Abstract
The study presents the discovery of novel butyrylcholinesterase (BuChE) inhibitors among derivatives of azaphenothiazines by application of in silico and in vitro screening methods. From an in-house library of compounds, 143 heterocyclic molecules derived from the azaphenothiazine scaffold were chosen for virtual screening. Based on results of the docking procedure, 15 compounds were identified as exhibiting the best fit for the two screening complexes (ligand – AChE and ligand – BuChE). Five compounds displayed moderate AChE and good BuChE inhibitory activity at screening concentrations of 10 µM. The IC50 values for active BuChE inhibitors were in the 11.8–122.2 nM range. Three of the most active inhibitors are tetra- or pentacyclic derivatives of azaphenothiazines with the same N-methyl-2-piperidinethyl substituent.
Introduction
Chemical modifications of structures of known pharmaceutical agents are a well-established approach to drug discovery. The tricyclic nitrogen- and sulfur-containing scaffold known as phenothiazine is a potent pharmacophoric moiety; thus phenothiazines are found among several important classes of drugs which possess neuroleptic, antiemetic and anti-histaminic properties. Phenothiazine derivatives exhibit wide-ranging biological activity – they are variously described as antipyretic, analgesic, anticonvulsant, anti-cancer, anti-bacterial, anti-fungal, anti-inflammatory, anti-malarial and immunosuppressive agents. Progress in researching the properties of phenothiazine derivatives has recently been reviewedCitation1,Citation2. Moreover, phenothiazine-like compounds possess potential anti-Alzheimer’s properties, due to their inhibitory potency against acetyl- and butyrylcholinesteraseCitation3,Citation4, neuroprotective activityCitation5,Citation6 as well as the ability to inhibit formation of amyloid beta (Aβ) fibrils and to inhibit the aggregation of tau proteinCitation7. Such properties of bioactive molecules are important from the point of view of neurodegeneration processes and dementia.
Various phenothiazine derivatives have been reported to inhibit cholinesterasesCitation8,Citation9 – among them neuroleptics such as chlorpromazine, thioridazine, derivatives of N-acetylaminophenothiazines, alkylamide and arylamide derivatives of phenothiazineCitation10,Citation11. Generally, most of these compounds are selective inhibitors of butyrylcholinesteraseCitation12. Structures of selected derivatives of phenothiazine which inhibit cholinesterases are presented in . It is interesting to note that the methylthioninium chloride (MTC) compound also known as methylene blue – an old antiseptic dye and a representative of phenothiazines – is currently in phase II clinical trials as potential drug candidate in the treatment of Alzheimer’s disease (AD). This compound has been shown to display multifunctional properties, including influence on the formation of β-amyloid plaques and neurofibrillary tangles, on mitochondrial function and on cholinergic, serotoninergic and glutamatergic neurotransmitter systemsCitation13,Citation14.
Figure 1. Structures of phenothiazine derivatives: neuroleptic drugs, MTC and other selective BuChE inhibitors.
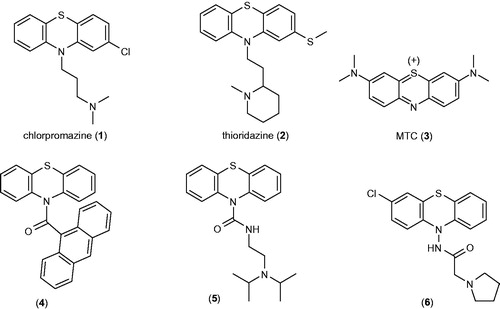
AD is a multifactorial neurodegenerative disorder which is classically characterized by extracellular accumulation of senile plaques composed of Aβ along with intracellular accumulation of the tau protein. AD is complex and still not completely understood as many different processes are involved in neuropathological changes leading to neuronal death. In addition, there is a body of evidence establishing the role of neurotransmitter systems, oxidative stress, metal ion dysregulation and inflammation in AD pathogenesisCitation15. In particular, loss of cholinergic neurotransmission in the brain contributes to the substantial cognitive and behavioral symptoms of ADCitation16. Most drugs currently available on the market for symptomatic treatment of AD are cholinesterase inhibitors such as donepezil, rivastigmine and galantamineCitation17. In healthy brain tissue, cholinesterases are involved in cholinergic neurotransmission by hydrolysis of acetylcholine (ACh), although at different rates: AChE is the main enzyme responsible for ACh hydrolysis, while BuChE plays a secondary (supportive) roleCitation18. As AD progresses, the balance of AChE and BuChE changes. In the AD brain, BuChE level in regions of the brain involved in cognition and behavior is significantly elevated while AChE level in these areas is decreased. Several in vivo experiments indicated that BuChE is able to compensate for the lack of AChE, enabling continued regulation of cholinergic neurotransmissionCitation19,Citation20. It has also been shown that cholinesterases display several non-classical properties associated with Aβ and neurofibrillary tangles, and are therefore important for AD pathogenesisCitation21–25. Recent studies have shown that BuChE exerts an influence on the modulation of motor control, awareness, cognition and behavior by regulation of ACh levels in the central nervous system (CNS)Citation26.
Both enzymes remain valuable targets in anti-AD drug discovery studiesCitation27–30. Many selective or dual acting inhibitors of both enzymes were developedCitation31,Citation32, and among them compounds which display activity also on Aβ aggregation and neurofibrillary tanglesCitation33–37.
Our research focuses on novel cholinesterase inhibitors with additional properties as potential multifunctional anti-Alzheimer’s drugsCitation38–40. The aim of the presented study has been the discovery of novel cholinesterase inhibitors among derivatives of azaphenothiazines by application of in silico and in vitro screening methods.
Methods
Chemistry
Synthesis of tested azaphenothiazines (7–21) was described earlierCitation41–44. Their structure and purity has been confirmed by the NMR spectra which were recorded on Bruker Fourier 300 spectrometers (1H at 500 MHz, 13C at 125 and 75 MHz) in CDCl3 or DMSO-d6 and fast atom bombardment mass spectra which were run on a Finnigan MAT 95 spectrometer at 70 eV. These data were presented for azaphenothiazines (7–9, 14, 17, 20)Citation41 for 21Citation42 and for remaining compounds were described elsewhereCitation43,Citation44.
Molecular modeling
Three-dimensional structures of potential cholinesterases inhibitors (143 ligands) were properly prepared and optimized for processing. In order to prepare compounds for docking with an appropriate geometry the CORINA (Molecular Networks) online application was used to create three-dimensional structures in the pdb formatCitation45. The resulting compounds were processed using the SYBYL 8.0 (St. Louis, MO) toolCitation46. Types of atoms were checked and corrected with each molecule assigned adequate formal and partial charges. Prepared compounds were stored in the mol2 format. Optimization of protein models used in the screening and docking was performed using GOLD 5.1Citation47. Results of docking were visualized using PyMOLCitation48. Detailed procedure was described and validatedCitation49.
Biological tests
The cholinesterase inhibition assay is based on the use of acetylthiocholine iodide (ATCh), and butyrylthiocholine iodide (BTCh) and 5,5′-dithio-bis (2-nitrobenzoic) acid (DTNB) to generate a yellow chromophore (5-mercapto-2-nitrobenzoic acid)Citation50. Reagents and chemicals – DTNB, ATCh, BTCh, AChE from Electrophorus electricus (425.96 U/mg solid), and BuChE from horse serum (2.5 units/1 mL), were purchased from Sigma-Aldrich (Poznań, Poland). All assays were performed using the Perkin Elmer Lambda 12 device. Detection was performed at 412 nm. The assay was carried out as per a previously described protocolCitation39,Citation40 using tacrine hydrochloride control. The compounds tested were dissolved in DMSO giving stock solution (100 µM). Each compound was tested at concentration 10 µM which was obtained by dissolving appropriate amount of stock solution in water. Two kinds of assays were conducted: the peak activity of the enzyme was determined by using a blank sample, followed by reference measurements (for tacrine) and measurements for the synthesized compounds. Data from concentration-inhibition experiments were integrated through non-linear regression analysis using the GraphPad Prism program (GraphPad Prism Software Inc. 2005, La Jolla, CA), producing estimates of IC50.
Kinetic studies were performed using Ellman’s method for compound 14. The test was carried out in 0, 10, 25, and 75 nM concentration of 14 for BuChE. Substrate of the reaction, butyrylthiocholine, was used in the following concentrations: (0.05, 0.1, 0.2, 0.3, 0.5 mM). Stock solution of ATC (0.5 mM in a well) was prepared in water and diluted before use. For each concentration of tested compound ATC was used in concentration of 0.05, 0.1, 0.2, 0.3, 0.5 mM in a well. The obtained data were used to create substrate-velocity curves, which were transformed in GraphPad Prism program to Lineweaver–Burk plots ().
Results and discussion
From an in-house library of compounds, 143 heterocyclic molecules derived from the azaphenothiazine scaffold were chosen. All these compounds had previously been synthesized (by Pluta and co-workersCitation41–44,Citation51–53) and their structures are listed in the supplement. Most of these compounds represent 6-substituted derivatives of pentacyclic diquino-1,4-thiazines and substituted derivatives of tetracyclic benzoazaphenothiazines. Their preliminary biological evaluation focused on anti-cancer, anti-proliferative and immunosuppressive properties. Some of the investigated derivatives of azaphenothiazines exhibited significant anti-cancer activity against several types of human cells as well as immunosuppressive activity in human and mouse modelsCitation42–44,Citation52,Citation53. Knowing that various phenothiazines possess inhibitory activity against cholinesterases, derivatives of azaphenothiazines were selected for our study.
In the first step, virtual screening was performed for all molecules (143 azaphenothiazines). For this molecular modelling study, we applied our custom method which has recently been described and validatedCitation49. In order to accurately check for possible interactions between enzymes with different conformations and test substances, two types of complexes with acetylcholinesterase inhibitors were used: 1ACJ – enzyme with tacrine and 2CKM – enzyme with bis-tacrineCitation54. These complexes were chosen due to possible similarity of interaction between tricyclic tacrine or bis-tacrine and our tetra- or pentacyclic azaphenothiazines. In the search for potential inhibitors of both enzymes (AChE and/or BuChE), our in silico experiments focused on complexes with AChE since it is known that inhibitors of AChE have to meet stricter structural requirements then those of BuChE. Details of the target preparation and docking settings are presented in . Based on results from the docking procedure, 15 compounds were chosen for the in vitro screening. Among 32 pentacyclic azaphenothiazines 5 compounds (7–9, 17, 20), and from the remaining tetracyclic azaphenothiazines 7 compounds (10–13, 16, 18, 19, 21) were identified as exhibiting the best fit for the two complexes used in screening and containing different substituents. Values of the evaluation function for these selected set of compounds were in the 83.05–88.15 and 82.98–86.84 range for the 1ACJ-AChE complex with tacrine, while 82.08–102.53 and 73.47–89.24 for the 2CKM-AChE complex with bis-tacrine, respectively. Moreover, 3 compounds (14–16) with lower values of the scoring function (70.07, 69.06, 66.63 for the 1ACJ-AChE complex with tacrine and 63.35, 61.60, 59.24 for the 2CKM-AChE complex with bis-tacrine, respectively) but with N-methylpiperidine substituent as important pharmacophoric moiety were selected. Values of the scoring function for reference compounds (complex with 2CKM) were 88.45 and 99.67 for tacrine and bis-tacrine, respectively.
Table 1. Details of the target preparation and docking settings for the selected complexes of inhibitors with AChE.
In the next step, the inhibitory potency of the selected compounds against AChE (from electric eel) and BuChE (from horse serum) was evaluated via the spectrophotometric Ellman’s methodCitation40. Experiments were carried out at 10 µM concentration of potential inhibitors. The measured activity is given as a percentage of enzyme inhibition and as IC50 values which were only determined for compounds with better than 50% inhibitory activity at 10 µM concentration. Structures of tested compounds and results of in vitro screening against cholinesterases are collected in . Ten compounds displayed weak AChE (1.41–7.01%) and weak BuChE (0.88–7.30%) inhibitory activity while five compounds showed moderate AChE (19.46–38.75%) and good BuChE (79.99–99.88%) inhibitory activity at 10 µM screening concentration. The IC50 values for active BuChE inhibitors were in the 11.8–122.2 nM range ( and ). Three of the most active inhibitors (14–16) are derivatives of tetra- or pentacyclic azaphenothiazines with the same N-methyl-2-piperidinethyl substituent. The inhibitory activity of their analogs with the 2-chloroethyl-propylurea substituent was significantly lower (17–18).
Table 2. Inhibition of AChEa and BuChEb at 10 µmol/L screening concentration by azaphenothiazine derivatives selected by virtual in silico screening.
Table 3. Physicochemical properties of selected, potential cholinesterases inhibitors: MW, Log P (average Log P from ALOGPS 2.1), molecular volume (Mvol from MarvinSketch).
The mechanism of BuChE inhibition was investigated in enzyme kinetic studies using Ellman’s test and compound 14, the most potent inhibitor. Enzyme kinetic was analyzed by recording substrate-velocity curve in the absence and presence of compound 14. Graphical analysis of Lineweaver–Burk plots of BuChE activity () showed different Km and constant Vmax value in different inhibitor concentrations. This suggest a competitive inhibition, revealing that this compound compete for the same binding site (CAS) as the substrate ACh.
Figure 3. Lineweaver–Burk plots resulting from substrate-velocity curves of BuChE activity of compound 14 in concentration of 10, 25 and 75 nM.
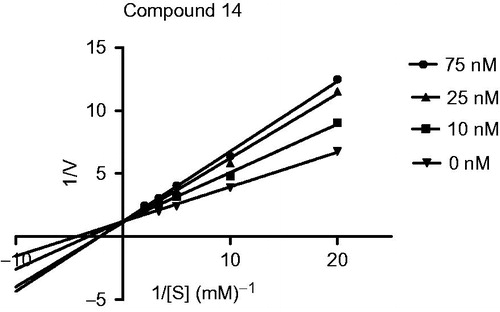
Molecular docking studies showed that ligands, despite structural similarity, were characterized by large variations in their binding mode with BuChE. For compounds with the N-methyl-piperidine substituent (14–16), the penta- or tetracyclic fragment occupies a large cavity in the active site of the enzyme, stacking along the gorge, while the piperidine substituent is oriented toward Trp82 or outside, in the direction of the peripheral binding site. Compounds 17 and 18 in which the 2-chloroethyl-propylurea substituent is much longer enable these azaphenothiazines to reach the bottom of the active site; however, their hydrophobic interactions are weaker. The most active compound 14 represents a large and strongly hydrophobic pentacyclic ring system, which is placed along the gorge of the enzyme between Trp82 and Ile442 at the bottom of the gorge, and Tyr332 near the entrance to the active site. The parallel arrangement of the aromatic rings of the ligand with Trp82 can create hydrophobic interactions and π–π stacking. The more hydrophilic N-methyl piperidine substituent of the ligand is oriented toward the catalytic triad and its arrangement enables interaction with the Ser198 residue. The protonated piperidine nitrogen atom creates cation-π interactions with Trp231 ().
Figure 4. Binding mode of compound 14 in the active site of butyrylcholinesterase. Marked spheres (yellow) show hydrophobic interactions while the arrow (green) indicates a hydrogen bond between the nitrogen atom and the serine 198 amino acid.
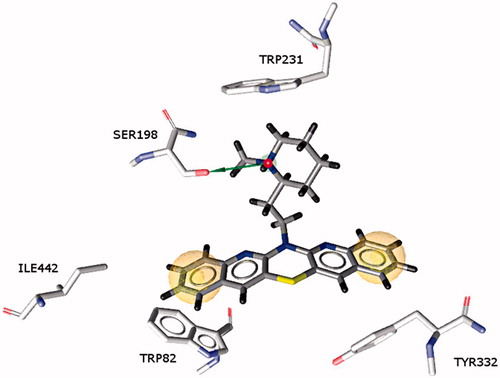
Derivatives of diazatetracene (15 and 16) which were less active than the pentacyclic ligand 14 generally display similar interactions within the active site of the enzyme; however, some differences have been observed (). Their arrangement also differs from that of compound 14. The smaller aromatic fragment creates weaker hydrophobic interactions with Trp82, which explains the lower activity of these compounds. In 15 the thiomethyl substituent, which is larger than a chlorine atom, may enter into hydrophobic interactions with the methyl group of Thr120 close to the entrance to the gorge.
Figure 5. Binding mode of compounds 15 and 16 in the active site of butyrylcholinesterase. Marked spheres (yellow) show hydrophobic interactions. (a) Compound 15 and (b) Compound 16.
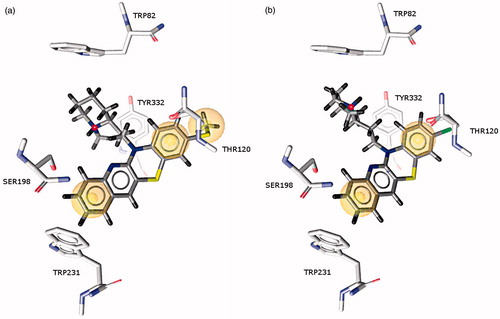
For all studied compounds physicochemical properties such as molecular weight (MW), lipophilicity (logarithm of partition coefficient, log P) and molecular volume (Mvol) were calculated. Data are collected in the Supplement and for tested inhibitors 7–21 in . MW for active inhibitors (14–18) was lower than that given by the rule of five (<500; 410.02–463.03), however log P values were in the 5.22–6.35 range, which indicated high lipophilicityCitation55. Such properties of compounds probably enable them to penetrate the blood–brain barrier and reach the CNSCitation56, however from the point of view of pharmacokinetics they should still be optimized. The calculated molecular volumes for active inhibitors (14–18) were in the 365.99–391.53 Å3 range. These values are in accordance with the results of biological tests and may explain selectivity toward BuChE. Both enzymes differ in terms of the calculated volume of the active site gorge; for AChE the corresponding volume is rather small (320 Å3), while for BuChE it is substantially larger (502 Å3)Citation9.
Conclusions
In conclusion, the role of BuChE as a therapeutic target in the treatment of AD remains a focus of pharmacological and clinical studiesCitation57. This paper reports the discovery of novel selective BuChE inhibitors among azaphenothiazine derivatives by application of virtual screening and in vitro assays. This led to identification of three BuChE inhibitors, derivatives of tetra- or pentacyclic azaphenotiazines. Molecular modeling studies proved the essential role of the N-methyl-2-piperidinethyl substituent in these azaphenothiazines regarding interactions at the active site of BuChE. These studies indicated that results of virtual screening (value of scoring function) were useful for identification of inhibitors while with a wide range of activity (from weak to strong). However, important were also results of analysis of binding mode of ligands in the active site of enzyme. The inhibitory potency of the most active compound 14 (IC50 = 12 nM) was comparable to that of reference tacrine (IC50 = 5 nM). Such activity against BuChE has previously been described for derivatives of phenothiazines. Our results present previously unacknowledged inhibitory activity against BuChE by tetra- or pentacyclic azaphenothiazies.
Supplementary material available online
Supplementary Table.
Supplementary Material
Download PDF (85 KB)Declaration of interest
This work was supported by the Jagiellonian University Collegium Medicum Student’s Grant no. gs 1/7/2011. The authors have declared no conflict of interest.
References
- Pluta K, Morak-Młodawska B, Jeleń M. Recent progress in biological activities of synthesized phenothizines. Eur J Med Chem 2011;46:3179–89
- Jaszczyszyn A, Gąsiorowski K, Świątek P, et al. Chemical structure of phenothiazines and their biological activity. Pharmacol Rep 2012;64:16–23
- Küçükkilinç T, Özer I. Multi-site inhibition of human plasma cholinesterase by cationic phenoxazine and phenothiazine dyes. Archiv Biochem Biophys 2007;461:294–8
- Darvesh S, Pottie IR, Darvesh KV, et al. Differential binding of phenothiazine urea derivatives to wild-type human cholinesterases and butyrylcholinesterase mutants. Bioorg Med Chem 2010;18:2332–44
- González-Muñoz GC, Arce MP, López B, et al. Old phenothiazine and dibenzothiadiazepine derivatives for tomorrow’s neuroprotective therapies against neurodegenerative diseases. Eur J Med Chem 2010;45:6152–8
- González-Muñoz GC, Arce MP, López B, et al. N-acylaminophenothiazines: neuroprotective agents displaying multifunctional activities for a potential treatment of Alzheimer’s disease. Eur J Med Chem 2011;46:2224–35
- Taniguchi S, Suzuki N, Masuda M, et al. Inhibition of heparin-induced tau filament formation by phenothiazines, polyphenols, and porphyrins. J Biolog Chem 2005;280:7614–23
- Radič Z, Pickering NA, Vellom DC, et al. Three distinct domains in the cholinesterase molecule confer selectivity for acetyl- and butyrylcholinesterase inhibitors. Biochemistry 1993;32:12074–84
- Saxena A, Redman AMG, Jiang X, et al. Differences in active site gorge dimensions of cholinesterases revealed by binding of inhibitors to human butyrylcholinesterase. Biochemistry 1997;36:14642–51
- Darvesh S, McDonald RS, Penwell A, et al. Structure-activity relationship for inhibition of human cholinesterases by alkyl amide phenothiazine derivatives. Bioorg Med Chem 2005;13:211–22
- Darvesh S, McDonald RS, Darvesh KV, et al. Selective reversible inhibition of human butyrylcholinesterase by aryl amide derivatives of phenothiazine. Bioorg Med Chem 2007;15:6367–78
- Debord J, Merle L, Bollinger JC, Dantoine T. Inhibition of butyrylcholinesterase by phenothiazine derivatives. J Enzyme Inhib Med Chem 2002;17:197–202
- Oz M, Lorke DE, Petroianu GA. Methylene blue and Alzheimer’s disease. Biochem Pharmacol 2009;78:927–32
- Oz M, Lorke DE, Hasan M, Petroianu GA. Cellular and molecular actions of Methylene Blue in the nervous system. Med Res Rev 2011;31:93–117
- Querfurth HW, LaFerla FM. Alzheimer’s disease. N Engl J Med 2010;362:329–44
- Davies P, Maloney JF. Selective loss of central cholinergic neurons in Alzheimer’s disease. Lancet 1976;2:1403
- Rodda J, Carter J. Cholinesterase inhibitors and memantine for symptomatic treatment of dementia. BMJ 2012;344:e2986
- Darvesh S, Hopkins DA, Geula C. Neurobiology of butyrylcholinesterase. Nat Rev Neurosci 2003;4:131–8
- Li B, Stribley JA, Ticu A, et al. Abundant tissue butyrylcholinesterase and its possible function in the acetylcholinesterase knockout mouse. J Neurochem 2000;75:1320–31
- Mesulam MM, Guillozet A, Shaw P, et al. Acetylcholinesterase knockouts establish central cholinergic pathways and can use butyrylcholinesterase to hydrolyze acetylcholine. Neuroscience 2002;110:627–39
- Bartolini M, Bertucci C, Cavrini V, Andrisano V. β-Amyloid aggregation induced by human acetylcholinesterase: inhibition studies. Biochem Pharmacol 2003;65:407–16
- Diamant S, Podoly E, Friedler A, et al. Butyrylcholinesterase attenuates amyloid fibril formation in vitro. Proc Natl Acad Sci USA 2006;103:8628–33
- Podoly E, Shalev DE, Shenhar-Tsarfaty S, et al. The Butyrylcholinesterase K variant confers structurally derived risks for Alzheimer pathology. J Biol Chem 2009;284:17170–9
- Darreh-Shori T, Soininen H. Effects of cholinesterase inhibitors on the activities and protein levels of cholinesterases in the cerebrospinal fluid of patients with Alzheimer’s disease: a review of recent clinical studies. Curr Alzh Res 2010;7:67–73
- Dinamarca MC, Sagal JP, Quintanilla RA, et al. Amyloid-β-Acetylcholinesterase complexes potentiate neurodegenerative changes induced by the Aβ peptide. Implications for the pathogenesis of Alzheimer’s disease. Mol Neurodegener 2010;5: 4. doi: 10.1186/1750-1326-5-4
- Reid GA, Chilukuri N, Darvesh S. Butyrylcholinesterase and the cholinergic system. Neuroscience 2013;234:53–68
- Giacobini E. Cholinesterase inhibitors: new roles and therapeutic alternatives. Pharmacol Res 2004;50:433–40
- Greig NH, Utsuki T, Ingram DK, et al. Selective butyrylcholinesterase inhibition elevates brain acetylcholine, augments learning and lowers Alzheimer β-amyloid peptide in rodent. Proc Natl Acad Sci USA 2005;102:17213–18
- Lane RM, Potkin SG, Enz A. Targeting acetylcholinesterase and butyrylcholinesterase in dementia. Int J Neuropsychoph 2006;9:101–24
- Pepeu G, Giovannini MG. Cholinesterase inhibitors and beyond. Curr Alzh Res 2009;6:86–96
- Musiał A, Bajda M, Malawska B. Recent developments in cholinesterases inhibitors for Alzheimer’s disease treatment. Curr Med Chem 2007;14:2654–79
- Singh M, Kaur M, Kukreja H, et al. Acetylcholinesterase inhibitors as Alzheimer therapy: from nerve toxins to neuroprotection. Eur J Med Chem 2013;70:165–88
- Viayna E, Sabate R, Muñoz-Torrero D. Dual inhiitors of β-amyloid aggregation and acetylcholinesterase as multi-target anti-Alzheimer drug candidates. Curr Top Med Chem 2013;13:1820–42
- Rizzo S, Tarozzi A, Bartolini M, et al. 2-Arylbenzofuran-based molecules as multipotent Alzheimer’s disease modifying agents. Eur J Med Chem 2012;58:519–32
- Carolan CG, Dillon GP, Khan D, et al. Isosorbide-2-benzyl carbamate-5-salicylate, a peripheral anionic site binding subnanomolar selective butyrylcholinesterase inhibitor. J Med Chem 2010;53:1190–9
- Yan JW, Li YP, Ye WJ, et al. Design, synthesis and evaluation of isaindigotone derivatives as dual inhibitors for acetylcholinesterase and amyloid beta aggregation. Bioorg Med Chem 2012;20:2527–34
- Silva D, Chioua M, Samadi A, et al. Synthesis, pharmacological assessment, and molecular modeling of acetylcholinesterase/butyrylcholinesterase inhibitors: effect against amyloid-β-Induced neurotoxicity. ACS Chem Neurosci 2013;4:547–65
- Bajda M, Ignasik M, Guzior N, Malawska B. Multi-target-directed ligands in Alzheimer’s disease treatment. Curr Med Chem 2011;18:4949–75
- Bajda M, Kuder K, Łażewska D, et al. Dual-acting diether derivatives of piperidine and homopiperidine with histamine H3 receptor antagonistic and anticholinesterase activity. Archiv Pharm – Chem Life Sci 2012;345:591–7
- Ignasik M, Bajda M, Guzior N, et al. Design, synthesis and evaluation of novel 2-(aminoalkyl)-isoindoline-1,3-dione derivatives as dual-binding site acetylcholinesterase inhibitors. Archiv Pharm – Chem Life Sci 2012;345:509–16
- Jeleń M, Pluta K. Synthesis of 6-aminoalkyldiquinithiazines and their acyl and sulfonyl derivatives. Heterocycles 2008;75:859–70
- Jeleń M, Pluta K. Synthesis of quinobenzo-1,4-thiazines from diquino-1,4-dithiin and 2,2′-dichloro-3,3′-diquinolinyl disulfide. Heterocycles 2009;78:2325–36
- Pluta K, Jeleń M, Zimecki M, et al. New derivatives of quino[3,2-b]benzo[1,4]thiazine, methods of synthesis, application for obtaining medicines and pharmaceutical formulation and their methods of preparation. (polish), Polish Patent Appl P.398835 (16.04.2012)
- Jeleń M, Pluta K, Zimecki M, et al. Synthesis and selected immunological properties of substituted quino[3,2-b]benzo[1,4]thiazines. Eur J Med Chem 2013;63:444–56
- Online demo – Corina. Available from: http://www.molecularnetworks.com/online_demos/corina_demo [last accessed 21 Nov 2013]
- Sybyl 8.0. St. Louis (MO): Tripos; 2007
- Gold Suite 5.1, The Cambridge Crystallographic Data Centre: Cambridge, UK; 2011
- PyMOL 0.99rc6. Palo Alto (CA): DeLano Scientific LLC; 2006
- Bajda M, Więckowska A, Hebda H, et al. Structure-based search for new inhibitors of cholinesterases. Int J Mol Sci 2013;14:5608–32
- Ellman GL, Courtney KD, Andres V, Feather-Stone RM. A new and rapid colorimetric determination of acetylcholinesterase activity. Biochem Pharmacol 1961;7:88–90
- Nowak M, Pluta K, Suwińska K, Straver L. Synthesis of new pentacyclic diquinothiazines. J Heterocycl Chem 2007;44:543–50
- Zimecki M, Artym J, Kocięba M, et al. The immunosuppressive activities of newly synthesized azaphenothiazines in human and mouse models. Cell Mol Biol Lett 2009;14:622–35
- Pluta K, Jeleń M, Morak-Młodawska B, et al. Anticancer activity of newly synthesized azaphenothiazines from NCI’s anticancer screening bank. Pharmacol Rep 2010;62:319–32
- Protein Data Bank. Available from: http://www.pdb.org [last accessed 21 Nov 2013]
- Lipinski CA, Lombardo F, Dominy BW, Feeney P. Experimental and computational approaches to estimate solubility and permeability in drug discovery and development settings. J Adv Drug Deliv Rev 1997;23:3–25
- Pajouhesh H, Lenz GR. Medicinal chemical properties of successful central nervous system drugs. NeuroRx 2005;2:541–53
- Nordberg A, Ballard C, Bullock R, et al. A review of butyrylcholinesterase as a therapeutic target in the treatment of Alzheimer’s disease. Prim Care Companion CNS Disord 2013;15.doi: 10.4088/PCC.12r01412