Abstract
Tungstate has been identified as a ground water contaminant at military firing ranges and can be absorbed by ingestion. In this study, C57BL6 mice were exposed to sodium tungstate (Na2WO4·2H2O) (0, 2, 62.5, 125, and 200 mg/kg/day) in their drinking water for an initial 28-day screen and in a one-generation (one-gen) model. Twenty-four hours prior to euthanasia, mice were intraperitoneally injected with Staphylococcal enterotoxin B (SEB) (20 μg/mouse) or saline as controls. After euthanasia, splenocytes and blood were collected and stained with lymphocyte and/or myeloid immunophenotyping panels and analyzed by flow cytometry. In the 28-day and one-gen exposure, statistically significant reductions were observed in the quantities of activated cytotoxic T-cells (TCTL; CD3+CD8+CD71+) and helper T-cells (TH; CD3+CD4+CD71+) from spleens of SEB-treated mice. In the 28-day exposures, CD71+ TCTL cells were 12.87 ± 2.05% (SE) in the 0 tungstate (control) group compared to 4.44 ± 1.42% in the 200 mg/kg/day (p < 0.001) group. TH cells were 4.85 ± 1.23% in controls and 2.76 ± 0.51% in the 200 mg/kg/day (p < 0.003) group. In the one-gen exposures, TCTL cells were 7.98 ± 0.49% and 6.33 ± 0.49% for P and F1 mice after 0 mg/kg/day tungstate vs 1.58 ± 0.23% and 2.52 ± 0.25% after 200 mg/kg/day of tungstate (p < 0.001). Similarly, TH cells were reduced to 6.21 ± 0.39% and 7.20 ± 0.76%, respectively, for the 0 mg/kg/day P and F1 mice, and 2.28 ± 0.41% and 2.85 ± 0.53%, respectively, for the 200 mg/kg/day tungstate P and F1 groups (p < 0.001). In delayed-type hypersensitivity Type IV experiments, tungstate exposure prior to primary and secondary antigen challenge significantly reduced footpad swelling at 20 and 200 mg/kg/day. These data indicate that exposure to tungstate can result in immune suppression that may, in turn, reduce host defense against pathogens.
Introduction
The consequences of exposure to soluble forms of tungsten have only recently undergone examination. Sources of tungsten, either naturally occurring or due to spent tungsten alloy ammunition at military firing ranges, can be solubilized by water (e.g. rainwater) into tungstate (), enter ground water systems, and be ingested (Clausen & Korte, Citation2009). The effects of exposure to tungsten and its alloys are of interest to the US military primarily because of its use in munitions. The switch from lead and depleted uranium to tungsten during the late 1990s occurred in part because it was thought that tungsten was an inert heavy metal and, therefore, environmentally and biologically safe, in contrast to lead and depleted uranium with their well-known environmental and health concerns (Briner, Citation2006, Citation2010; Clausen & Korte, Citation2009).
The lack of toxicological information regarding tungsten became evident following the recognition of an acute lymphoblastic leukemia (ALL) cluster in Churchill County, Nevada (Rubin et al., Citation2007; Steinberg et al., Citation2007), which initial reports suggested was attributed to high levels of tungstate in ground and drinking water sources. Significant geologic deposits of tungsten and tungsten mining operations are present in Churchill County and nearby communities. Notably, the causative connection between tungstate exposure and ALL was never definitively confirmed. In a separate study, tungsten alloy (Co/Ni/W) fragments representative of alloy used in military munitions resulted in aggressive rhabdomyosarcomas when implanted into the legs of rats (Kalinich et al., Citation2005). Again, the role of tungsten was unclear and the other metals (i.e., Co and Ni) present in the alloy may have also contributed to tumor initiation. These data and other studies demonstrated the need for a more thorough examination of the toxicological effects of exposure to tungsten and its soluble forms.
Tungstate has been shown to have a number of specific physiological effects in exposed animals and humans. This compound is a competitive inhibitor of molybdenum-containing enzymes such as xanthine oxidase (XO) (Pacher et al., Citation2006), which is found in all tissues and broadly across all species. This enzyme is part of the purine biosynthetic pathway that catalyzes conversion of hypoxanthine to xanthine and finally uric acid. XO is also a well-known producer of reactive oxygen species (ROS) that can contribute to oxidative tissue damage during ischemia reperfusion (Wright et al., Citation2004). In addition, the ROS produced by XO have been shown to contribute to host defenses in microbial infection models (Umezawa et al., Citation1997; Vorbach et al., Citation2003). Tungstate inhibition of XO in mice increased the susceptibility to leukemic outcomes when coupled with respiratory syncytial virus (RSV) exposure (Fastje et al., Citation2011). While the mechanism of the leukemic transformation is unclear, recent data demonstrate that XO-dependent ROS have a central role in antigen presentation and cell maturation of Kupffer cells and other professional antigen-presenting cells (APC) (Maemura et al., Citation2005).
In vitro studies have demonstrated that tungstate and tungsten alloys have a wide range of biological effects. We have previously shown that tungstate exposure increases apoptosis and decreases cellular proliferation of isolated human peripheral blood leukocytes (Osterburg et al., Citation2010). Additionally, cytokine secretion from peripheral blood mononuclear cells (PBMC) induced by lipopolysaccharide (LPS), Concanavalin A (ConA), or CD3/CD28 beads were significantly reduced after tungstate exposure. In hepatocyte cell lines, tungstate exposure changes phosphate-dependent pathway signaling, an effect that is dependent on the specific cell line as well as the species (Johnson et al., Citation2010). In C2C12, SKNMC, and HEK293 cell lines (Verma et al., Citation2011), the carcinogenic potential of tungsten alloys has been explored by examining induced epigenetic changes where tungsten alloy (Co/Ni/W) exposure resulted in histone H3 dephosphorylation; there was evidence of synergism of tungstate with the cobalt and nickel present in the alloy in causing this effect. Human osteoblasts also have been transformed into neoplastic phenotypes when exposed to tungsten alloys (Nishino et al., Citation2008).
In addition, tungstate is a competitive inhibitor of cellular phosphatases due to its structural similarity to phosphate. Increased phosphorylation of intracellular proteins results from binding to the catalytic pocket, thereby blocking the function of cellular phosphatases such as protein phosphatases and protein tyrosine phosphatases (Chen et al., Citation2009; Egloff et al., Citation1995; Rodriguez-Hernandez et al., Citation2012; Yang et al., Citation1998). Altered phosphorylation has been noted both in vitro as well as in vivo. Changes in the phosphorylation of ERK1/2, p38, H1b, and GSK3β, for example, have been reported (Dominguez et al., Citation2003; Piquer et al., Citation2007; Osterburg et al., Citation2010). Taken together, the effects of tungstate on ROS and intracellular signaling suggest that exposure could alter the function of cells of the immune system.
Military families exposed to spent tungsten munitions in the environment and communities near natural tungstate sources are potentially exposed to tungstate, particularly through ground water contamination, and an examination of the effects on immune function is needed. Thus, the objective of this study was to determine if tungstate exposure had detrimental effects on immune function following acute exposures as well as in a one-generation (one-gen) exposure. This study examined the consequences of tungstate exposure on the immune response of mice after activation of the immune system with either Staphylococcal enterotoxin B (SEB) or in a delayed-type hypersensitivity (DTH) Type IV model. The study here investigated the effects of tungstate in a 28-day and a one-gen exposure to determine acute as well as longer-term exposure toxicities.
Materials and methods
Reagents
Sodium tungstate dihydrate (CAS# 10213-10-2) and Staphylococcal enterotoxin B (SEB) were obtained from Sigma (St. Louis, MO). Lipopolysaccharide (LPS, Escherichia coli, Type O111:B4) was purchased from List Biological Laboratories, Inc. (Campbell, CA). Anti-mouse CD3e Alexa 647 (Rat IgG2β), CD4 Alexa 700 (Rat IgG2β), CD8 Pacific Blue (Rat IgG2β), CD19 PE-Cy5 (Rat IgA), CD161c/NK1.1 R-PE (Rat IgG2α), CD71 FITC (Rat IgG2β), CD11b Alexa 647 (Rat IgG2β), and F4/80 Pacific Blue (Rat IgG2α) were procured from eBiosciences (San Diego, CA). Non-specific isotype antibodies Rat IgG2β Alexa-647, Rat IgG2β Alexa 700, Rat IgG2β Pacific Blue, Rat IgG2β FITC, Mouse IgA PE-Cy5, Mouse R-PE IgG2α, Rat IgM FITC, Rat R-PE IgG2α, and Rat IgG2α Pacific Blue were also from eBiosciences. Ly6C FITC (Rat IgM), Ly6G R-PE (Rat IgG2α) and FcBlock were bought from BD Biosciences (Franklin Lakes, NJ). Gr-1 Pacific Orange (Rat IgG2β), isotype control Rat IgG2β Pacific Orange, and non-specific rat serum was purchased from Invitrogen (Carlsbad, CA). ELISA plates were procured from Meso Scale Discovery (Gaithersburg, MD) and/or from eBiosciences. NP-O-Su (4-hydroxy-3-nitrophenylacetic acid active ester) was bought from Biosearch Technologies, Inc. (Novato, CA).
Animal care
C57BL6 (28-day study = 8–12-week-old, 19–22 g; one-gen study = 8–12-week-old, 15–18 g) mice were obtained from Charles River Laboratories (Wilmington, MA). Animals were housed in an Association for Assessment and Accreditation of Laboratory Animal Care (AAALAC) approved facility under animal protocols approved by the Wright-Patterson Air Force Base and/or University of Cincinnati Institutional Animal Care and Use Committee (IACUC). Mice were allowed to acclimatize to the animal facility for 7 days before commencement of the experimental phase. During the course of the experiment, animals had a 12 h day/night cycle in a temperature-controlled room (22 °C). All animals had ad libitum access to filtered water and a low molybdenum diet; CA.170481 AIN-76 A Purified Diet (Harlan Laboratories, Madison, WI).
Individual mice were identified by ear punch. Body weights were recorded weekly and quantities of tungstate adjusted appropriately in water bottles. Water consumption was determined using graduated water bottles. Measurements were made daily of water consumed by mice. The tungstate in the water bottles was administered to calculate approximate ingested doses of tungstate based on an estimated water consumption of 4.5 ml/mouse/day. For the 28-day study, mice were administered 0, 62.5, 125, or 200 mg/kg/day of tungstate for the course (Guandalini et al., Citation2011). In the one-gen study parental mice were dosed with 0, 2, 62.5, 125, or 200 mg/kg/day of tungstate. Both P and F1 mice were maintained on these doses for the course of the study. These quantities of tungstate in the two studies were selected based on previous work that used similar doses in rats (McInturf et al., Citation2011). Water bottles were changed 2–3 times weekly, always using water from the source and a 1 M sodium tungstate stock supply.
In the one-gen exposure, mice were exposed to tungstate for 90 days prior to mating (Weeks 1–12). The next 7 weeks comprised gestation and weaning (Weeks 13–19). After pups (F1) were weaned, the parents (P) were necropsied as described, ≈19 weeks after initiation of the study. The F1 generation was exposed to tungstate for a further 90 days after weaning and then necropsied. Mice were housed singly during the course of the study and pair mated for breeding. After confirmation of pregnancy, males were removed. During all phases of the one-gen study mice were kept on the appropriate tungstate dose.
Delayed-type hypersensitivity
C57BL6 mice were given tungstate orally by including appropriate doses (0, 0.2, 2, 20, 200 mg/kg/day) in their drinking water for 28 days. Experiments were then conducted as described in Current Protocols in Immunology (Luo & Dorf, Citation2001). Briefly, NP-O-Su was solubilized in 2% dimethyl sulfoxide (DMSO, 99%) for administration of 50 µl as a dorsal subcutaneous injection. This was immediately followed by 0.1 ml of borate-buffered saline solution (pH 8.6) to enhance haptenization and primary immune responses. During the sensitization phase, appropriate tungstate doses continued to be administered to the treated mice. Ten days later, the mice were anesthetized with isofluorane (3.5%) for injection of 20 µl of NP-O-Su mixed (1:20) with phosphate-buffered saline (PBS, pH 7.8) into the right hind foot pad with a 28-gauge needle; an equivalent volume of PBS was injected into the contralateral foot. The extent of footpad swelling was measured with a dial gauge 24 h post-injection.
Immune activation and necropsy
Twenty-four hours prior to necropsy, mice were injected intraperitoneally (IP) with either sterile saline or 20 µg of SEB in saline to induce an immune response. Animals were euthanized by CO2 asphyxiation and blood was obtained by cardiac puncture with a 23-gauge needle and placed into Na2EDTA-anti-coagulant-coated tubes. Tissues were harvested according to normal procedures, and the spleens and blood were processed for subsequent flow cytometric staining and analyses. Briefly, the blood and spleens were each placed in ice-cold flow buffer (FB; 1X PBS, 0.1% sodium azide, 0.1 g CaCl2/L, 0.5% BSA [pH 7.4]). Single-cell homogenates were made by grinding each intact spleen between two frosted slides and then passing the mixture through a 70 -µm mesh filter.
Flow cytometry
Flow cytometry was performed as previously described with minor modifications (Babcock & Dawes, Citation1994; Bommireddy et al., Citation2003). Briefly, whole blood or splenocyte single cell suspensions were placed into appropriate tubes and then non-specific antibody binding was reduced by adding 1 µg/tube of FcBlock and rat serum (1:20/tube) and incubating the samples for 30 min on ice. Antibodies were then added and the tubes incubated at room temperature (RT) in the dark. Cells were then washed with FB, pelleted by centrifugation, the supernatant was discarded, and the cell pellets were re-suspended and fixed in a final concentration of 2% paraformaldehyde for 15 min at RT. After fixation, contaminating red blood cells (RBC) were removed by acidic water lysis of the RBC. The cell suspension was restored to tonicity with an addition of an appropriate quantity of 10X PBS, followed by FB. The cells were then pelleted by centrifugation (350 × g, 10 min, 4 °C), washed 2X in FB, and fixed in a final concentration of 1.6% paraformaldehyde. Samples were analyzed on a LSRII flow cytometer (BD Biosciences, San Jose, CA) equipped with 405, 488, and 633 nm lasers and appropriate filters. Data were collected with FACSDiva software (v 5.02, BD Biosciences) and analyzed with FCS Express (v3, De Novo Software, Los Angeles, CA). Non-specific binding was monitored by inclusion of tubes with fluorescent-labeled isotype controls. Discrimination between negative and positive populations was determined by the use of fluorescent-minus one (FMO) controls. At least 100,000 events per tube were collected. The gating strategy used for these samples was to first collect typical immunological cell populations with FSC vs SSC. These events were then subjected to FSC-W vs FSC-A to exclude doublets and aggregates from further analysis.
ELISA
A portion of the blood from each cardiac puncture was centrifuged and the plasma removed and frozen at −20 °C for later analysis by ELISA using either a Meso Scale Discovery system or by standard ELISA (for IL-6, TNFα, IL-10, and IFNγ) procedures in accordance with manufacturer instructions.
Statistics
All statistical tests were performed with Systat (v11 or v13, Systat Software Inc., Chicago, IL). Statistical significance was assumed at p < 0.05. Comparisons between treatments (tungstate dose, immune challenge) were performed as two-way analysis of variance (ANOVA). Differences in weights were determined by repeated measures analysis of variance (RM-ANOVA). When assumptions of normality and/or equal variances were violated, non-parametric Kruskal-Wallis tests were performed. Specific post-hoc tests were performed and multiple test corrections performed when appropriate.
Results
Previous studies have shown that tungstate exposure results in reduced water consumption due to palatability (taste) changes of the water (Johnson et al., Citation1974). To address this, we conducted a preliminary experiment in which we measured water consumption over a 7-day period with a range of doses (62.5, 125, and 200 mg/kg/day) intended for use in subsequent experiments. As is shown in , there were no significant changes in water consumption due to the quantity of tungstate present in the water. Therefore, supplementation of the water with glucose to increase palatability was not needed. Additionally, we used these data to estimate the quantity of water consumed per mouse per day (∼4.5 ml) to formulate initial tungstate doses of 0, 2, 62.5, 125, and 200 mg/kg/day.
Figure 1. Drinking water volumes of C57BL6 mice exposed to tungstate orally. Animal cages included water bottles with graduated markings. Daily water consumption was recorded and water volumes, as well as tungstate concentrations, adjusted as necessary. There were eight mice per group; error bars represent ± standard error (SE).
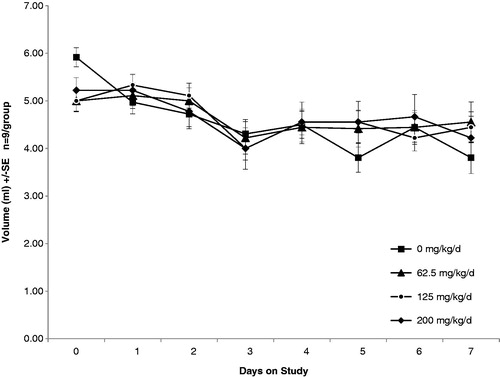
During the course of the 28-day and the one-gen exposures we measured body weights to determine if there were tungstate-dependent changes. In , weights by sex and study are shown. We found no statistically significant changes in body weight due to any tungstate dose levels in the 28-day, one-gen, or delayed-Type IV hypersensitivity experiments (data not shown). The 200 mg/kg/day males in the P generation show a consistent trend towards decreased weights (). This observation, however, was not statistically significant. Additionally, we did not observe statistically significant changes in the number of live births, litter size, or sex ratio at any dose of tungstate tested (data not shown).
Figure 2. Weights of mice exposed to tungstate. Male and female mice were placed in cages and provided various doses of tungstate in their drinking water. Body weights were monitored during the course of exposure. Open symbols, male; closed symbols, female. Doses indicated by Circle = 0 mg/kg/day, square = 2 mg/kg/day, diamond = 62.5 mg/kg/day, X = 125 mg/kg/day, triangle = 200 mg/kg/day. (A) Mice exposed for 28 days. Values shown are mean ± SE of 11–12 mice/timepoint/sex. (B) Parental mice exposed for 12 weeks before mating, followed by gestation, birth, and weaning. Values shown are mean ± SE of 6–8 mice/timepoint/sex. (C) F1 mice (offspring) exposed after weaning for 12 weeks. Values shown is mean ± SE of 6–8 mice/timepoint/sex.
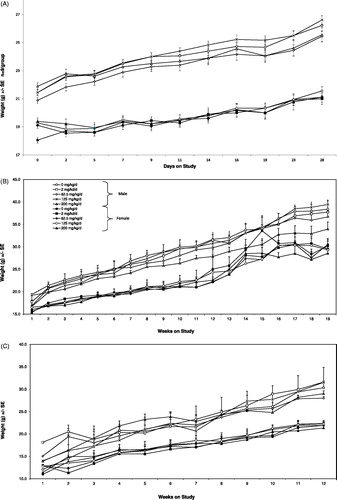
The focus of these studies was to determine changes in immunological populations. Therefore, we performed complete blood counts and measured hematological parameters from the blood of animals at necropsy. With two exceptions (monocyte% and red blood cell distribution width), there were no statistical differences in the data between P and F1; therefore, the data for P and F1 have been combined in . As shown in , there were no significant changes in any of the parameters measured in response to tungstate, with the exception of the percent monocytes (%MO). There were fewer lymphocytes in the F1 generation compared to the P generation (p < 0.023), but this was not dose-related. Additionally, the red blood cell distribution width (RDW) was higher in the P generation vs the F1 pups (p < 0.004). The percentage of monocytes was dose-dependently lower at higher concentrations when compared to control (p < 0.003). Other parameters suggest a dose-dependent trend (e.g. hematocrit); however, these trends were not statistically significant. Because there were no significant alterations in overall quantities of hematological populations we next examined the immune response to SEB of lymphocytes from mice exposed to tungstate.
Figure 3. Complete blood counts and hematological parameters of one-generational exposures. A portion of anti-coagulated whole blood from tungstate exposed mice was analyzed by a Hemavet 950 (Drew Scientific) within 4 h of necropsy. Significant differences between generations were very limited; therefore, CBC data for the P and F1 generations were combined for clarity. All parameters use the left axis, with the exception of PLT. Each bar represents the mean ± SE of 11–12 mice/parameter. White blood cell K/µl (WBC), hemoglobin g/dL (HB), mean corpuscular HB concentration g/dL (MCHC), mean platelet volume fL (MPV), lymphocyte K/µl (LY), monocyte K/µl (MO), neutrophil K/µl (NE), eosinophil K/µl (EO), basophil K/µl (BA), red blood cell count M/µl (RBC), hematocrit % (HCT), mean corpuscular volume fL (MCV), mean corpuscular HB pg (HCB), red cell distribution width % (RDW), percent lymphocyte (%LY), percent monocyte (%MO), percent neutrophil (%NE), percent eosinophil (%EO), percent basophil (%BA), platelets K/µl (PLT).
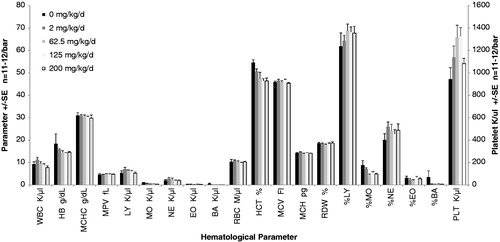
For immunophenotyping studies, 24 h prior to necropsy, SEB (20 µg/mouse) or saline was injected IP. The SEB was used as an activator of the adaptive immune response because of its major histocompatibility complex II (MHC II) and T-cell receptor (TCR) cross-linking properties (Krakauer, Citation2005). At necropsy, splenocytes and blood were harvested and stained with panels of antibodies for analysis by flow cytometry. We have not observed any sex-dependent effects based on the tungstate exposure (data not shown) and therefore grouped male and female results. Some sex-dependent differences in innate immune response were observed (neutrophils [Gr1++CD11b+] and monocytes [Gr1−CD11b+] were different between males and females) (data not shown). However, these were un-related to tungstate exposure. Tungstate-dependent changes in either the 28-day or one-gen exposures were only observed in the spleens of animals. Furthermore, any statistically significant differences between the innate or immune responses of P and F1 mice were not noted.
As shown in , tungstate exposure for 28 days did not result in significant changes in the percentage of helper T-cells (TH; CD3+CD4+). However, the number of activated helper T-cells (CD3+CD4+CD71+), or CD71+ (transferrin receptor) TH cells (, top right) were significantly reduced at the 200 mg/kg/day dose as compared to in the controls (4.85 ± 1.23% for control vs 2.76 ± 0.51% in tungstate group, p < 0.003). Little to no effect on these cells was noted in the controls receiving IP saline. Consistent with these data, the one-gen exposures resulted in reduced quantities of CD71+ TH cells in the parental mice, 6.21 ± 0.39% for the controls and 2.28 ± 0.41% for the 200 mg/kg/day dose group (). The F1 controls in the SEB-treated groups were 7.20 ± 0.76% and 2.85 ± 0.53% for the 200 mg/kg/day tungstate groups (p < 0.001). No statistically significant differences were noted in the overall quantity of CD3+CD4+ TH cells (). Additionally, there were no significant effects of generation on the suppression of CD71+ TH cells in SEB-treated mice exposed to tungstate.
Figure 4. T-Helper (TH) cell quantities and CD71+ TH cells in spleens of mice exposed to tungstate. Mice were exposed to tungstate for either 28 days (A, B) or 12–19 weeks in a one generational (C–E) model. Bars are the mean ± SE of 11–12 mice in the 28-day study and six mice in the one-gen exposure. (A) Quantities of CD3+CD4+ cells in spleens 24 h after challenge with saline or Staphylococcal enterotoxin B (SEB; 20 µg/mouse). (B) Quantities of TH cells that are CD71+ in spleens 24 h after challenge with saline or SEB. (C) Quantities of CD3+CD4+ cells in spleens 24 h after challenge with SEB in parental (P) or first filial (F1) groups. (D) Quantities of CD3+CD4+ and CD71+ cells in spleens 24 h after challenge with saline in P or F1 groups. (E) Quantities of CD3+CD4+ and CD71+ cells in spleens 24 h after challenge with SEB in P or F1 groups. *p < 0.003, **p < 0.001.
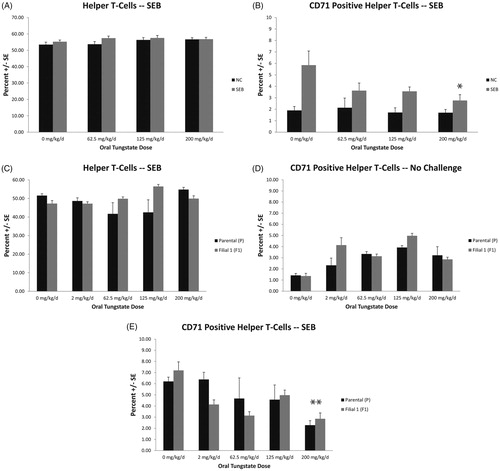
We also observed a significant reduction in the quantity of activated cytotoxic T-cells (CD3+CD8+CD71+) in the spleens of tungstate-treated mice. As with the TH cells, this effect occurred in the high-dose tungstate groups. As shown in there was no effect of the 28-day tungstate treatment on the quantity of cytotoxic T-cells (CD3+CD8+) in the saline controls or in the SEB-challenged mice. The number of CD71+ CD8 cytotoxic T-cells was significantly reduced in the SEB-treated () mice at high tungstate doses. The 0 mg/kg/day dose resulted in 12.87 ± 2.05% CD71+ cytotoxic T-cells vs 4.44 ± 1.42% in the 200 mg/kg/day group (p < 0.001). There were no statistically significant differences in quantities of CD3+CD8+ cells in the one-gen study (, middle left). The cytotoxic CD3+CD8+CD71+ cells () in the parental mice were 7.98 ± 0.49% in the 0 mg/kg/day SEB-challenged groups and 1.58 ± 0.23% in the corresponding 200 mg/kg/day group. The F1 mice were 6.33 ± 0.49% for the controls and 2.52 ± 0.25% in the 200 mg/kg/day tungstate group (p < 0.001).
Figure 5. Cytotoxic T (TCTL) cell quantities and CD71+ TCTL cells in spleens of mice exposed to tungstate. Mice were exposed to tungstate for either 28 days (A, B) or 12–19 weeks in a one generational (C–E) model. Bars are the mean ± SE of 11–12 mice in the 28-day study and six mice in one-gen exposure. (A) Quantities of CD3+CD8+ cells in spleens 24 h after challenge with saline or Staphylococcal enterotoxin B (SEB; 20 µg/mouse). (B) Quantities of TCTL cells that are CD71+ in spleens 24 h after challenge with saline or SEB. (C) Quantities of CD3+CD8+ cells in spleens 24 h after challenge with SEB in parental (P) or first filial (F1) groups. (D) Quantities of CD3+CD8+ and CD71+ cells in spleens 24 h after challenge with saline in P or F1 groups. (E) Quantities of CD3+CD8+ and CD71+ cells in spleens 24 h after challenge with SEB in P or F1 groups. *p < 0.001, **p < 0.001.
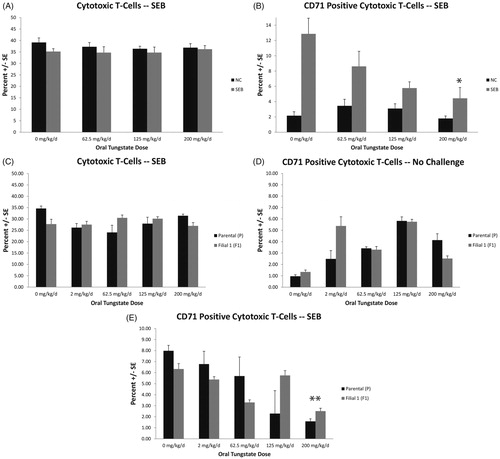
Among cytokines measured in plasma, the only significant change across both studies was a dose-dependent quantitative decrease in interferon (IFN)-γ levels in SEB-treated mice. In the 28-day study () there was a decrease from 6.98 ± 0.77 pg/ml in the control mice to near baseline levels in the high-tungstate dose hosts (p < 0.001). In a similar manner, the P mice of the one-gen exposure had a reduction from the 0 mg/kg/day value of 7.11 ± 1.7 to 3.74 ± 2.9 pg/ml (p < 0.001). Although not statistically significant, the F1 mice had an overall reduced IFNγ response, especially at the 62.5 mg/kg/day dose, compared to their parents.
Figure 6. In situ IFNγ production by SEB-activated T-cells from tungstate-exposed mice. Mice were exposed to tungstate for either 28 days or 12–19 weeks in a one generational exposure. IFNγ quantities in isolated plasma were measured by standard ELISA methods. (A) IFNγ in plasma 24 h after SEB or saline challenge. (B) IFNγ in plasma of P and F1 generations 24 h after SEB or saline challenge. All values shown are mean ± SE from six mice/indicated group. *p < 0.001 versus control, **p < 0.001 versus control.
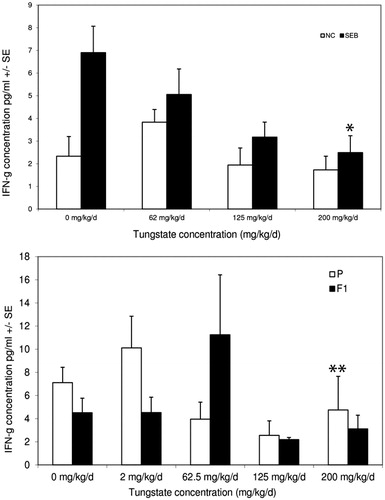
To determine if there were any more immunologically relevant effects in response to SEB after tungstate exposure, the magnitude of footpad swelling by Type IV DTH induction was measured. In separate DTH trials () the 200 mg/kg/day dose resulted in significantly less swelling in the NP-O-Su challenged footpads. In the first experiment (DTH 1), both the 20 and 200 mg/kg/day-dosed hosts had less edema than the saline controls (control vs 20 mg/kg/day [p < 0.017]; control vs 200 mg/kg/day [p < 0.013]). In a subsequent series (DTH 2), it was again found that there was significantly reduced swelling as a result of the 200 mg tungstate/kg/day treatment (p < 0.029). However, at two lower doses (i.e. 2 and 0.2 mg/kg/day), significantly reduced swelling compared to control mice that had not been exposed to tungstate was not observed.
Figure 7. Tungstate-dependent immune suppression in a delayed-type hypersensitivity model. Mice were exposed to tungstate in their drinking water for 28 days prior to the initiation of primary and secondary immune responses with NP-O-Su. The left footpad received saline injection, and the right footpad immunogen. Twenty-four hours after secondary challenge, footpad thickness was measured using dial gauges. The difference between left and right footpad thickness for each mouse was calculated and plotted; each bar represents the mean ± SE of eight mice. DTH1 and DTH2 represent separate DTH experiments with only the control and 200 mg/kg/day doses being in common between the mice. *p < 0.017 versus control (DTH1), ‡p < 0.013 versus control (DTH1), **p < 0.029 versus control (DTH2).
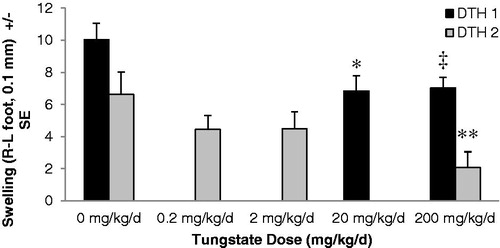
Discussion
The data presented here demonstrate that tungstate exposure by oral routes can result in immunosuppression. This has been shown by the reduction in the quantity of CD71+ helper and cytotoxic T-cells present in the high-dose tungstate exposure groups (200 mg/kg/day) when challenged with SEB. Consistent with immunosuppression after SEB exposure, a reduced immune-dependent footpad swelling after DTH induction was also observed. The reduced swelling with the medium (20 mg/kg/day) and high (200 mg/kg/day) doses of tungstate demonstrated that the influx of immune cells and associated inflammation was significantly diminished in the tungstate-treated animals compared to the controls. Taking these findings together, it appears that tungstate exposure can potentially result in the reduced ability of the host immune system to respond appropriately to challenges, which may increase the risk of an individual to suffer adverse outcomes after exposure to pathogens. Previous work with tungstate has shown that bioaccumulation occurs in a manner consistent with a two-compartment model (Leggett, Citation1997). Consistent with this work we found significant accumulations in bone, spleen, liver, and kidneys after 28 days of exposure (Guandalini et al., Citation2011). The bone marrow and spleen are important immunological organs, and higher tungstate concentrations locally might affect immune processses needed for host defense.
In the current experiments, immunophenotyping was used to examine type and quantity of various immune-related cells present in tissues after tungstate exposure. In particular, we showed there were significantly fewer activated (CD71+) T-cytotoxic (TCTL) and T-helper (TH) cells in spleens of SEB-challenged mice when compared to controls. SEB is a bacterial super-antigen that cross-links T-cell receptors (TCR) with the major histocompatibility complex Class II (MHC II) on antigen-presenting cells (APC; Krakauer, Citation2005). This, in turn, activates lymphocytes independently of requirements for MHC II-bound peptides and receptor co-stimulation. After tungstate exposure, any significant changes in the overall abundance of lymphocytes or other hematological parameters were not observed. While there was a trend towards reduced numbers of white blood cells (WBC) (), this was not statistically significant. Consistent with this, we did not observe changes (i.e. CD3+CD4+ and CD3+CD8+) of the activated (CD71+) cells ( and ) in the parent populations. This indicates that the depression of CD71+ TH and TCTL cells resulted from alterations in lymphocyte activation/proliferation due to tungstate rather than pathological hematopoietic changes in overall abundance of lymphocytes. Among myeloid-derived cells (i.e., macrophages, monocytes, neutrophils), no tungstate-dependent changes were observed (data not shown). As the myeloid markers used in this study did not examine early and immature sets of these cells, the measured myeloid populations were already terminally differentiated; as such, they could not respond to proliferative stimuli by cell division. It is possible, however, that functional changes in myeloid populations may be present, but were not examined. Previous in vitro work suggests this may be the case (Osterburg et al., Citation2010), as reduced cytokine production in LPS-, ConA-, and CD3/CD28-stimulated PBMC was noted.
Reactive oxygen species from XO are well-known contributors to oxidative stress, and inhibition of XO by allopurinol, tungstate, or other inhibitors can significantly reduce re-perfusion injury (Fang et al., Citation2010; Nielsen et al., Citation1997; Ono et al., Citation2009; Peglow et al., Citation2011). Additionally, ROS from XO has been described to be a requirement for antigen presentation in Kupffer cells (Matsue et al., Citation2003; Maemura et al., Citation2005) for secondary messenger signaling during antigen presentation. Indeed, a significant reduction in T-cell proliferation occurs when intracellular ROS production by either XO or NADPH oxidase is inhibited. This may be a mechanism that explains the tungstate-dependent immune suppression we observed in the 28-day, one-gen, and DTH exposures. The SEB immune challenge directly activates T-cells by cross-linking MHC II and the TCR without the requirement for peptide binding to MHC II or other co-stimulatory receptors. Other researchers have noted that, in a model of interstitial pneumonia, inhibition of XO by 4-amino-6-hydroxypyrazolo-(3,4-d)-pyrimidine reduces superoxide () and nitric oxide (NO) levels and thereby significantly diminishes SEB-dependent inflammation in these animals (Miyakawa et al., Citation2002).
Furthermore, tungstate-dependent inhibition of XO ROS production may affect the intracellular and extracellular redox environment. Sklavos et al. (Citation2008) have shown that altering the redox state of CD8 TCTL cells can reduce proliferation and cytokine release. Others have shown that redox modulation can reduce the effectiveness of APC (Tse et al., Citation2004). These data would be consistent with decreased DTH responses we noted here in NP-O-Su-challenged mice. Indeed, XO inhibition by a synthetic compound significantly reduced DTH responses in dinitro-fluorobenzene (DNFB)-sensitized Balb/c mice (Horiuchi et al., Citation1999). Allopurinol, another inhibitor of XO, has been shown to be immunosuppressive in ovalbumin (OVA)-sensitized Balb/c mice (Kato et al., Citation2000).
Immune suppression may also be due to increased apoptosis in splenic lymphocytes. We showed in a previous study increased apoptosis in peripheral blood leukocytes (PBL) after 48 and 72 h of exposure to various doses of tungstate (Osterburg et al., Citation2010). These in vitro experiments demonstrated that at 0.1 mM and 1 mM of tungstate there were significant increases in the quantity of cells undergoing early and late apoptosis after activation with ConA. In terms of in vivo tungsten concentrations we observed splenic concentrations of 7–14 ppm in the 200 mg/kg/day group in the 28-day study (Guandalini et al., Citation2011). These doses are in the range of the 0.1 mM tungstate used in the in vitro experiments of ∼24 ppm of W, where effects on cell cycle were observed. While the effects at lower dose were not necessarily changed as compared to controls, the longer exposure in the animal may alter the outcome. Additionally, the data suggest that there is a greater impact on lymphocyte apoptosis in activated cells. This would be in accordance with the data from the current studies, where we did not observe here significant changes in the quantity of TH and TCTL cells in control mice in either the 28-day or one-gen study. There is increased background (CD71) in the non-challenged mice in the one-gen studies; however, the reason for this is unknown and the differences between doses were not statistically significant. Regardless, very little effect of tungstate was seen in the absence of an immune challenge on baseline immunological parameters.
Previously, it was observed that high doses of tungstate reduced the quantity of cells undergoing cell division (Osterburg et al., Citation2010). This occurred in peripheral blood lymphocytes (PBL) at a 1 mM tungstate dose and with 1.0 and 0.1 mM tungstate in THP-1 cells; these data suggested that reduced quantities of TH and TCTL cells might be due to decreases in proliferation. The quantity of proliferation in splenocytes was measured by the expression of the CD71 surface transferrin receptor (that mediates iron uptake) and is strongly correlated with proliferation in lymphocytes (Nguyen et al., Citation2003; Premaud et al., Citation2011). We have consistently seen a reduced level of CD71 on SEB-treated cells in both the 28-day and one-gen study. Additionally, in a 90-day exposure (data not shown), reduced CD71 surface expression in the 200 mg/kg/day tungstate group was noted. Because CD71 reductions due to tungstate were only seen in SEB-challenged mice, this would suggest that reduced CD71 levels of TH and TCTL cells in the spleens of SEB-treated mice was a result of either suppressed cell cycling or, potentially, alterations to apoptosis of these cells.
Tungstate is a known phosphatase inhibitor and altered phosphorylation may account for the changes observed both in vitro and in vivo. Notably, cellular changes in phosphorylation depend on the cell line being examined (Johnson et al., Citation2010). We have seen changes in the phosphorylation of histone H1b, which would be consistent with reductions in the proliferative capacity of stimulated lymphocytes (Osterburg et al., Citation2010). Others have seen reduced ERK1/2, GSK3β, and p38 phosphorylation (Amigo-Correig et al., Citation2011; Gomez-Ramos et al., Citation2006; Piquer et al., Citation2007). Many observed effects of tungstate exposure in animals can be explained by this mechanism because changes in phosphorylation can alter key proteins that promote cell cycle progression. There is also the potential for blocking the transducing signal at the receptor, or at various points along intracellular phospho-signaling pathways.
SEB was employed in the current study as an activator of adaptive immunity. However, to show that tungstate was immunosuppressive in a more immunologically relevant sense, a delayed-type hypersensitivity Type IV mouse model (DTH) mediated by CD8 TCTL cells and CD4 TH cells (Pichler, Citation2003) was utilized. As seen in the 28-day and one-gen exposures, these were the cells affected by tungstate. It was shown in separate DTH experiments here that there was significantly reduced footpad swelling in hosts that received the 200 and 20 mg/kg/day tungstate doses. Lower doses (i.e. ≤2 mg/kg/day; NOAEL) did not result in significant decreases in swelling. These data were consistent with tungstate exposures that resulted in immunosuppression. The studies here also documented decreased quantities of CD71+ TH and TCTL cells in the spleens of these mice, although the data were suggestive but not statistically significant.
Rather than the spleen, in the case of DTH, it may have been more informative to examine lymphocyte populations in the foot-draining lymph nodes. The 20 mg/kg/day tungstate dose used in the DTH 1 experiment resulted in decreased swelling compared to control. Unlike in the SEB challenge, doses <200 mg tungstate/kg/day generally did not affect quantities of CD71+ cells. However, the mechanisms of immune stimulation between the SEB and DTH models were significantly different. SEB is a super-antigen and the co-stimulatory mechanisms for lymphocyte activation were not necessary. Because the DTH response requires normal physiological immune responses, it may be more sensitive to tungstate than a strong agonist like SEB. Additionally, immune suppression during DTH may be very dependent on the tungstate present in the microenvironment of the draining lymph nodes. This aspect of tungsten distribution has not yet been examined, and the mechanisms of immune suppression remain unknown. Changes in ROS, antigen presentation, and phosphorylation remain as possible mechanisms of effect. Additionally, cellular alterations due to tungstate that limit the ability of T-cells to proliferate after stimuli remain to be investigated.
The consequences of tungstate exposure, at least in animals, would suggest that exposure reduces host defense against infection. Data from Fastje et al. (Citation2009) are consistent with alterations in host defense. These researchers show that tumor suppressor gene DMBT1 expression was reduced in offspring after tungstate exposure in utero. Further, those authors later demonstrated that tungstate exposure – with subsequent exposure to respiratory syncytial virus – led to development of leukemia (Fastje et al., Citation2011). The mechanism of the DMBT1 suppression is unclear, but DMBT1 has been linked to immune function (Ligtenberg et al., Citation2007; Mollenhauer et al., Citation2000). It is possible that any reduced immune surveillance due to tungstate exposure might synergize with changes in expression of DMBT1.
The data here suggested that tungstate exposure by oral routes could result in immunologic outcomes wherein host defense was reduced. The dose required for immune suppression in the SEB models (200 mg/kg/day) was very high; it is unlikely that this dose will be encountered through environmental sources. In contrast, in the DTH model, the dose required to observe an effect was lower with 200 and 20 mg/kg/day of tungstate reducing host immune responses. These values were approximately equivalent to exposures of 1000 and 100 ppm W, respectively. Exposure to these levels is unlikely due to environmental sources, which are substantially lower (<1 ppm W) (Rubin et al., Citation2007). Even at military firing ranges, quantities of W from spent ammunitions can reach ∼400 ppm in the topsoil (Clausen & Korte, Citation2009); in groundwater at these sites, W levels were significantly less (<0.56 ppm). Alternatively, biomass from sites high in W presents a wide range of potential sources for W exposures (Wilson & Pyatt, Citation2006). Bio-burdens in forage plants and livestock in areas around active W mines can reach appreciable levels (Pyatt & Pyatt, Citation2004). Even so, the potential consequence to human health as a result of any W bioaccumulation by these potential food sources remains unknown.
When used as part of a pharmacologic regimen, tungstate levels attained in a host could be much higher than what might be associated with environmental encounters. At the time of the current study, a group in Spain was exploring the potential use of tungstate as a weight loss supplement (due to preliminary findings that oral tungstate exposure resulted in weight loss in rats) (Amigo-Correig et al., Citation2011; Munoz et al., Citation2001; Rodriguez-Hernandez et al., Citation2012). Nevertheless, while a recent study did not provide evidence for tungstate-dependent weight loss (Hanzu et al., Citation2010), other pharmacological uses of tungstate may still be possible (e.g. as a replacement for allopurinol against Type 4 hypersensitivity pathologies). There also is the possibility of high W doses being attained after accidental exposure. In one instance, a soldier was exposed to significant quantities of W by drinking wine from a recently-used gun barrel. Post-exposure, he presented with sequelae that included acute nausea, seizures, renal failure, and onset of coma (Marquet et al., Citation1996, Citation1997). In both the pharmacologic and accidental exposure cases, under acidic conditions in the stomach or in soils, tungstate could have polymerized into forms that possess greater toxicity as polymers (i.e., metatungstate) than as the monomer (Strigul et al., Citation2010). The effects of metatungstate and other polymeric forms remain largely unexplored; thus, as with tungstate itself, these should be investigated further.
Taken together, the data from the current studies clearly indicate tungstate exposure could result in suppression of adaptive immunity. Our data indicates little to no effect of tungstate at any dose on groups of mice exposed only to tungstate without being co-exposed to an immune stressor (e.g. SEB or DTH). This may suggest that some biological processes, such as T-cell activation, are more sensitive to tungstate. Additionally, observation of these tungstate-dependent toxic effects may depend on activation of specific cellular pathways. While the quantity of tungstate necessary to reduce adaptive immunity would appear to be uncommon from environmental sources, the plausibility for such outcomes arising from either pharmacologic or accidental exposures, or from long-term (re: bioaccumulation) exposures, to tungstate remains high, and needs to be investigated further.
Declaration of interest
The authors report no conflicts of interest. The views expressed in this article are those of the author and do not necessarily reflect the official policy or position of the Department of the Navy, Department of Defense, nor the US Government. This work was supported by work unit number 60862. The experiments reported herein were conducted in compliance with the Animal Welfare Act and in accordance with the principles set forth in the ‘Guide for the Care and Use of Laboratory Animals’, Institute of Laboratory Animals Resources, National Research Council, National Academy Press, 1996. V.M., M.S. and G.C. are military service members (or employee of the U.S. Government). This work was prepared as part of my official duties. Title 17 U.S.C. §105 provides that ‘Copyright protection under this title is not available for any work in the United States Government’. Title 17 U.S.C. §101 defines a US Government work as a work prepared by a military 762 service member or employee of the US Government as part of that person’s official duties.
Acknowledgements
We would like to thank our summer interns, Connie Fu, Thomas Park, Michael Sun, and Elizabeth Marconne, for their help in the day-to-day management of this study. We would also like to thank staff of the Environmental Health Effects Laboratory at Wright-Patterson Air Force Base. These individuals helped in various ways during the experiments: Sue Prues, Tracy Doyle, Shawn McInturf, Pedro Ortiz, Karen Mumy, and Michelle Okolica.
References
- Amigo-Correig, M., Barcelo-Batillori, S., Piquer, S., et al. 2011. Sodium tungstate regulates food intake and body weight through activation of the hypothalamic leptin pathway. Diabetes Obes. Metab. 13:235–242
- Babcock, G. F., and Dawes, S. M. 1994. Immunophenotyping using fixed cells. Meth. Cell Biol. 41:81–93
- Bommireddy, R., Saxena, V., Ormsby, I., et al. 2003. TGF-β1 regulates lymphocyte homeostasis by preventing activation and subsequent apoptosis of peripheral lymphocytes. J. Immunol. 170:4612–4622
- Briner, W. 2010. The toxicity of depleted uranium. Int. J. Environ. Res. Publ. Health. 7:303–313
- Briner, W. E. 2006. The evolution of depleted uranium as an environmental risk factor: Lessons from other metals. Int. J. Environ. Res. Publ. Health. 3:129–135
- Chen, Y., Jakoncic, J., Carpino, N., and Nassar, N. 2009. Structural and functional characterization of the 2H-phosphatase domain of Sts-2 reveals an acid-dependent phosphatase activity. Biochemistry. 48:1681–1690
- Clausen, J. L., and Korte, N. 2009. Environmental fate of tungsten from military use. Sci. Total Environ. 407:2887–2893
- Dominguez, J. E., Munoz, M. C., Zafra, D., et al. 2003. The anti-diabetic agent sodium tungstate activates glycogen synthesis through an insulin receptor-independent pathway. J. Biol. Chem. 278:42785–42794
- Egloff, M. P., Cohen, P. T., Reinemer, P., and Barford, D. 1995. Crystal structure of the catalytic subunit of human protein phosphatase 1 and its complex with tungstate. J. Mol. Biol. 254:942–959
- Fang, J., Seki, T., Qin, H., et al. 2010. Tissue protective effect of xanthine oxidase inhibitor, polymer conjugate of (styrene-,maleic acid copolymer) and (4-amino-6-hydroxypyrazolo[3,4-d]pyrimidine), on hepatic ischemia-reperfusion injury. Exp. Biol. Med. (Maywood). 235:487–496
- Fastje, C. D., Harper, K., Terry, C., et al. 2011. Exposure to sodium tungstate and Respiratory Syncytial Virus results in hematological/immunological disease in C57BL/6J mice. Chem. Biol. Interact. 196:89–95
- Fastje, C. D., Kim, L., Sun, N. N., et al. 2009. Prenatal exposure of mice to tungstate is associated with decreased transcriptome-expression of the putative tumor suppressor gene, DMBT1: Implications for childhood leukemia. Land. Contam. Reclam. 17:169–178
- Gomez-Ramos, A., Dominguez, J., Zafra, D., et al. 2006. Sodium tungstate decreases the phosphorylation of tau through GSK3 inactivation. J. Neurosci. Res. 83:264–273
- Guandalini, G. S., Zhang, L., Fornero, E., et al. 2011. Tissue distribution of tungsten in mice following oral exposure to sodium tungstate. Chem. Res. Toxicol. 24:488–493
- Hanzu, F., Gomis, R., Coves, M. J., et al. 2010. Proof-of-concept trial on the efficacy of sodium tungstate in human obesity. Diabetes Obes. Metab. 12:1013–1018
- Horiuchi, H., Ota, M., Kitahara, S., et al. 1999. Allopurinol increases ear swelling and mortality in a dinitrofluorobenzene-induced contact hypersensitivity mouse model. Biol. Pharm. Bull. 22:810–815
- Johnson, D. R., Ang, C., Bednar, A. J., and Inouye, L. S. 2010. Tungsten effects on phosphate-dependent biochemical pathways are species and liver cell line dependent. Toxicol. Sci. 116:523–532
- Johnson, J. L., Rajagopalan, K. V., and Cohen, H. J. 1974. Molecular basis of the biological function of molybdenum. Effect of tungsten on xanthine oxidase and sulfite oxidase in the rat. J. Biol. Chem. 249:859–866
- Kalinich, J. F., Emond, C. A., Dalton, T. K., et al. 2005. Embedded weapons-grade tungsten alloy shrapnel rapidly induces metastatic high-grade rhabdomyosarcomas in F344 rats. Environ. Health Perspect. 113:729–734
- Kato, C., Sato, K., Wakabayashi, A., and Eishi, Y. 2000. The effects of allopurinol on immune function in normal BALB/c and SCID mice. Int. J. Immunopharmacol. 22:547–556
- Krakauer, T. 2005. Chemotherapeutics targeting immune activation by staphylococcal super-antigens. Med. Sci. Monit. 11:RA290–295
- Leggett, R. W. 1997. A model of the distribution and retention of tungsten in the human body. Sci. Total Environ. 206:147–165
- Ligtenberg, A. J., Veerman, E. C., Nieuw Amerongen, A. V., and Mollenhauer, J. 2007. Salivary agglutinin/glycoprotein-340/DMBT1: A single molecule with variable composition and with different functions in infection, inflammation and cancer. Biol. Chem. 388:1275–1289
- Luo, Y., and Dorf, M. E. 2001. Delayed-type hypersensitivity. In: Current Protocols in Immunology. (Bierer, B. E., Coligan, J. E., Margulies, D. H., Shevach, E. M., and Strober, W., Somerset, NJ: John Wiley & Sons. Chapter 4, Unit 45
- Maemura, K., Zheng, Q., Wada, T., et al. 2005. Reactive oxygen species are essential mediators in antigen presentation by Kupffer cells. Immunol. Cell. Biol. 83:336–343
- Marquet, P., Francois, B., Lotfi, H., et al. 1997. Tungsten determination in biological fluids, hair, and nails by plasma emission spectrometry in a case of severe acute intoxication in man. J. Forensic Sci. 42:527–530
- Marquet, P., Francois, B., Vignon, P., and Lachatre, G. 1996. A soldier who had seizures after drinking quarter of a litre of wine. Lancet. 348:1070
- Matsue, H., Edelbaum, D., Shalhevet, D., et al. 2003. Generation and function of reactive oxygen species in dendritic cells during antigen presentation. J. Immunol. 171:3010–3018
- McInturf, S. M., Bekkedal, M. Y., Wilfong, E., et al. 2011. The potential reproductive, neurobehavioral and systemic effects of soluble sodium tungstate exposure in Sprague-Dawley rats. Toxicol. Appl. Pharmacol. 254:133–137
- Miyakawa, H., Sato, K., Shinbori, T., et al. 2002. Effects of inducible nitric oxide synthase and xanthine oxidase inhibitors on SEB-induced interstitial pneumonia in mice. Eur. Respir. J. 19:447–457
- Mollenhauer, J., Herbertz, S., Holmskov, U., et al. 2000. DMBT1 encodes a protein involved in the immune defense and in epithelial differentiation and is highly unstable in cancer. Cancer Res. 60:1704–1710
- Munoz, M. C., Barbera, A., Dominguez, J., et al. 2001. Effects of tungstate, a new potential oral antidiabetic agent, in Zucker diabetic fatty rats. Diabetes. 50:131–138
- Nguyen, X. D., Eichler, H., Dugrillon, A., et al. 2003. Flow cytometric analysis of T-cell proliferation in a mixed lymphocyte reaction with dendritic cells. J. Immunol. Meth. 275:57–68
- Nielsen, V. G., Tan, S., Baird, M. S., et al. 1997. Xanthine oxidase mediates myocardial injury after hepatoenteric ischemia-reperfusion. Crit. Care Med. 25:1044–1050
- Nishino, T., Okamoto, K., Eger, B. T., and Pai, E. F. 2008. Mammalian xanthine oxidoreductase - mechanism of transition from xanthine dehydrogenase to xanthine oxidase. FEBS J. 275:3278–3289
- Ono, T., Tsuruta, R., Fujita, M., et al. 2009. Xanthine oxidase is one of the major sources of superoxide anion radicals in blood after reperfusion in rats with forebrain ischemia/reperfusion. Brain Res. 1305:158–167
- Osterburg, A. R., Robinson, C. T., Schwemberger, S., et al. 2010. Sodium tungstate (Na2WO4) exposure increases apoptosis in human peripheral blood lymphocytes. J. Immunotoxicol. 7:174–182
- Pacher, P., Nivorozhkin, A., and Szabo, C. 2006. Therapeutic effects of xanthine oxidase inhibitors: Renaissance half a century after the discovery of allopurinol. Pharmacol. Rev. 58:87–114
- Peglow, S., Toledo, A. H., Anaya-Prado, R., et al. 2011. Allopurinol and xanthine oxidase inhibition in liver ischemia reperfusion. J. Hepatobil. Pancreat. Sci. 18:137–146
- Pichler, W. J. 2003. Delayed drug hypersensitivity reactions. Ann. Intern. Med. 139:683–693
- Piquer, S., Barcelo-Batillori, S., Julia, M., et al. 2007. Phosphorylation events implicating p38 and PI3K mediate tungstate-effects in MIN6 beta cells. Biochem. Biophys. Res. Commun. 358:385–391
- Premaud, A., Rousseau, A., Johnson, G., et al. 2011. Inhibition of T-cell activation and proliferation by mycophenolic acid in patients awaiting liver transplantation: PK/PD relationships. Pharmacol. Res. 63:432–438
- Pyatt, F. B., and Pyatt, A. J. 2004. The bioaccumulation of tungsten and copper by organisms inhabiting metalliferous areas in North Queensland, Australia: An evaluation of potential health implications. J. Environ. Health Res. 3:13–18
- Rodriguez-Hernandez, C. J., Guinovart, J. J., and Murguia, J. R. 2012. Anti-diabetic and anti-obesity agent sodium tungstate enhances GCN pathway activation through Glc7p inhibition. FEBS Lett. 586:270–276
- Rubin, C. S., Holmes, A. K., Belson, M. G., et al. 2007. Investigating childhood leukemia in Churchill County, Nevada. Environ. Health Perspect. 115:151–157
- Sklavos, M. M., Tse, H. M., and Piganelli, J. D. 2008. Redox modulation inhibits CD8 T-cell effector function. Free Rad. Biol. Med. 45:1477–1486
- Steinberg, K. K., Relling, M. V., Gallagher, M. L., et al. 2007. Genetic studies of a cluster of acute lymphoblastic leukemia cases in Churchill County, Nevada. Environ. Health Perspect. 115:158–164
- Strigul, N., Koutsospyros, A., and Christodoulatos, C. 2010. Tungsten speciation and toxicity: Acute toxicity of mono- and poly-tungstates to fish. Ecotoxicol. Environ. Saf. 73:164–171
- Tse, H. M., Milton, M. J., and Piganelli, J. D. 2004. Mechanistic analysis of the immunomodulatory effects of a catalytic antioxidant on antigen-presenting cells: Implication for their use in targeting oxidation-reduction reactions in innate immunity. Free Rad. Biol. Med. 36:233–247
- Umezawa, K., Akaike, T., Fujii, S., et al. 1997. Induction of nitric oxide synthesis and xanthine oxidase and their roles in the anti-microbial mechanism against Salmonella typhimurium infection in mice. Infect. Immun. 65:2932–2940
- Verma, R., Xu, X., Jaiswal, M. K., et al. 2011. In vitro profiling of epigenetic modifications underlying heavy metal toxicity of tungsten-alloy and its components. Toxicol. Appl. Pharmacol. 253:178–187
- Vorbach, C., Harrison, R., and Capecchi, M. R. 2003. Xanthine oxidoreductase is central to the evolution and function of the innate immune system. Trends Immunol. 24:512–517
- Wilson, B., and Pyatt, F. B. 2006. Bio-availability of tungsten in the vicinity of an abandoned mine in the English Lake District and some potential health implications. Sci. Total Environ. 370:401–408
- Wright, R. M., Ginger, L. A., Kosila, N., et al. 2004. Mononuclear phagocyte xanthine oxidoreductase contributes to cytokine-induced acute lung injury. Am. J. Respir. Cell. Mol. Biol. 30:479–490
- Yang, J., Liang, X., Niu, T., et al. 1998. Crystal structure of the catalytic domain of protein-tyrosine phosphatase SHP-1. J. Biol. Chem. 273:28199–28207