Abstract
Context: Plants transformed by virus-based vectors have emerged as promising tools to rapidly express large amounts and inexpensive antigens in transient condition.
Objective: We studied the possibility of transient-expression of an HBsAg-fused polytopic construct (HCVpc) [containing H-2d and HLA-A2-restricted CD8+CTL-epitopic peptides of C (Core; aa 132-142), E6 (Envelope2; aa 614-622), N (NS3; aa 1406-1415), and E4 (Envelope2; aa 405-414) in tandem of CE6NE4] in tobacco (Nicotiana tabacum) leaves for the development of a plant-based HCV vaccine.
Materials and methods: A codon-optimized gene encoding the Kozak sequence, hexahistidine (6×His)-tag peptide, and HCVpc in tandem was designed, chemically synthesized, fused to HBsAg gene, and inserted into Potato virus X (PVX-GW) vector under the control of duplicated PVX coat protein promoter (CPP). The resulted recombinant plasmids (after confirmation by restriction and sequencing analyses) were transferred into Agrobacterium tumefaciens strain GV3101 and vacuum infiltrated into tobacco leaves. The effect of gene-silencing suppressor, p19 protein from tomato bushy stunt virus, on the expression yield of HCVpc–HBsAg was also evaluated by co-infiltration of a p19 expression vector.
Results: Codon-optimized gene increased adaptation index (CAI) value (from 0.61 to 0.92) in tobacco. The expression of the HCVpc–HBsAg was confirmed by western blot and HBsAg-based detection ELISA on total extractable proteins of tobacco leaves. The expression level of the fusion protein was significantly higher in p19 co-agroinfiltrated plants.
Discussion and conclusion: The results indicated the possibility of expression of HCVpc–HBsAg constructs with proper protein conformations in tobacco for final application as a plant-derived HCV vaccine.
Introduction
Hepatitis C virus (HCV), a globally expanding infective virus and the major cause of blood-borne chronic hepatitis, has already infected around 170 million people worldwide, while around 3–4 million infected individuals are also added to this reservoir each year (Hajarizadeh et al., Citation2013). A major fraction of these patients develop chronic disease that may advance to cirrhosis and hepatocellular carcinoma in time (Chen & Morgan, Citation2006). The only well-established treatment [ribavirin + interferon (IFN)-a] is effective in less than 50% of the patients and, recently presented, “Direct Acting Antivirals (DAAs)” are also extremely expensive and might be available only in a few developed countries in near future (Fauvelle et al., Citation2013). Despite these alarming rates and extensive studies during the last two decades, no approved vaccine is available for this viral pathogen and fatal disease to date (Chevaliez & Pawlotsky, Citation2012; Fauvelle et al., Citation2013).
HCV has a single-stranded positive-sense RNA genome encoding three structural (core, E1, and E2) and six non-structural proteins (NS2–NS5) (Roohvand & Kossari, Citation2011). The occurrence of high mutation rates in HCV antigens and the generation of quasi-species are the major obstacles against preparation of an efficient vaccine for this infection (Chevaliez & Pawlotsky, Citation2012). Identification of conserved epitopes in HCV proteins and advancements in the formulation of vaccines in novel modalities, however, resulted in the availability of a few HCV vaccines for human clinical trials in recent years (Roohvand & Kossari, Citation2011, Citation2012). In fact, prior immunization studies indicated that vigorous and multi-specific CD8+-cytotoxic T-lymphocyte (CTL)/T helper cell(Th)1 responses as well as high IFN-γ levels seem to play a significant role in clearance of the virus (Roohvand & Kossari, Citation2011). Accordingly, design and application of epitope-based immunogens by arranging isolated CTL epitopes (8–10 amino acids) in the context of synthetic multi-epitopic/polytopic HCV vaccines have been reported (Arashkia et al., Citation2010; Memarnejadian & Roohvand, Citation2010; Memarnejadian et al., Citation2009). Polytope vaccine studies were also described for different tumors and infectious diseases (Suhrbier, Citation2002). The main advantages of multi-epitope (polytope) vaccine modalities include the co-delivery of multiple conserved and crucial epitopes from various antigens, and preventing deleterious effects encountered with the application of entire proteins (Arashkia et al., Citation2010; Huang et al., Citation2013; Mishra et al., Citation2014).
The use of plants as a novel approach to provide safe and inexpensive source of biopharmaceutical and vaccine antigens for human diseases has several practical advantages compared with fermentation or cell-culture systems (Memari et al., Citation2010; Ohadi et al., Citation2013). These include reduced manufacturing cost, delivery by the oral route (in which downstream processing costs will also be reduced), the ease of agricultural scale production, and safety from contaminating human or animal pathogens (Fischer et al., Citation2004). Over the past two decades, a number of vaccine antigens have been expressed in different types of plants (Daniell et al., Citation2009) and several plant-derived viral proteins such as hepatitis B surface antigen (Guan et al., Citation2010) and Norwalk virus Capsid protein (Lai & Chen, Citation2012) are in clinical trial vaccine studies. There are a few studies addressing the application of plants for HCV antigen production too. In one of the first studies, a consensus sequence of hypervariable region 1 (HVR1), a potential neutralizing epitope of HCV, was genetically fused to the C-terminal of the B subunit of cholera toxin (CTB). Mice immunized with intranasal administration of tobacco extract containing HVR1/CTB elicited anti-HVR1 antibodies which are specifically bound to HCV VLPs (Nemchinov et al., Citation2000). Recently, successful application of cucumber (Natilla et al., Citation2004; Nuzzaci et al., Citation2007; Piazzolla et al., Citation2005), potato virus X (Uhde-Holzem et al., Citation2010), and papaya mosaic viruses (Denis et al., Citation2007) for the expression of the same mimotopes derived from the HVR1 region and E2 epitope, respectively, with proper immunogenicity is also reported. More recently, transgenic tobacco plants carrying HCV core gene were generated and expressed protein was shown to be immunoreactive, with both anti-core antibodies and HCV-infected human serum (Nianiou et al., Citation2008). In addition, successful expression of the first 143 amino acid N-terminal of HCV core protein in tobacco chloroplasts was also recently reported (Madesis et al., Citation2010).
Although these prior reports indicated the possibility of expression of HCV antigens in plants by generation of transgenic (stable) plants for HCV antigens (HCV core in tobacco) or by the whole virus (for mimotopes of HVR1 and E2 epitope), there are no reports on transient expression of HCV polytopic constructs specially via application of agroinfiltration technique. However, advantages like simplicity and feasibility of rapid protein expression with no tissue culture and regeneration costs have promoted plant transient expression to become a system of choice for industrial production scale (Komarova et al., Citation2010; Mett et al., Citation2008; Meyers et al., Citation2008). The production of seasonal influenza vaccines in tobacco could be considered as a successful example of transient expression in plants (Shoji et al., Citation2012). For a better expression result in plants, several factors have to be considered such as codon-optimization of the heterologous gene according to plant codon adaptation index (Love et al., Citation2012; Perlak et al., Citation1991), selection of the proper expression vector (Gleba et al., Citation2005; Hefferon, Citation2012), and efficient suppression of the post-transcriptional gene silencing (PTGS) phenomena that limit the expression of foreign genes in plants. One of the most promising methods to interfere with PTGS thereby preventing the silencing of transgen relies on the co-expression of viral suppressors. In particular, the 19 kDa gene product (P19) of TBSV is known as a potent suppressor which binds to short-interfering RNA (siRNA) as a dimer and suppressor of the RNA silencing pathway with high selectivity (Scholthof, Citation2006; Voinnet et al., Citation2003).
We have recently described in silico design and construction, novel synthetic polytope arrangements based on four immunodominant murine and human-restricted CTL epitopes, which are derived from HCV structural and NS antigens. The polytopes demonstrated their successful expression in mammalian cells and elicited CD8 + responses towards the H-2D epitopes of the constructed polytopes in immunized BALB/c mice (Memarnejadian et al., Citation2009). Our findings indicated the potency of Hepatitis B virus surface antigen (HBsAg)-based plasmo-virus like particles (VLPs) as a promising strategy for the improvement of multi-CTL epitope vaccines when fused with HBsAg, even in the absence of cognate CD4+epitopes (Memarnejadian & Roohvand, Citation2010).
In the present study, we describe construction and transient expression of the C-terminally HBsAg-fused and highly codon-optimized gene version of the same HCV polytope construct (HCVpc) by a potato virus X-based vector (PVX) in the Iranian jafarabadi-tobacco plant cultivar which is an accessible high biomass producer in Iran. In addition, the impact of the viral suppressor p19 to improve of the yield in recombinant protein production was evaluated.
Materials and methods
Codon optimization of the polytope gene and construction of the vectors
The pcPOL-CE6NE4 plasmid encoding H-2d and HLA-A2-restricted CD8+CTL-epitopic peptides of C (Core; aa 132–142), E6 (Envelope2; aa 614–622), N (NS3; aa 1406–1415), and E4 (Envelope1; aa 405–414) in tandem of CE6NE4 polytopic construct () (Memarnejadian & Roohvand, Citation2010) was used as the source of the sequence information for CE6NE4 polytope. A pcDNA vector harboring the HBsAg (pcDNA–HBsAg) was used as the source of HBsAg (Memarnejadian et al., Citation2009) for the construction of polytope–HBsAg plant expression vectors. The PVX-GW vector (Lacorte et al., Citation2010), which was kindly gifted by Dr. Cristiano Lacorte (EMBRAPA Recursos Genéticos e Biotecnologia, Brasília, Brazil), was used as the PVX-based viral vector.
Figure 1. The schematic presentation of the HCVpc (CE6NE4) with encoding amino acids (Memarnejadian & Roohvand, Citation2010) which was employed in this study (A) and the nucleotide sequence comparison between original and tobacco plant optimized nucleotides in the corresponding HCVpc (B). The changes to the original sequence are shown by lower cases. Location of the Kozak sequence and 6×His-tag nucleotides are indicated. “ATG” denote the “Start” codons.
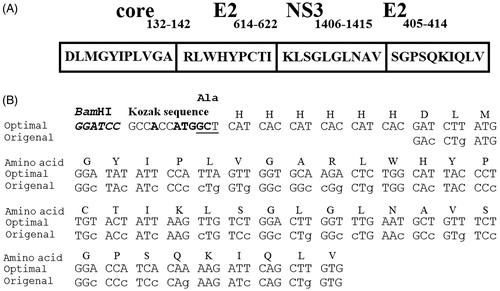
Several modifications for optimized (plant) codon-usage of nucleotide sequence for the CE6NE4 polytope were considered including (i) codon optimization according to codon adaptation index (Sharp & Li, Citation1987) for the expression in Nicotiana tabacum L. and (ii) addition of Kozak (GCCACCATGGC) sequence (Kozak, Citation1989) and hexahistidine (6×His)-tag for nickel affinity purification at 5′ site (). The plant-optimized CE6NE4 gene for recombinant expression in tobacco was chemically synthesized and delivered as a clone in pUC57 plasmid (pUC-polytope) by ShineGene Molecular Biotech, Inc. (Shanghai, China).
For the construction of the HCVpc–HBsAg, the synthetic gene was amplified in a PCR reaction using pfu enzyme and two primers: F-polytope and R-polytope harboring NheI and HindIII restriction sites as forward and reverse primers, respectively (), employed pUC-polytope as a template. PCR amplification was performed with 25 cycles at 94 °C for 30 s, 58 °C for 30 s, 72 °C for 90 s, and 72 °C for 15 min. The 170 bp amplicon was subsequently digested by Nhe I and Hind III enzymes and cloned into the same sites of pcDNA–HBsAg vector to insert the CE6NE4 gene upstream of HBsAg sequence to produce pcDNA–polytope–HBsAg construct (). For the construction of HCVpc–HBsAg plant expression vector, the corresponding sequence was PCR amplified (in the same condition mentioned above) from pcDNA–polytope–HBsAg construct using the following prime pairs: F kozak-VX and R HBs-VX harboring ClaI and SalI restriction sites as forward and reverse primers, respectively (). The 870 bp amplicon was subsequently digested by ClaI and SalI enzymes and cloned into the same sites of PVX-GW vector under the control of the duplicated PVX-coat protein subgenomic-promoter (CPP) to produce the pVX-poly-HBs construct ().
Table 1. List of the primers.
The constructs were confirmed by restriction and sequencing analyses using F kozak-VX and R HBs-VX specific primers. All cloning and molecular procedures were conducted according to the standard protocols (Sambrook & Russell, Citation2001).
Transformation of Agrobacterium tumefaciens by plant expression vector
Agrobacterium tumefaciens strain GV3101 harboring the helper plasmid pSOUP (Hellens et al., Citation2000) which is essential for the replication of pVX-GW based on pGreen plasmids in Agrobacterium species was transformed with the pVX–poly-HBs vector () via standard freeze-thaw protocol (Höfgen & Willmitzer, Citation1988). Subsequently, transformed Agrobacteria cells were selected on plates containing 100 µg/ml Rifampicin (RIF) and 50 µg/ml Kanamycin (KAN) and incubated for 72 h at 28 °C. Transformed colonies were confirmed by gene-specific colony PCR using F kozak-VX and R HBs-VX specific primers as described above. All chemicals, culture media, and antibiotics were purchased from Sigma (St. Louis, MO) and Merck (Darmstadt, Germany) companies.
Co-agroinfiltration of N. tabacum leaves by p19 silencing-suppressor protein
Suppressing of silencing of gene expression in tobacco was conducted by co-infiltratration of Agrobacterium strain LB4404 harboring p19 silencing-suppressor gene construct from tomato bushy stunt virus (TBSV) (Voinnet et al., Citation2003) (GenBank accession number: M21958). The gene was previously cloned into pCAMBIA 1304 vector (CAMBIA Co., Canberra, Australia) in our lab constructing p19-pCAMBIA 1304-vector. The agroinfiltration protocol was done. In brief, the Agrobacteria cultures were first adjusted to an OD600 value of 1.0 with MS medium supplemented with 2% (w/v) sucrose, 10 mM MES pH 5.5, 10 mM MgCl2, and induced for an additional 3 h with 400 µM acetosyringone (Kapila et al., Citation1997). The Agrobacteria suspensions were subsequently mixed in equal ratios for p19 plasmid and pVX–poly-HBs expression construct. The leaves of N. tabacum cultivar Jafarabadi (kindly provided by Dr. Tohidfar, Agricultural Biotechnology Research Institute of Iran) were then immersed in the above suspension while a vacuum of 0.5 mbar was applied for 2 min. The infiltrated leaves were incubated at 23 °C with a 16-h photoperiod for 5 d on wet Whatman paper in glass containers.
Protein extraction
The infiltrated tobacco leaves were grounded using liquid nitrogen, mortar, and pestle into a fine powder. Subsequently, 0.5 ml extraction buffer was added per gram of leave containing 50 mM NaH2PO4, pH 8, 300 mM NaCl, 0.1% Tween 20, 10 mM β-Mercaptoethanol, 1 mM PMSF, and 5 mM EDTA. The total soluble protein (TSP) was separated using centrifugation for 20 min at 4 °C (14 000 rpm). Protein concentrations were determined by Bradford assay (Bradford, Citation1976) using bovine serum albumin (BSA) as standard.
Western blot analyses
For western blotting, an equal concentration of TSP (30–50 µg) from plant extracts (transformed as sample and non-transformed as control) was loaded to a 12% SDS-acrylamide gel and, by the end of electrophoresis protein, bands were transferred to the nitrocellulose membrane according to standard protocol (Towbin et al., Citation1979) and coated with 3% BSA. Subsequently and after several washes, the corresponding protein bands were identified with anti-HBs monoclonal Ab (Cedarlane, Burlington, Canada) treatment followed by goat anti-mouse IgG/HRP conjugate (Jackson Immunoresearch, West Grove, PA) tracking and DAB (Roche, Mannheim, Germany) substrate resolution.
ELISA analyses
The ELISA method was performed on total soluble proteins extracted from leaves using the commercial HBsAg-ELISA kit (DIA PRO Diagnostic Bioprobes, Milan, Italy). In brief, 50 μg of appropriately diluted TSP of each plant extract was added to coated wells with a mix of mouse monoclonal antibodies specific to HBsAg determinants (“a”, “d”, and “y”) in duplicate. After 1 h incubation at 37 °C and several washing steps, a second mixture of horseradish peroxidase (HRP)-conjugated monoclonal antibodies (specific to a different epitope of the determinant “a” and against “preS”) were dispensed into the wells and incubation continued at 37 °C for 1 h. Finally, substrate and color development solutions were added and the absorbance was measured at 450 nm. As recommended by the manufacturer, the cut-off value was calculated as follows: cut-off = OD450 for negative control + 0.050. Test results were interpreted as a ratio of the sample OD450 (S) and the cut-off value (Co), (S/Co). The sample was considered positive if S/Co value was 0.9–1.1 or > 1.1 and negative if it was <0.9.
Statistical analyses
Prism 5.0 software (GraphPad Software Inc., San Diego, CA) was used for the data handling and results are presented as means ± standard error of the mean (SEM). Between-group comparisons were performed by one-way ANOVA, followed by the Bonferroni test. Statistical significance was set at p ≤ 0.05.
Results
Codon-optimization of polytope gene (CE6NE4) for efficient expression in plant
represents the nucleotide changes applied in the basal sequence of CE6NE4 in order to improve its codon-utilization for efficient expression in plants. Other elements (Kozak Citation1989, His-tag) were also included in the CE6NE4 basal sequence (). As shown in , the corresponding nucleotide alterations increased the codon adaptation index (CAI) value from 0.61 to 0.92 and reduced the GC content from 65.33 to 40.57 (). Results of DNA sequencing (not shown) confirmed that the Kozak (GCCACCATGGC) sequence (Kozak, Citation1989) was properly situated upstream of the CE6NE4 sequence ().
Figure 2. The distribution of codon usage frequency along the length of the HCVpc (CE6NE4) gene sequence before (right) and after (left) optimization for expression in N. tabacum. (A) The corresponding nucleotide alterations increased the codon adaptation index (CAI) value from 0.61 to 0.92 (the frequency of the non-optimized bases is decreased after optimization). The value of 100 is set for the codon with the highest usage frequency. (B) The corresponding nucleotide alterations reduced the GC content from 65.33 to 40.57.
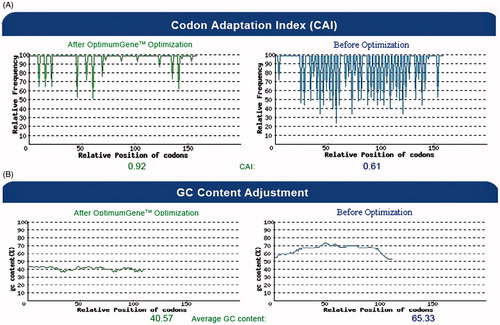
Construction of the vectors
represents the schematic of the construction steps for the pcDNA–polytope–HBsAg vector and represents the insertion of the HCV pol–HBsAg under the control of CP promoter in pVX–poly-HBs vector, upstream of the NOS-Ter transcriptional terminator. Agarose gel electrophoresis of the PCR-amplified inserts () and restriction-analyzed sequences indicated the proper amplified sizes for three fusion clones. Later, the gene was cloned in PVX vector and sequencing results (not shown) confirmed the insertion of the fusion gene with no alterations in the nucleotides. The resulting plasmids were transferred into A. tumefaciens GV3101 target hosts. Transformed Agrobacteria were screened for recombinant constructs using specific primers (F-kozak-VX and R-HBs-pVX) and it was confirmed by colony-PCR assay. One positive clone was subsequently used for agroinfiltration of N. tabacum leaves by p19 silencing-suppressor gene co-expression procedure as outlined in the Method section.
Figure 3. Schematic diagram for the construction of HCVpc–HBsAg segment (A) and agarose gel electrophoresis analyses results for different DNA fragments (B). (A) Strategy for the construction of fusion gene (see text for details); (a) pUC-polytope plasmid containing 150 bp polytope fragment, (b) pcDNA plasmid containing 680 bp HBsAg fragment, (c) constructed pcDNA–polytope–HBsAg. (B) Colony PCR analysis; lane 1: 10 kb DNA ladder, lanes 2, 3, and 5: positive fusion clones (the 870 bp fragment was amplified by the fusion polytope-HBsAg-specific primers), lane 4: positive fusion clone (the 170 bp fragment was amplified by polytope-specific primers), lane 6: intact pcDNA vector as negative control.
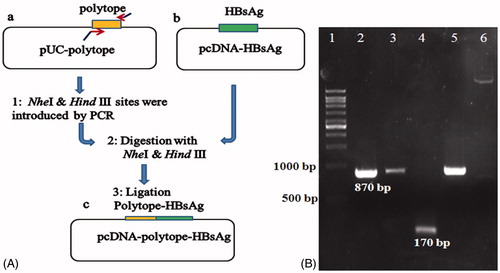
Figure 4. Schematic diagram of the constructed pVX–poly-HBs vector (A) and Western blotting analysis (B). (A) The synthetic and optimized gene after fusion to HBsAg was inserted into the ClaI/SalI sites of PVX-GW vector under the control of duplicated PVX-coat protein subgenomic-promoter (CPP). (B) Western blotting: lane 1: leaves agroinfiltrated with pVX-poly-HBs, lane 2: Prestained Protein Ladder (Fermentas, Amherst, NY). Lane 3: negative control (tobacco leaves transformed by pVX vector alone; i.e., without HCVpc–HBsAg gene). In lanes 1 and 3, around 50 µg of plant TSP were applied in each lane. Appearance of the doublet bands (indicated arrows) could be due to the presence of glycosylated and non-glycosylated forms of HBsAg (about 33 and 30 kDa) in the expressed HCVpc–HBsAg.
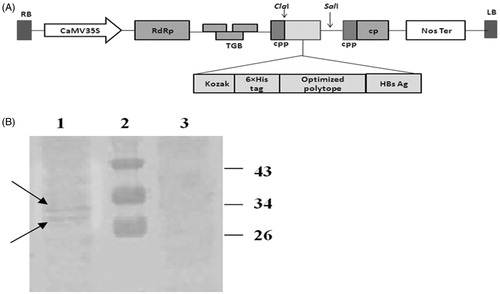
Analyses of HCVpc–HBsAg expression in tobacco leaves by Western blotting
As shown in , results of western blot analysis on protein extracted from tobacco leaves using anti-HBs Ab indicated that the expression of HCVpc–HBsAg in tobacco leaves produced two distinct protein bands attributed to the unglycosylated and glycosylated forms of HBsAg, with about 3-kDa difference in the molecular weight, which is in accordance with prior reports for the expression of HBsAg (Jin et al., Citation2002). These results imply to the invariant post-translational modification and folding of HBsAg after the addition of ectopic epitopes and are in accordance with prior observations (Memarnejadian et al., Citation2009).
Analysis of the expression levels of HCVpc–HBsAg in tobacco leaves by ELISA
Confirmation of the expression for HCVpc–HBsAg protein by pVX–poly-HBs construct in tobacco leaves and its expression level in the presence and absence of p19 co-agroinfiltration was analyzed by ELISA. The tobacco leaves transformed by PVX (vector without HCVpc–HBsAg insert) and co-agroinfiltrated with p19 constructs were used as negative control and determination of the cut-off value (). The ELISA test showed that the tobacco leaves transformed with pVX-poly-HBs are positive for HBsAg. The comparison of ELISA results indicated that the expression level of pVX–poly-HBs in the presence of p19 co-agroinfiltration was significantly higher than in its absence (p < 0.05) ().
Figure 5. Analysis of the expression of HCVpc-HBsAg in tobacco leaves in the absence and presence of p19 co-agroinfiltration by ELISA. The expression of HCVpc–HBsAg by pVX–poly-HBs in tobacco leaves was compared in the presence or absence of co-agroinfiltration with gene-silencing suppressor p19 construct by ELISA. Leaf control that denotes the tobacco leaves transformed by pVX vector alone (i.e., without HCVpc–HBsAg gene) in the presence of co-agroinfiltration with p19 construct. The experiment was repeated three times at least.
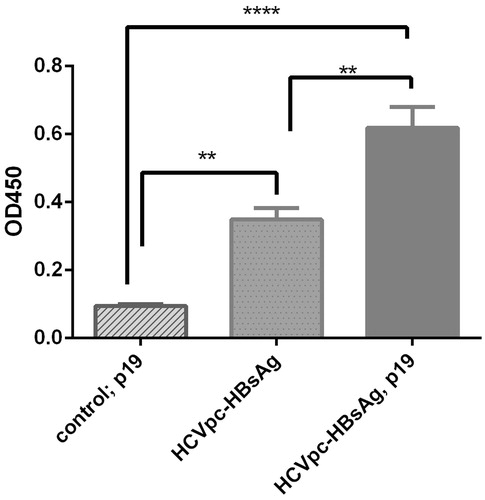
Discussion
In the present study, we evaluated a regionally adapted tobacco-host (the Iranian jafarabadi-tobacco plant cultivar) for transient expression of an HCVpc which has been considered as a vaccine candidate. Due to the production of high amount of leaf biomass, tobacco is the plant of choice for the application of agroinfiltration which is the selected system for the production of recombinant protein in plant leaves. In addition, because of extensive studies and information on tobacco cell molecular biology as a model plant, tobacco might be the first choice in such studies (Tremblay et al., Citation2010). Although other tobacco plants such as Australian Nicotiana species, Nicotiana benthamiana is a well-known and hyper-susceptible host for plant-derived recombinant protein expression (Goodin et al., Citation2008), but the Iranian jafarabadi-tobacco plant cultivar is one of the currently available and adapted tobacco plants with high leaf mass in our hands in Iran. For this purpose, the designed HCV polytope gene, which includes 47 N-terminally amino acids of fusion protein, was adapted based on the codon usage of highly expressed nuclear-encoded genes of N. tabacum (Perlak et al., Citation1991). In agreement with this, codon optimization within approximately 40 amino acids of the N-terminus could be particularly considered as significant in increasing recombinant protein production (Batard et al., Citation2000). In this respect as shown in , the codon-optimization approach has increased the CAI value of HCV polytope, from 0.61 to 0.92 and in favor of codon-utilization for N. tabacum (Sharp & Li, Citation1987). The plant preference for guanine at position +4 and Cytosine at position +5 (85% and 77%, respectively) of Kozak sequence (Lütcke et al., Citation1987) has been shown for plants. Therefore, the GCT sequences (GCCACCATGGCT) which encodes for alanine (a nonpolar amino acid) were added following the ATG codon ().
In two recent decades, HBsAg was expressed extensively in various plant hosts preserving the wild-type DNA sequence (Pniewski, Citation2013). In addition, Santi et al. (Citation2006) have shown that the expressed HBsAg could assemble as 22 nm virus like particles. The latter advantage could bring better immune response for polytope-HBsAg fusion protein that carries native HBsAg with optimized polytope-coding sequences; however, in our study, its conformation has not been tested.
In our study, for transient expression of the recombinant protein in plant, PVX-based vector, a viral replicative vector was employed (Lacorte et al., Citation2007). The reason behind this selection was the higher expression levels achieved by plant–viral replicate vectors compared with classical binary vector (Komarova et al., Citation2011). In addition, transgenic plant generation needs longer time periods and shows more deleterious effect because of gene silencing (Nianiou et al., Citation2008) in contrary to transient-expression approach (Peach & Velten, Citation1991). Therefore, the latter has been applied as method of choice due to the simplicity and feasibility of rapid protein production with no tissue culture and regeneration costs; and, moreover, it is adapted for large-scale industrial production plans disregarding the nature of the protein (toxic/non toxic) (Komarova et al., Citation2010; Mett et al., Citation2008; Meyers et al., Citation2008). In addition, using Agrobacterium infiltrating strategy for delivery of viral-based expression vector into leaf tissues has the benefit of cassette delivery into many plant cells which eliminates incubation time for the spread of natural viral infection in plants (4 d versus 2 weeks). This is advantageous over direct inoculation of viral genome. Moreover, in the recent study, the agroinfiltration method has been adjusted to co-delivery of gene suppressing silencer p19 to survey the effect on the expression level of polytope–HBsAg fusion protein.
The results of Western blotting using anti-HBs indicated the expression of the HCVpc–HBsAg protein by the pVX-poly-HBs construct in tobacco leaves (). The fusion protein amino acid sequence (HCV polytope–HBsAg) was evaluated for potential glycosylation sites using several glycosylation prediction servers (NetOGlyc 4.0, http://www.cbs.dtu.dk/services/NetOGlyc/;.NetNGlyc.1.0,http://www.cbs.dtu.dk/services/NetNGlyc/ and GlycoEP http://www.imtech.res.in/raghava/glycoep/). As expected and in accordance with prior report (Memarnejadian et al., Citation2009), results indicated potential glycosylation of the fusion protein for HBsAg-fused section, while HCV polytope peptide showed no potential N-glycosylation or O-glycosylation sites (results not shown). Accordingly, the detected protein bands appeared as two distinct ones attributed to the unglycosylated and glycosylated forms of HBsAg, with around 3-kDa difference in molecular weight () (Jin et al., Citation2002), which is in accordance with our prior results in the expression of N-terminally fused HBsAg–CE6NE4 construct in mammalian cells (Memarnejadian et al., Citation2009). These final results, in accordance with other reports on the application of HBsAg as a vaccine carrier and/or particulate-forming factor, imply to the post-translational modification of HBsAg after the addition of ectopic epitopes in either N- or C-terminal of this antigen (Hui et al., Citation1999; Jin et al., Citation2002). According to several analyses, glycosylation of HBsAg proteins occurs in plant cells as well (Ehsani et al., Citation1997). Existence of a potential N-glycosylation site in the “a” determinant region of HBsAg and recruiting monoclonal antibodies against “a” determinant in the ELISA test could impact the detection of fusion protein in both preparations evenly.
Prevention of silencing mechanisms is one of the key elements for obtaining high-expression levels in plants. It is shown that co-expression of viral-silencing suppressor proteins such as p19, potyvirus P1/HC-Pro, eliminates the effects of post-transcriptional gene silencing (PTGS) in the transient expression system (Komarova et al., Citation2010). Application of p19-mediated co-agroinfiltration has already shown to improve the recombinant expression in plants by several folds (Voinnet et al., Citation2003). The recent reports on expression levels of SARS-CoV N (Zheng et al., Citation2009), antibodies (Komarova et al., Citation2011), and GFP (Komarova et al., Citation2012) proteins in the presence of p19 have shown 3–5 to 100-folds increase of recombinant protein. Our ELISA result analyses are in agreement with the above reports. We have shown that the production of p19 enhances the HCVcp–HBsAg expression level significantly (p < 0.05) ().
In conclusion, the present report is the first (to the best of our knowledge) to address construction of a transient-tobacco expression system for HCV polytopic–HBsAg fusion construct. To have the highest possible expression efficiency, the codon optimized (based on the codon adaptation index for nuclear-encoded genes of tobacco) HCVpc DNA sequence fused to HBsAg was designed downstream of Kozak sequence. Following its insertion into the PVX-based viral vector, the levels of expressed protein in the presence and absence of p19 co-agroinfilteration were compared. Results indicated the significant effect of our design to enhance the expression levels for HCVpc. To our knowledge, all the above-mentioned reports are novel regarding HCVpc and provide valuable information for plant-based transient expression studies on polytopic fusion construct. The next steps might be the evaluation of plant-derived HCVpc for immunization studies.
Acknowledgements
The present study has been in part to fulfill the thesis of Mrs. S. Mohammadzadeh in Ph.D. Program of Medical Biotechnology, Graduate School of Pasteur Institute of Iran. The authors would like to acknowledge Mrs. Rahimeh Rasouli for her technical assistance.
Declaration of interest
The authors have no competing interests. The recent work was financially supported by Pasteur Institute of Iran.
References
- Arashkia A, Roohvand F, Memarnejadian A, et al. (2010). Construction of HCV-polytope vaccine candidates harbouring immune-enhancer sequences and primary evaluation of their immunogenicity in BALB/c mice. Virus Genes 40:44–52
- Batard Y, Hehn A, Nedelkina S, et al. (2000). Increasing expression of P450 and P450-reductase proteins from monocots in heterologous systems. Arch Biochem Biophys 379:161–9
- Bradford MM. (1976). A rapid and sensitive method for the quantitation of microgram quantities of protein utilizing the principle of protein-dye binding. Anal Biochem 72:248–54
- Chen SL, Morgan TR. (2006). The natural history of hepatitis C virus (HCV) infection. Int J Med Sci 3:47–52
- Chevaliez S, Pawlotsky JM. (2012). Virology of hepatitis C virus infection. Best Pract Res Clin Gastroenterol 26:381–9
- Daniell H, Singh ND, Mason H, Streatfield SJ. (2009). Plant-made vaccine antigens and biopharmaceuticals. Trends Plant Sci 14:669–79
- Denis J, Majeau N, Acosta-Ramirez E, et al. (2007). Immunogenicity of papaya mosaic virus-like particles fused to a hepatitis C virus epitope: Evidence for the critical function of multimerization. Virology 363:59–68
- Ehsani P, Khabiri A, Domansky NN. (1997). Polypeptides of hepatitis B surface antigen produced in transgenic potato. Gene 190:107–11
- Fauvelle C, Lepiller Q, Felmlee DJ, et al. (2013). Hepatitis C virus vaccines – Progress and perspectives. Microb Pathog 58:66–72
- Fischer R, Stoger E, Schillberg S, et al. (2004). Plant-based production of biopharmaceuticals. Curr Opin Plant Biol 7:152–8
- Gleba Y, Klimyuk V, Marillonnet S. (2005). Magnifection – A new platform for expressing recombinant vaccines in plants. Vaccine 23:2042–8
- Goodin MM, Zaitlin D, Naidu RA, Lommel SA. (2008). Nicotiana benthamiana: Its history and future as a model for plant-pathogen interactions. Mol Plant Microbe Interact 21:1015–26
- Guan ZJ, Guo B, Huo YL, et al. (2010). Overview of expression of hepatitis B surface antigen in transgenic plants. Vaccine 28:7351–62
- Hajarizadeh B, Grebely J, Dore GJ. (2013). Epidemiology and natural history of HCV infection. Nat Rev Gastroenterol Hepatol 10:553–62
- Hefferon KL. (2012). Plant virus expression vectors set the stage as production platforms for biopharmaceutical proteins. Virology 433:1–6
- Hellens RP, Edwards EA, Leyland NR, et al. (2000). pGreen: A versatile and flexible binary Ti vector for Agrobacterium-mediated plant transformation. Plant Mol Biol 42:819–32
- Höfgen R, Willmitzer L. (1988). Storage of competent cells for Agrobacterium transformation. Nucleic Acids Res 16:9877
- Huang XJ, Lü X, Lei YF, et al. (2013). Cellular immunogenicity of a multi-epitope peptide vaccine candidate based on hepatitis C virus NS5A, NS4B and core proteins in HHD-2 mice. J Virol Methods 189:47–52
- Hui J, Mancini M, Li G, et al. (1999). Immunization with a plasmid encoding a modified hepatitis B surface antigen carrying the receptor binding site for hepatocytes. Vaccine 17:1711–18
- Jin J, Yang JY, Liu J, et al. (2002). DNA immunization with fusion genes encoding different regions of hepatitis C virus E2 fused to the gene for hepatitis B surface antigen elicited immune responses to both HCV and HBV. World J Gastroenterol 8:505–10
- Kapila J, De Rycke R, Van Montagu M, Angenon G. (1997). An Agrobacterium-mediated transient gene expression system for intact leaves. Plant Sci 122:101–8
- Komarova TV, Baschieri S, Donini M, et al. (2010). Transient expression systems for plant-derived biopharmaceuticals. Expert Rev Vaccines 9:859–76
- Komarova TV, Kosorukov VS, Frolova OY, et al. (2011). Plant-made trastuzumab (herceptin) inhibits HER2/Neu + cell proliferation and retards tumor growth. PLoS One 6:e17541
- Komarova TV, Schwartz AM, Makarov AA, Dorokhov YL. (2012). A new viral vector exploiting RNA polymerase I-mediated transcription. Biochemistry (Mosc) 77:532–8
- Kozak M. (1989). The scanning model for translation: An update. J Cell Biol 108:229–41
- Lacorte C, Lohuis H, Goldbach R, Prins M. (2007). Assessing the expression of chicken anemia virus proteins in plants. Virus Res 129:80–6
- Lacorte C, Ribeiro SG, Lohuis D, et al. (2010). Potato virus X and Tobacco mosaic virus-based vectors compatible with the Gateway™ cloning system. J Virol Methods 164:7–13
- Lai H, Chen Q. (2012). Bioprocessing of plant-derived virus-like particles of Norwalk virus capsid protein under current Good Manufacture Practice regulations. Plant Cell Rep 31:573–84
- Love AJ, Chapman SN, Matic S, et al. (2012). In planta production of a candidate vaccine against bovine papillomavirus type 1. Planta 236:1305–13
- Lütcke HA, Chow KC, Mickel FS, et al. (1987). Selection of AUG initiation codons differs in plants and animals. EMBO J 6:43–8
- Madesis P, Osathanunkul M, Georgopoulou U, et al. (2010). A hepatitis C virus core polypeptide expressed in chloroplasts detects anti-core antibodies in infected human sera. J Biotechnol 145:377–86
- Memari HR, Ramanan RN, Ariff AB. (2010). Comparison of expression systems for the production of human interferon-α2b. Cent Eur J Biol 5:446–55
- Memarnejadian A, Roohvand F. (2010). Fusion of HBsAg and prime/boosting augment Th1 and CTL responses to HCV polytope DNA vaccine. Cell Immunol 261:93–8
- Memarnejadian A, Roohvand F, Arashkia A, et al. (2009). Polytope DNA vaccine development against hepatitis C virus: A streamlined approach from in silico design to in vitro and primary in vivo analyses in BALB/c mice. Protein Pept Lett 16:842–50
- Mett V, Musiychuk K, Bi H, et al. (2008). A plant-produced influenza subunit vaccine protects ferrets against virus challenge. Influenza Other Respir Viruses 2:33–40
- Meyers A, Chakauya E, Shephard E, et al. (2008). Expression of HIV-1 antigens in plants as potential subunit vaccines. BMC Biotechnol 8:53
- Mishra S, Losikoff PT, Self AA, et al. (2014). Peptide-pulsed dendritic cells induce the hepatitis C viral epitope-specific responses of naïve human T cells. Vaccine 32:3285–92
- Natilla A, Piazzolla G, Nuzzaci M, et al. (2004). Cucumber mosaic virus as carrier of a hepatitis C virus-derived epitope. Arch Virol 149:137–54
- Nemchinov LG, Liang TJ, Rifaat MM, et al. (2000). Development of a plant-derived subunit vaccine candidate against hepatitis C virus. Arch Virol 145:2557–73
- Nianiou I, Kalantidis K, Madesis P, et al. (2008). Expression of an HCV core antigen coding gene in tobacco (N. tabacum L.). Prep Biochem Biotechnol 38:411–21
- Nuzzaci M, Piazzolla G, Vitti A, et al. (2007). Cucumber mosaic virus as a presentation system for a double hepatitis C virus-derived epitope. Arch Virol 152:915–28
- Ohadi M, Rasouli R, Darzi-Eslam E, et al. (2013). Expression of Shigella flexneri ipaB gene in tobacco. Avicenna J Med Biotechnol 5:118–24
- Peach C, Velten J. (1991). Transgene expression variability (position effect) of CAT and GUS reporter genes driven by linked divergent T-DNA promoters. Plant Mol Biol 17:49–60
- Perlak FJ, Fuchs RL, Dean DA, et al. (1991). Modification of the coding sequence enhances plant expression of insect control protein genes. Proc Natl Acad Sci USA 88:3324–8
- Piazzolla G, Nuzzaci M, Tortorella C, et al. (2005). Immunogenic properties of a chimeric plant virus expressing a hepatitis C virus (HCV)-derived epitope: New prospects for an HCV vaccine. J Clin Immunol 25:142–52
- Pniewski T. (2013). The twenty-year story of a plant-based vaccine against hepatitis B: Stagnation or promising prospects? Int J Mol Sci 14:1978–98
- Roohvand F, Kossari N. (2011). Advances in hepatitis C virus vaccines. Part one: Advances in basic knowledge for hepatitis C virus vaccine design. Expert Opin Ther Pat 21:1811–30
- Roohvand F, Kossari N. (2012). Advances in hepatitis C virus vaccines, part two: Advances in hepatitis C virus vaccine formulations and modalities. Expert Opin Ther Pat 22:391–415
- Sambrook J, Russell DW. (2001). Molecular Cloning: A Laboratory Manual, Vol. 1–3. Cold Spring Harbor, New York: Cold Spring Harbor Laboratory Press
- Santi L, Huang Z, Mason H. (2006). Virus-like particles production in green plants. Methods 40:66–76
- Scholthof HB. (2006). The Tombusvirus-encoded P19: From irrelevance to elegance. Nat Rev Microbiol 4:405–11
- Sharp PM, Li WH. (1987). The codon Adaptation Index – A measure of directional synonymous codon usage bias, and its potential applications. Nucleic Acids Res 15:1281–95
- Shoji Y, Farrance CE, Bautista J, et al. (2012). A plant-based system for rapid production of influenza vaccine antigens. Influenza Other Respir Viruses 6:204–10
- Suhrbier A. (2002). Polytope vaccines for the codelivery of multiple CD8 T-cell epitopes. Expert Rev Vaccines 1:207–13
- Towbin H, Staehelin T, Gordon J. (1979). Electrophoretic transfer of proteins from polyacrylamide gels to nitrocellulose sheets: Procedure and some applications. Proc Natl Acad Sci USA 76:4350–4
- Tremblay R, Wang D, Jevnikar AM, Ma S. (2010). Tobacco, a highly efficient green bioreactor for production of therapeutic proteins. Biotechnol Adv 28:214–21
- Uhde-Holzem K, Schlösser V, Viazov S, Fischer R. (2010). Commandeur U. Immunogenic properties of chimeric potato virus X particles displaying the hepatitis C virus hypervariable region I peptide R9. J Virol Methods 166:12–20
- Voinnet O, Rivas S, Mestre P, Baulcombe D. (2003). An enhanced transient expression system in plants based on suppression of gene silencing by the p19 protein of tomato bushy stunt virus. Plant J 33:949–56
- Zheng N, Xia R, Yang C, et al. (2009). Boosted expression of the SARS-CoV nucleocapsid protein in tobacco and its immunogenicity in mice. Vaccine 27:5001–7