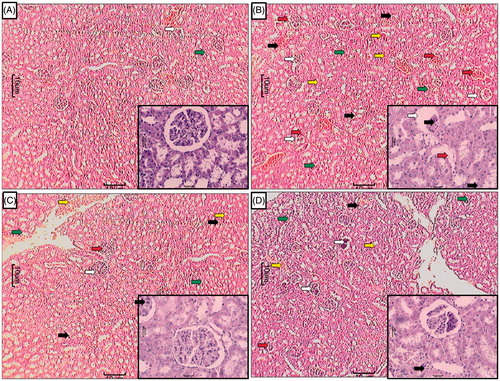
Table 5. Effect of treatment of APME on paracetamol-induced pathological alteration in rat kidney.
Discussion
In recent times, APAP-induced toxicity is a significant clinical problem and this has generated a heated debate (Watkins & Seeff, Citation2006). Hence, understanding of pathophysiological processes behind APAP-induced toxicity played a decisive role in the development of new therapeutic strategies.
Administration of APAP caused significant saturation of glucuronidation and sulfuration conjugation pathway that results in GSH depletion and formation of reactive metabolites. An elevated levels of reactive metabolites caused mitochondrial dysfunction and generation of oxidative stress which leads to hepatotoxicity as well as nephrotoxicity (Ahmad et al., Citation2012).
Various endogenous biomarkers such as ALT and AST serves as a gold standard for hepatotoxicity. ALT is considered as the better index of liver injury, since ALT plays a vital role in the conversion of alanine to pyruvate and glutamate which is released in a similar manner and that represents almost 90% of total enzymes present in the body. Hence, ALT is more specific parameter of hepatotoxicity (Drotman & Lawhorn, Citation1978; Visnagri et al., Citation2012, Citation2013). In the present investigation, elevated levels of ALT and AST in serum are the consequence of APAP-induced liver dysfunction and denote the damage to the hepatic cells. This elevated levels of ALT and AST is accompanied by hepatocellular fatty deposition and centrilobular necrosis which was corroborated with the histopathological findings of liver tissue of APAP rats. Administration of a methanol extract of Artemisia pallens showed a significant reduction in elevated levels of serum ALT and AST and may restore the functional integrity of hepatic cells thus revealing the hepatoprotective nature of APME against APAP hepatotoxicity. APME treatment also reduced the hepatocellular fatty deposition as well as necrosis revealed by the histopathological examination of APME treated liver tissue.
In APAP-induced hepatotoxicity, bile canaliculi cell lining caused release of ALP in response to cholestasis as well as increased biliary pressure (Gaw et al., Citation2008). In contrast, serum bilirubin (total as well as direct) is a conventional indicator of hepatic diseases. During the process of glucuronidation, bilirubin is taken by liver parenchyma cells from the blood and conjugates with glucoronic acid in the presence of enzyme glucuronyl-transferase and further this conjugated product is excreted into bile. Damage to hepatic parenchymal cells caused increased level of total bilirubin and direct bilirubin in serum (Chakrapani & Satyanarayana, Citation2013). Administration of APME decreased the level of total bilirubin and direct bilirubin level suggesting its role in the promotion of glucuronidation.
LDH is mainly present in the cell cytoplasm and it is extruded into the serum during cell injury or necrosis (Shiva Kumar et al., Citation2014). Serum LDH is a sensitive intracellular enzyme which is most useful in the diagnosis of hepatic damage (Kim et al., Citation2000). APAP caused a significant increase in the level of serum LDH which was restored to the normal value after APME treatment.
It has been well documented that oxidative stress also played an important role in the induction of APAP-induced toxicity which may result in the damage of some susceptible amino acids of proteins (Kandhare et al., Citation2013a). Decreased levels of serum albumin is most common in liver diseases which are followed by an elevated level of β and γ globulins via production of IgG and IgM (Kaplan et al., Citation2003). Spleen is a vital organ of the immune system and its relative weight has been found to increase with increased production of IgG and IgM. It has been previously reported that APAP-induced toxicity is associated with enlargement of the spleen (Spellberg, Citation1954). Hypoalbuminia was observed after APAP administration but the trend turns towards normal after APME treatment which in turn also decreased splenic enlargement.
Kidneys remove metabolic wastes such as urea, uric acid, creatinine, and ions. It has been reported that BUN, serum creatinine, and serum uric acid are the most important and reliable hallmark of renal dysfunction (Praveen et al., Citation2008; Visnagri et al., Citation2014). In the present investigation, on one hand, administration of APAP resulted in significant increase in BUN and, serum creatinine, on the other hand, serum uric acid level was significantly decreased after APAP administration. Similar results with APAP administration were reported earlier (Ahmad et al., Citation2012). APME treatment maintained these biochemical variables closer to those in normal rats which suggest that Artemisia pallens plays a role, either directly or indirectly, in providing protection against APAP-induced renal toxicity or delay in its development. The results of the present investigation are in accordance with the findings of previous researcher who showed that substantial reduction of BUN and serum creatinine level by administration of Artemisia campestris leaf extract in early diabetes induced nephropathy (Sefi et al., Citation2012).
It has been reported that APAP caused significant alteration in lipoprotein and cholesterol metabolism (Kobashigawa & Kasiske, Citation1997). Clinically it has been proven that high cholesterol levels and hyperlipidemia are associated with decreased oxygen release leading to vascular complications. The level of erythrocyte membrane peroxidation was significantly elevated in APAP toxicity that leads to hemolytic changes. With marked increase in cholesterol to phospholipids ratio, the microviscosity of cell membrane was significantly elevated leading to cellular rigidity (McConnell & Hubbell, Citation1971). Alteration in cell membrane structure is reflected by an increase in the cholesterol level which leads to impaired fluidity, permeability, activity of associated enzymes, and transport system. With increasing the availability of free fatty acids, the tryglycerides levels were significantly increased in APAP-induced toxicity that leads to decreased hepatic release of lipoprotein and increased esterification of free fatty acids. In the present study, these fatty changes were observed in the histological examination of liver tissue of APAP rats which showed the presence of few fatty globules with vesicular fat (grade 2) (). Treatment with APME showed significant reduction in the cholesterol and triglyceride. Histopathological evaluation of liver tissue from APME-treated rats () also supports these findings.
The liver plays a major role in protein metabolism and also in the synthesis of serum protein. Hypoproteinemia is a key feature of liver damage and decreased serum protein level occurs due to defect in protein biosynthesis by disruptions and disassociation of polyribosome from endoplasmic reticulum after APAP toxicity (Dubey et al., Citation1994). The serum albumin and globulin are the main components of total protein and mainly synthesized by the liver (Chakrapani & Satyanarayana, Citation2013). In APAP rats, the level of protein was decreased due to cirrhosis that in turn decreased the level of serum albumin. Treatment with APME significantly restored the altered level of total protein in the liver as well as kidney.
During the metabolism of APAP via glucuronidation and sulfuration reactions by the microsomal CYP-450 enzyme system in liver, a highly reactive intermediate, NAPQI, is produced (Mitchell et al., Citation1973). NAPQI has high affinity for GSH which causes depletion of cellular GSH leading to renal and hepatic damage (Mitchell et al., Citation1973). Generation of ROS played a pivotal role in the depletion of intracellular GSH and cell damage in APAP-induced toxicity (Manov et al., Citation2002). GSH is an abundant tripeptide, non-enzymatic biological antioxidant (Kandhare et al., Citation2012c,Citationd). The GSH redox cycle plays an important role in the detoxification of free radical species such as hydrogen peroxide, superoxide, and alkoxy radicals to maintain cell metabolism and integrity (Prakash et al., Citation2001). It has been reported that SOD is one of the most important enzymes in the enzymatic antioxidant defense system (Curtis et al., Citation1972; Patil et al., Citation2012a) and it reflects as the most sensitive enzymatic index in tissue toxicity caused by ROS and oxidative stress. It has a central role in detoxification of ROS via scavenging superoxide anion to form hydrogen peroxide (Patil et al., Citation2012b,Citationc). Therefore, reduction in the activity of SOD indicates the toxic effects of ROS produced by APAP. Consistent with previous studies (Olaleye & Rocha, Citation2008; Visarius et al., Citation1996), administration of APAP in the present investigation caused significant depletion in the SOD and GSH levels in renal as well as hepatic tissue. Results of the present investigation suggested that APME treatment significantly restored the declined level of intracellular SOD and GSH that plays an essential role in detoxification as well as prevention of APAP-induced liver and kidney toxicity.
An elevated level of ROS caused depletion of protective antioxidant moieties (SOD and GSH) resulting in widespread propagation of the alkylation as well as peroxidation, causing damage to the macromolecules in vital bio-membrane (Patil et al., Citation2012d; Pesh-Imam & Recknagel, Citation1977; Raygude et al., Citation2012a). It has been reported that lipoperoxidation is responsible for the destruction of cell membrane via rearrangement of the double bond in the unsaturated fatty acids in the lipid membrane (Kandhare et al., Citation2012a,Citationb). In the APAP-induced toxicity, lipid peroxidation has been postulated to initiate the destructive process of liver and kidney (Baskin & Salem, Citation1997). In the present investigation, elevated levels of MDA were observed in APAP rats as compared with normal rats which suggest enhanced lipid peroxidation leading to tissue damage and failure of antioxidant defense mechanisms. Treatment with APME showed significant reduction in the elevated level of MDA via its antioxidant potential to prevent the formation of excessive free radicals.
APAP-induced increased oxidative stress caused induction of vicious cycle which releases various pro-inflammatory mediators such as NO via inducible nitric oxide synthase resulting in cellular dysfunction (Ahmad et al., Citation2012; Kamble et al., Citation2013; Patil et al., Citation2011; Raygude et al., Citation2012b). Results of the present investigation are in agreement with the findings of the previous study where administration of APAP significantly increased NO level in liver as well as kidney (Ahmad et al., Citation2012; Michael et al., Citation1999). It has been documented that administration of a substance with NO scavenging or NOS (nitric oxide synthase) inhibitory potential prevented APAP-induced toxicity via inhibition of hepatic microvascular constriction to improve hemodynamic (Ishida et al., Citation2002). Reduction of elevated levels of NO by APME administration showed substantial protection bestowed to rats against APAP hepatotoxicity and nephrotoxicity.
The QSAR analysis carried out on a series of sesquiterpene lactones with respect to hepatoprotective activity by previous research showed that predictive ability (r2 test) lies within 0.942–0.969 (Paukku et al., Citation2009). It has been reported that Artemisia pallens contains sesquiterpene lactones (Puranik & Deshpande, Citation2010; Suresh et al., Citation2011). The presence of sesquiterpene lactones moieties in Artemisia pallens may contribute to its hepatoprotective potential.
In conclusion, oral administration of a methanol extract of Artemisia pallens promoted renal and hepatic antioxidant enzyme activity to protect against APAP-induced hepatotoxicity as well as nephro-toxicity. Results of the present investigation suggest that Artemisia pallens may find immense therapeutic potential in clinical application in a variety of conditions where cellular damage is a consequence of oxidative stress. However, further study is in progress for elucidation of actual mechanism of action of Artemisia pallens at the molecular level.
Acknowledgements
The authors acknowledge Dr. S. S. Kadam, Vice Chancellor, Bharati Vidyapeeth University and Dr. K. R. Mahadik, Principal, Poona College of Pharmacy for keen interest and providing the necessary facilities to carry out the study.
Declaration of interest
There is no conflict of interest between any of the authors.
References
- Ahmad ST, Arjumand W, Nafees S, et al. (2012). Hesperidin alleviates acetaminophen induced toxicity in Wistar rats by abrogation of oxidative stress, apoptosis and inflammation. Toxicol Lett 208:149–61
- Anderson B, Holford N, Armishaw J, Aicken R. (1999). Predicting concentrations in children presenting with acetaminophen overdose. J Pediatr 135:290–5
- Ashok P, Upadhaya K. (2010). Analgesic and anti-inflammatory properties of Artemisia pallens Wall Ex. DC. Pharm Res 3:249–56
- Baskin SI, Salem H. (1997). Oxidants, Antioxidants, and Free Radicals. Bristol (PA): CRC Press, Taylor & Francis, 193–202
- Chakrapani U, Satyanarayana U. (2013). Biochemistry, 4th ed. India: Elsevier
- Curtis S, Moritz M, Snodgrass P. (1972). Serum enzymes derived from liver cell fractions. I. The response to carbon tetrachloride intoxication in rats. Gastroenterol 62:84–92
- Drotman R, Lawhorn G. (1978). Serum enzymes as indicators of chemically induced liver damage. Drug Chem Toxicol 1:163–71
- Dubey G, Agrawal A, Dixit S. (1994). Effect of Liv. 52 on different bio-chemical parameters in alcoholic cirrhosis. Antiseptic 91:205–8
- Gaw A, Murphy M, Cowan R, et al. (2008). Clinical Biochemistry: An Illustrated Colour Text. 3rd ed. Churchill Livingstone: Elsevier Health Sciences
- Ghosh A, Sil PC. (2007). Anti-oxidative effect of a protein from Cajanus indicus L. against acetaminophen-induced hepato-nephro toxicity. J Biochem Mol Biol 40:1039–49
- Gosavi TP, Kandhare AD, Ghosh P, Bodhankar SL. (2012). Anticonvulsant activity of Argentum metallicum, a homeopathic preparation. Der Pharm Lett 4:626–37
- Hart SE, Beierschmitt WP, Wyand DS, et al. (1994). Acetaminophen nephrotoxicity in CD-1 mice: I. Evidence of a role for in situ activation in selective covalent binding and toxicity. Toxicol Appl Pharmacol 126:267–75
- Hegde K, Joshi AB. (2010). Hepatoprotective and antioxidant effect of Carissa spinarum root extract against CCl4 and paracetamol-induced hepatic damage in rats. Bangladesh J Pharmacol 5:73–6
- Ishida Y, Kondo T, Ohshima T, et al. (2002). A pivotal involvement of IFN-γ in the pathogenesis of acetaminophen-induced acute liver injury. FASEB J 16:1227–36
- Kamble H, Kandhare AD, Bodhankar S, et al. (2013). Effect of low molecular weight galactomannans from fenugreek seeds on animal models of diabetes mellitus. Biomed Aging Pathol 3:145–51
- Kandhare A, Raygude K, Ghosh P, Bodhankar S. (2011a). The ameliorative effect of fisetin, a bioflavonoid, on ethanol-induced and pylorus ligation-induced gastric ulcer in rats. Int J Green Pharm 5:236–43
- Kandhare AD, Bodhankar SL, Singh V, et al. (2013a). Anti-asthmatic effects of type-A procyanidine polyphenols from cinnamon bark in ovalbumin-induced airway hyperresponsiveness in laboratory animals. Biomed Aging Pathol 3:23–30
- Kandhare AD, Ghosh P, Ghule AE, Bodhankar SL. (2013b). Elucidation of molecular mechanism involved in neuroprotective effect of Coenzyme Q10 in alcohol induced neuropathic pain. Fund Clin Pharmacol 27:603–22
- Kandhare AD, Ghosh P, Ghule AE, et al. (2013c). Protective effect of Phyllanthus amarus by modulation of endogenous biomarkers and DNA damage in acetic acid induced ulcerative colitis: Role of phyllanthin and hypophyllanthin. Apollo Med 10:87–97
- Kandhare AD, Kumar VS, Adil M, et al. (2012a). Investigation of gastro protective activity of Xanthium strumarium L. by modulation of cellular and biochemical marker. Orient Pharm Exp Med 12:287–99
- Kandhare AD, Raygude KS, Ghosh P, et al. (2012b). Neuroprotective effect of naringin by modulation of endogenous biomarkers in streptozotocin induced painful diabetic neuropathy. Fitoterapia 83:650–9
- Kandhare AD, Raygude KS, Ghosh P, et al. (2012c). Therapeutic role of curcumin in prevention of biochemical and behavioral aberration induced by alcoholic neuropathy in laboratory animals. Neurosci Lett 511:18–22
- Kandhare AD, Raygude KS, Ghosh P, et al. (2012d). Effect of hydroalcoholic extract of Hibiscus rosa sinensis Linn. leaves in experimental colitis in rats. Asian Pac J Trop Biomed 5:337–44
- Kandhare AD, Raygude KS, Ghosh P, et al. (2011b). Patentability of animal models: India and the globe. Int J Pharm Biol Arch 2:1024–32
- Kaplan LA, Pesce AJ, Kazmierczak SC. (2003). Clinical Chemistry: Theory, Analysis, and Correlation. London: Mosby
- Kim K-A, Lee W, Kim J, et al. (2000). Mechanism of refractory ceramic fiber-and rock wool-induced cytotoxicity in alveolar macrophages. Int Arch Occup Environ Health 74:9–15
- Kobashigawa JA, Kasiske BL. (1997). Hyperlipidemia in solid organ transplantation. Transplantation 63:331–8
- Larson AM, Polson J, Fontana RJ, et al. (2005). Acetaminophen-induced acute liver failure: Results of a United States multicenter, prospective study. Hepatology 42:1364–72
- Manov I, Hirsh M, Iancu TC. (2002). Acetaminophen hepatotoxicity and mechanisms of its protection by N-acetylcysteine: A study of Hep3B cells. Exper Toxicol Pathol 53:489–500
- McConnell HM, Hubbell WL. (1971). Molecular motion in spin-labeled phospholipids and membranes. J Am Chem Soc 93:314–26
- Michael SL, Pumford NR, Mayeux PR, et al. (1999). Pretreatment of mice with macrophage inactivators decreases acetaminophen hepatotoxicity and the formation of reactive oxygen and nitrogen species. Hepatology 30:186–95
- Mitchell J, Jollow D, Potter W, et al. (1973). Acetaminophen-induced hepatic necrosis. IV. Protective role of glutathione. J Pharmacol Exp Ther 187:211–17
- Mitchell JR, Mcmurtry RJ, Statham CN, Nelson SD. (1977). Molecular basis for several drug-induced nephropathies. Am J Med 62:518–26
- Nassar I, Pasupati T, Judson JP, Segarra I. (2009). Reduced exposure of imatinib after coadministration with acetaminophen in mice. Ind J Pharmacol 41:167–72
- Nelson SD. (1990). Molecular mechanisms of the hepatotoxicity caused by acetaminophen. Semin Liver Dis 10:267–78
- Olaleye MT, Rocha B. (2008). Acetaminophen-induced liver damage in mice: Effects of some medicinal plants on the oxidative defense system. Exp Toxicol Pathol 59:319–27
- Patil M, Kandhare A, Bhise S. (2011). Pharmacological evaluation of ameliorative effect of aqueous extract of Cucumis sativus L. fruit formulation on wound healing in Wistar rats. Chronicles of Young Scientists 2:207–13
- Patil M, Kandhare A, Bhise S. (2012a). Anti-inflammatory effect of Daucus carota root on experimental colitis in rats. Int J Pharm Pharm Sci 4:337–43
- Patil MVK, Kandhare AD, Bhise SD. (2012b). Anti-arthritic and anti-inflammatory activity of Xanthium srtumarium L. ethanol extract in Freund's complete adjuvant induced arthritis. Biomed Aging Pathol 2:6–15
- Patil MVK, Kandhare AD, Bhise SD. (2012c). Effect of aqueous extract of Cucumis sativus Linn. fruit in ulcerative colitis in laboratory animals. Asian Pac J Trop Biomed 2:S962–9
- Patil MVK, Kandhare AD, Ghosh P, Bhise SD. (2012d). Determination of role of GABA and nitric oxide in anticonvulsant activity of Fragaria vesca L. ethanol extract in chemically induced epilepsy in laboratory animals. Orient Pharm Exp Med 12:255–64
- Paukku Y, Rasulev B, Syrov V, et al. (2009). Structure-hepatoprotective activity relationship study of sesquiterpene lactones: A QSAR analysis. Int J Quantum Chem 109:17–27
- Pesh-Imam M, Recknagel RO. (1977). Lipid peroxidation and the concept of antioxygenic potential: Vitamin E changes in acute experimental CCl4, BrCCl3, and ethanol-induced liver injury. Toxicol Appl Pharmacol 42:463–75
- Prakash J, Gupta S, Kochupillai V, et al. (2001). Chemopreventive activity of Withania somnifera in experimentally induced fibrosarcoma tumours in Swiss albino mice. Phytother Res 15:240–4
- Praveen S, Kumar KD, Venkatesan D, Sathendra ER. (2008). Effect of the ethanol extract of Indigofera barberi (L) in acute acetaminophen-induced nephrotoxic rats. Adv Biotech 7:28–31
- Prescott L. (1979). The third Lilly Prize Lecture. University of London, January, 1979. The nephrotoxicity and hepatotoxicity of antipyretic analgesics. Br J Clin Pharmacol 7:453–62
- Pujar PP, Sawaikar DD, Rojatkar SR, Nagasampagi BA. (2000). A new germacranolide from Artemisia pallens. Fitoterapia 71:590–2
- Puranik V, Deshpande N. (2010). GC-MS study and isolation of a sesquiterpene lactone from Artemisia pallens. Orient J Chem 26:143–6
- Raygude KS, Kandhare AD, Ghosh P, Bodhankar SL. (2012a). Anticonvulsant effect of fisetin by modulation of endogenous biomarkers. Biomed Prev Nutr 2:215–22
- Raygude KS, Kandhare AD, Ghosh P, et al. (2012b). Evaluation of ameliorative effect of quercetin in experimental model of alcoholic neuropathy in rats. Inflammopharmacology 20:331–41
- Rojatkar S, Pawar S, Pujar P, et al. (1996). A germacranolide from Artemisia pallens. Phytochemistry 41:1105–6
- Ruikar AD, Khatiwora E, Ghayal N, et al. (2011). Studies on aerial parts of Artemisia pallens wall for phenol, flavonoid and evaluation of antioxidant activity. J Pharm Bioall Sci 3:302–5
- Sefi M, Fetoui H, Soudani N, et al. (2012). Artemisia campestris leaf extract alleviates early diabetic nephropathy in rats by inhibiting protein oxidation and nitric oxide end products. Pathol Res Pract 208:157–62
- Shiva Kumar V, Rajmane AR, Mohammad A, et al. (2014). Naringin ameliorates acetic acid induced colitis through modulation of endogenous oxido-nitrosative balance and DNA damage in rats. J Biomed Res 28:132–45
- Sipma G, Van Der Wal B. (1968). The structure of davanone a new sesquiterpene from davana: (Artemisia pallens Wall.). Recueil des Travaux Chimiques des Pays-Bas 87:715–20
- Spellberg MA. (1954). Diseases of the liver. Am J Med Sci 228:243–8
- Starzl TE, Esquivel C, Gordon R, Todo S. (1987). Pediatric liver transplantation. Transplant Proc 19:3230–5
- Subramoniam A, Pushpangadan P. (1999). Development of phytomedicines for liver disease. Ind J Pharmacol 31:166–75
- Suresh J, Singh A, Vasavi A, et al. (2011). Phytochemical and pharmacological properties of Artemisia pallens. Int J 2:3081–90
- Valenzuela A, Garrido A. (1993). Biochemical bases of the pharmacological action of the flavonoid silymarin and of its structural isomer silibinin. Biol Res 27:105–12
- Visarius TM, Putt DA, Schare JM, et al. (1996). Pathways of glutathione metabolism and transport in isolated proximal tubular cells from rat kidney. Biochem Pharmacol 52:259–72
- Visnagri A, Kandhare AD, Chakravarty S, et al. (2014). Hesperidin, a flavanoglycone attenuates experimental diabetic neuropathy via modulation of cellular and biochemical marker to improve nerve functions. Pharm Biol 52:814–28
- Visnagri A, Kandhare AD, Ghosh P, Bodhankar SL. (2013). Endothelin receptor blocker bosentan inhibits hypertensive cardiac fibrosis in pressure overload-induced cardiac hypertrophy in rats. Cardiovasc Endocrinol 2:85–97
- Visnagri A, Kandhare AD, Shiva Kumar V, et al. (2012). Elucidation of ameliorative effect of co-enzyme Q10 in streptozotocin-induced diabetic neuropathic perturbation by modulation of electrophysiological, biochemical and behavioral markers. Biomed Aging Pathol 2:157–72
- Watkins PB, Seeff LB. (2006). Drug-induced liver injury: Summary of a single topic clinical research conference. Hepatology 43:618–31
- Yamasaki ML, Sasaki K, Mizutani N, et al. (2001). Pharmacological characterization of the leukocyte kinetics after intranasal antigen challenge in a guinea pig model of allergic rhinitis. Inflamm Res 50:474–82