Abstract
Carbon nanotubes (CNTs) represent one of the most promising engineered nanomaterials, with possible applications in advanced engineering and biomedical technologies. During their production, human exposure to CNTs may occur via inhalation. Therefore, the aim of this study was to mimic inhalation of multi-walled CNTs (MWCNTs) in vitro as realistically as possible, by producing MWCNTs aerosols via an Air–Liquid Interface Cell Exposure System (ALICE), combined with a 3D epithelial airway barrier model cultivated at the air–liquid interface (ALI). To address the consequences of an extended exposure period, repeated exposures of MWCNTs (total deposition 1.15 μg/cm2) were applied to the co-culture system, either over one day (one day repeated exposure) or three days (three day repeated exposure scenario). Although in both repeated exposure scenarios MWCNTs were found to interact with the different cell types, they did not induce any cytotoxicity or alterations in cell morphology, nor did they elucidate any significant increase in pro-inflammatory markers compared to control cultures. Similar results were also observed following single MWCNTs exposures at deposited concentrations of 0.14, 0.20 and 0.39 µg/cm2. Cells exposed repeatedly to MWCNTs for three days, however did show a decrease in reduced glutathione levels, although not significant (p > 0.05). In conclusion, we have presented a realistic in vitro alternative to mimic occupational exposure of MWCNTs and by applying this approach it was shown that repeated MWCNT exposures to lung cell cultures at the ALI elicit a limited biological impact over a three day period.
Introduction
Carbon nanotubes (CNTs) are being manufactured at a range of 11–1000 tons per year, and represent one of the most promising nanomaterials (Piccinno et al., Citation2012). Their unique strength and excellent electrical, spectroscopic and thermal properties (Donaldson et al., Citation2006; Kostarelos et al., Citation2009; Thurnherr et al., Citation2009; Zhang et al., Citation2007) have led to various consumer and industrial applications ranging from conducting composites or electronics to sports equipment and protective clothing (Robertson, Citation2004; Van Berlo et al., Citation2012). These exceptional characteristics, as well as the ability for CNTs to penetrate the plasma membrane together with their large loading capability to carry various bioactive agents (e.g. drugs) are intensively being explored for potential biomedical applications, in detection, monitoring and therapy of diseases (De la Zerda et al., Citation2008; Kostarelos et al., Citation2009; Zavaleta et al., Citation2008; Zhang et al., Citation2010).
Human exposure to CNTs is possible to occur via the skin, the blood circulation or the gastrointestinal tract (Oberdörster et al., Citation2005). Nonetheless, inhalation is widely accepted as the primary route of entry for nanomaterials, such as CNTs, especially when considering occupational exposure (Oberdorster et al., Citation2007). Inhalation of CNTs may lead to an innate immune response and activation of a variety of intracellular signaling cascades resulting in potentially hazardous consequences in human health (Johnston et al., Citation2010; Van Berlo et al., Citation2012). Although, an increasing amount of studies have tried to investigate the CNT–lung cell interaction, the current picture of the pulmonary toxicity of industrially produced CNTs remains unclear. Most notably, research studies often appear conflicting due to variations in: applied doses, physicochemical characteristics of CNTs (length, width, number of walls), and dispersion state or the test system used (Clift et al., Citation2013; Gasser et al., Citation2012; Thurnherr et al., Citation2009; Wick et al., Citation2007).
The extreme high aspect ratio of CNTs together with their potential low solubility in the lungs have raised the concern that long-term exposure to CNTs via inhalation may also lead to similar lung pathology analogous to those observed with other fibrous particles (Maynard et al., Citation2004). In the first long-term inhalation study, rats exposed to multi-walled CNTs (MWCNTs) aerosols up to 2.5 mg/m3 showed increased lung weights, clear inflammation and granuloma formation, but no pulmonary fibrotic effects (Ma-Hock et al., Citation2009). Whilst in another 13 week sub-chronic study in which rats were exposed to 0.4–6 mg/m3 MWCNTs, it was demonstrated that the induced pathological changes are consistent with overload-related phenomena (Pauluhn, Citation2010). Furthermore, in a recent study, rats exposed repeatedly to a 5 mg/m3 MWCNT aerosol showed fibrotic response that was found to develop and persist up to 336 days (d) after exposure (Mercer et al., Citation2013). Although it represents a severe overload study, Ryman-Rasmussen et al. also reported increased pleural mononuclear cell accumulation and sub-pleural fibrosis up to 6 weeks after single inhalation exposure of 30 mg/m3 for 6 h in mice (Ryman-Rasmussen et al., Citation2009). In another in vivo inhalation study, mice were exposed repeatedly for 19 days to 1970 ng/d (corresponding to 1000 d of a human exposure), 197 ng/d (100 d human exposure) and 19.7 ng/d (10 d) MWCNTs, with a post-incubation time up to 84 days (Erdely et al., Citation2013). Mice exposed to high doses of CNTs showed persistent cytotoxicity and inflammation 84 d after exposure, whereas mice exposed to 197 ng/d CNTs showed transient cytotoxicity and no polymorphonuclear cell influx. For the lowest test dose, only a low grade inflammatory response was observed.
So far, attempts to nebulise CNTs have focused mainly on animal-based in vivo strategies (McKinney et al., Citation2009; Kasai et al., Citation2014; Porter et al., Citation2013). In addition, many publications on acute or long-term consequences of industrial CNT exposures were performed in vitro by using lung cell cultures under submerged conditions (Clift et al., Citation2014; Snyder-Talkington et al., Citation2013; Tabet et al., 2009; Thurnherr et al., Citation2011; Wang et al., 2011). However, such exposures in suspension do not represent a realistic situation of what would be expected in the human lung when fibers are inhaled. Since exposure to CNTs might occur repetitively, i.e. occupational exposures at the workplace or biomedical applications, it is crucial to address the biological effects upon repeated CNTs exposure. Consequently, the aim of this study was to mimic inhalation of CNTs in vitro and reveal the mechanisms that underlay the manifestation of potential adverse effects, by producing MWCNTs aerosols using the Air–Liquid Interface Cell Exposure System (ALICE) as described by Lenz et al. (Citation2009) for spherical ZnO nanoparticles (NPs), as well as gold NPs (Brandenberger et al., Citation2009) and silver NPs (Herzog et al., Citation2013). Recently, it has further been highlighted as a reliable system for the aerosolisation of high aspect ratio materials such as cellulose nanocrystals (Endes et al., Citation2013, Citation2014). As a lung cell model a sophisticated 3D in vitro model of the human epithelial airway barrier composed of epithelial cells as well as human blood monocyte derived macrophages and dendritic cells was used (Rothen-Rutishauser et al., Citation2005). Single exposures were compared with repeated exposures over a period of three days.
Materials and methods
Chemical and Reagents
All chemicals and reagents used were purchased from Sigma Aldrich (Buchs, Switzerland), unless otherwise stated.
Lung cell cultures
Since upon inhalation, nanomaterials deposit mainly in the alveolar region of the lung (Oberdörster et al., Citation2005), we have used the A549 (alveolar type II) cell line combined with two primary immune cells, as described by Rothen-Rutishauser et al. (Citation2005, Citation2008). When A549 cells are cultured at the air–liquid interface (ALI), they produce surfactant, which is released at the apical side of the cells, resulting in a surface tension similar to values measured in vivo, in pulmonary alveoli (Blank et al., Citation2006).
Briefly, the co-culture consists of the human (alveolar) epithelial A549 cell line, combined with human monocyte-derived dendritic cells (MDDCs) and macrophages (MDMs). A549 cells were cultured in Rosewell Park Memorial Institute (RPMI) 1640 supplemented medium with 10% Fetal Calf Serum (FCS; PAA Laboratories, Chemie Brunschwig AG, Basel, Switzerland), 1% l-Glutamine (L-Glut; Life Technologies, Europe, Zug, Switzerland), 1% Penicillin/Streptomycin (P/S; 10 000 units/ml/10 000 μg/ml, Gibco, Zug, Switzerland) at 37 °C, 5% CO2. Cells were seeded at a density of 0.5 × 106 cells/ml, in BD Falcon™ cell culture inserts for 6-well plates (high pore density transparent PET membrane, with 3 μm diameter pore size and 4.2 cm2 effective growth area; BD biosciences, Allschwil, Switzerland). Inserts were placed in six-well culture plates (BD biosciences) and cells were grown for 5 days under submerged conditions (2 ml medium in the upper and 3 ml in the lower chamber of the insert). Peripheral human blood monocytes were isolated from human blood buffy coats (Blood Donation Service, Bern University Hospital, Bern, Switzerland), as previously described by Blank et al. (Citation2011). Monocytes were cultured for 6 days at a density of 106 cells/ml in supplemented RPMI medium as used for the epithelial cells. For the generation of MDDCs and MDMs, the growth factors GM-CSF, IL-4 (for MDDC [10 ng/ml]) and MCSF (for MDM [10 ng/ml]) were added to the culture medium.
The triple co-cultures were achieved, as previously described by Blank et al. (Citation2007), resulting in a cell density of ∼9714 epithelial cells/mm2, ∼231 MDM/mm2, and ∼411 MDDC/mm2. After 24 h under submerged conditions the triple co-cultures are transferred to the ALI conditions, by removing the medium in the upper chamber and replacing the medium in the lower transwell chamber with 1.2 ml of fresh culture medium. The cells were exposed to air for additional 24 h and then the co-cultures were ready for the experimentation.
Air--Liquid Interface Cell Exposure System
Nebulisation of MWCNTs was performed using the ALICE as described by Lenz et al. (Citation2009) and Endes et al. (Citation2013, Citation2014). Briefly, the ALICE consists of a nebuliser (droplet generator), an exposure chamber, an incubation chamber (which is connected to a flow system providing humidity and temperature conditions suitable for cell cultivation) as well as a quartz crystal microbalance (QCM; detection limit 0.09 μg/cm2, 5 MHz, Stanford Research Systems, GMP SA, Renens, Switzerland) for real-time determination of the deposited material. For each exposure, 1 ml of MWCNT suspension with 500 μM NaCl (NAAPREP® physiological saline, GlaxoSmithKline, France) was added in the nebuliser (customised eFlow nebuliser system, PARI Pharma GmbH, Starnberg, Germany). The aerosol is generated by the vibrating perforated membrane of the eFlow nebuliser, transported in the exposure chamber (flow rate: 5 l/min), where it gently deposits onto cells cultured at the ALI. The chosen flow rate is optimal for the cloud to get sufficiently mixed and diverted to all sides of the exposure chamber, thus resulting in uniform droplet deposition. Quantification of MWCNT deposition (μg/cm2) was measured by the incorporated quartz crystal microbalance (QCM). Briefly, the deposited MWCNT mass was quantified by the QCM which was placed inside the exposure chamber of the ALICE. At the end of each aerosolisation process, the QCM was taken out of the chamber and left to air-dry. The quantification of the deposited material, on the vibrating piezoelectric crystal of the QCM, is a result of the linear decrease in the resonance frequency of the crystal due to increasing deposited mass. The difference in the recorded frequency of the crystal before and after the nebulisation process was measured and subsequently converted to a metric of mass per area (µg/cm2), as previously described in detail by Lenz et al. (Citation2009).
MWCNTs and positive controls samples
MWCNTs (Cheaptubes Inc., Grafton, VT) dispersed in Pluronic F127 (160 ppm) were used as previously described in Thurnherr et al. (Citation2009). The stock suspension of MWCNTs (250 µg/ml) was diluted in Pluronic F127 to the working concentrations of 25 and 125 µg/ml. Detailed characterisation of MWCNT suspensions in terms of length, diameter and endotoxin level were previously reported by Thurnherr at al. (Citation2009) and Clift et al. (Citation2011a).
To examine potential biological effects of the dispersant in the co-culture system, Pluronic F127 was assessed independently at a concentration of 160 ppm and tested with all biochemical endpoints used. Additionally, crystalline Dörentruper Quartz (DQ12; ≤5 μm; Robock, Citation1973) at a concentration of 0.1 mg/ml (Endes et al., Citation2014) was used as a positive (pro-)inflammatory “particle control”, since it is known to induce severe pulmonary inflammation (Clouter et al., Citation2001; Monteiller et al., Citation2007).
Exposures scenarios
To assess the biological impact of a single exposure of aerosolised MWCNTs, cells were exposed to three different concentrations of MWCNTs (25, 125 and 250 μg/ml) using the ALICE system and the cellular response was determined after a post-incubation time of 24 h.
Subsequently, to address the effects of a prolonged exposure period, repeated exposures of nebulised MWCNTs (250 μg/ml) were applied to the triple co-cultures, either three times over one day (one day scenario) or three times in three days (three day scenario). Similar, to the single exposures the biological effects were assessed after a 24 h post-incubation period following the last MWCNT exposure.
Transmission electron microscopy
Characterisation of MWCNT deposition
In order to characterise the deposited MWCNT aerosols, transmission electron microscopy (TEM) copper grids were exposed to nebulised particles using the ALICE system. Representative images of deposited MWCNTs were captured using a Hitachi H-7100 (Hitachi, Japan) at 75 kV fitted with a Morada 11 MPix digital CCD camera 14 (Olympus, Japan). The average length and width distribution of nebulised MWCNTs was derived from 130 individual measurements using ImageJ software (Schneider et al., Citation2012).
Interaction of MWCNTs with the triple co-culture
Intracellular MWCNTs were also visualised by TEM (FEI Morgagni, Phillips Electron Optics, Zurich, Switzerland) operated at 80 kV. Prior to the TEM analysis, the exposed cells were fixed with 2.5% glutaraldehyde in HEPES buffer, and then post-fixed with 1% osmium tetroxide and stained with 0.5% uranyl acetate. Subsequently, the samples were dehydrated in a graded ethanol series and embedded in epon (Blank et al., Citation2011). Finally, ultrathin sections (50–80 nm) were cut from the embedded samples, mounted on copper grids and stained with lead citrate and uranyl acetate.
Biochemical analysis
Lactate dehydrogenase (LDH) release
The release of lactate dehydrogenase (LDH) was assessed, since this is known to be a useful indicator for cytotoxicity (Clift et al., Citation2011b). For this reason, the medium in the lower chamber of the inserts was collected and analyzed using a LDH cytotoxicity detection kit (Roche Applied Science, Mannheim, Germany), according to the manufacturer’s manual. Determination of LDH activity was performed photometrically by measuring the absorbance at 490 nm (reference wavelength at 630 nm). Each sample was tested in triplicate and LDH values were expressed relative to negative control. For positive controls, co-cultures were exposed apically to 0.2% Triton X-100 in H2O (100 μl) for 24 h.
Cell morphology
After the post-incubation period, co-cultures were fixed for 15 min in 3% paraformaldehyde (PFA) in phosphate buffered saline (PBS) and treated with 0.1 M glycine in PBS for another 15 min or stored at 4 °C. To permeabilise the cell membrane, cells were treated with 0.2% Triton X-100 in PBS for 15 min. Phalloidin rhodamine (R-415; Molecular Probes, Life Technologies Europe B.V., Zug, Switzerland) was used to stain the F-actin cytoskeleton at a 1:50 dilution, whilst the nucleus was stained with DAPI ([1 μg/ml] in 0.3% Triton X-100 in PBS) (Sigma Aldrich, Switzerland), respectively. For optical analysis, samples were embedded in Glycergel (DAKO Schweiz AG, Baar, Switzerland). Visualisation of samples was achieved by using an inverted laser scanning confocal microscope (LSM) 710 (Axio Observer.Z1, Carl Zeiss, Jena, Germany). Image processing was performed using the restoration software IMARIS (Bitplane AG, Zurich, Switzerland).
Oxidative stress
The concentration of total reduced glutathione (GSH) was determined with a diagnostic glutathione assay kit (Cayman Chemical Company, Ann Arbor, MI), following the manufacturer’s instructions. Briefly, cells were collected by gently scrapping them from the insert membrane. Cells were then homogenised in 50 mM cold MES buffer (2-(N-morfolino)ethanesulphonic acid) and centrifuged (10 000 g for 15 min at 4 °C). The cell sample was then deproteinated by the addition of equal volumes of metaphosphoric acid (MPA) reagent to each sample. After 5 min standing at RT, samples were centrifuged (2000 g for 5 min at 4 °C) and assessed for their GSH content according to the manufacturer’s protocol. GSH values are reported relative to the total amount of protein of each sample, as determined by the Pierce bicinchoninic acid (BCA) protein assay kit (Pierce Protein research Products, Thermo Scientific, Rockford, IL). Cells exposed to (100 mM) tert-butyl hydrogen peroxide (TBHP) served as the positive control.
Cytokine/chemokine quantification
The (pro-)inflammatory response of the triple co-culture after MWCNTs exposure was measured by quantifying the amount of the pro-inflammatory mediators, interleukin 1β (IL-1β), tumor necrosis factor α (TNF-α) and interleukin 8 (IL-8) by using the commercially available DuoSet ELISA Development Kit (R&D Systems, Switzerland) according to the supplier’s protocol. Co-cultures treated with Lipopolysaccharide (LPS from Pseudomonas aeruginosa; 1 μg/ml; Sigma Aldrich, Buchs, Switzerland) for 24 h acted as the positive control for the (pro-)inflammatory proteins tested.
Statistical analysis
Data are presented as the mean ± standard error of the mean (SEM). Statistical analysis was performed using GraphPad Prism 5 (GraphPad Software Inc., La Jolla, CA). A parametric one-way analysis of variance (ANOVA) followed by Bonferroni post-hoc test was performed. Results were considered significant if p < 0.05.
Results
Nebulisation of MWCNTs and deposition characterisation
Different concentrations of MWCNTs (25, 125 and 250 µg/ml) were initially aerosolised using the ALICE system. Subsequently, the morphology of the deposited MWCNTs was examined via conventional TEM. Electron micrographs revealed the typical tubular structure of MWCNTs ( and Supplementary Figure 1A–C). Qualitative analysis of the TEM complimented with qualitative analysis of these images via ImageJ software showed that the deposited MWCNTs had an average length of 3.4 ± 2.2 µm and a diameter of 30 ± 10.3 nm (). These values were similar to the size dimensions of the stock suspension (length: 1–10 µm, width: 5–30 nm; Clift et al., Citation2011a), indicating that the aerosolisation process did not result in the fracture of the MWCNTs.
Figure 1. Morphology and size distribution of deposited MWCNTs. (A) Transmission electron microscopy (TEM) images of 125 μg/ml MWCNTs deposited on TEM grids by using the ALICE system (scale bar: 5 μm). (B) Histogram represents the length distribution (in μm) of nebulised MWCNTs.
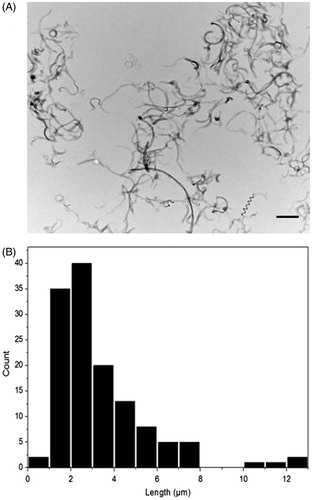
The deposition of MWCNTs was quantified using the online QCM during the entire nebulisation process. The QCM results combined with the TEM images showed that the ALICE revealed a dose dependent, reproducible and controlled deposition of MWCNTs (Supplementary Figure 1D). More specifically, the average deposited values of the different applied MWCNTs concentrations (25, 125 and 250 µg/ml) were 0.14, 0.2 and 0.39 µg/cm2 respectively. In addition, DQ12 revealed an average deposited dose of 0.20 µg/cm2 (Supplementary Figure 2). To highlight the accuracy of the QCM in measuring the deposition, it is important to mention that the negative control (ultrapure H2O) was below the detection limit (0.09 μg/cm2) of the system.
QCM measurements revealed similar deposition for the MWCNTs in both repeated scenarios with average deposited value of 1.15 µg/cm2. The corresponding dose dependent deposition is also being supported by the TEM micrographs indicating the efficiency of the system for repeated nebulisations ().
Figure 2. MWCNTs distribution and deposition after acute and repeated exposures. TEM images of deposited MWCNTs after (A) single exposure (B) one day and (C) three day repeated exposure (scale bar: 5 μm). (D) Graph represents the deposited values (µg/cm2) of MWCNTs after single and repeated exposures as measured by the QCM (n = 3).
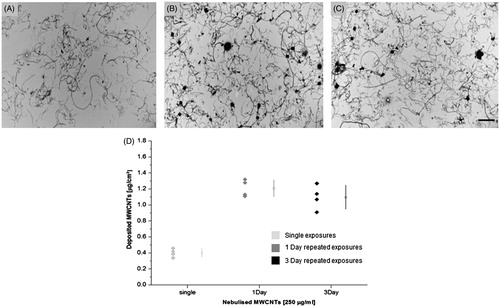
Cellular uptake of MWCNTs
TEM micrographs of the triple co-culture after the three day repeated exposure () showed that MWCNTs were found in all cell types tested (MDM, A549 and MDDC). Mostly, MWCNTs were observed to be localised within vesicular structures (), although there were examples whereby MWCNTs were seen to be present in the cells cytosol (images not shown). Interestingly, although MWCNTs were observed in almost every cell analyzed, the majority of MWCNTs were found to be present inside MDM both as agglomerates and as single tubes. Single MWCNTs were also found within A549 cells. In the case of the one day repeated exposure, MWCNTs were predominantly detected in MDM as well as in small amount within A549 cells (Supplementary Figure 3). Similar observations were also observed following single MWCNTs exposures at 0.39 µg/cm2 (Supplementary Figure 3). Generally, in both single and one day repeated exposure a much lower CNT load per cell was observed in comparison to the three day repeated exposure scenario.
Figure 3. MWCNTs uptake in the triple cell co-culture after three day repeated exposure. TEM micrograph (A) of MDM exposed to MWCNTs repeatedly for three days. Higher magnification image of the MDM (A′) revealed the presence of MWCNTs (white arrows) inside of vesicles. Lower magnification image of A549 cell (B) exposed to MWCNTs for three days. Higher magnification micrograph of the epithelial cell (B′) showing MWCNTs present inside membrane bound compartments. MDDC exposed to MWCNTs (C) after three day repeated exposure at a low magnification. MWCNTs internalised by the MDDC and localizing within a vesicular structure (C′). White arrows indicate the position of MWCNTs. Images A–C scale bars: 5 µm. Images A′–C′ scale bars: 500 nm.
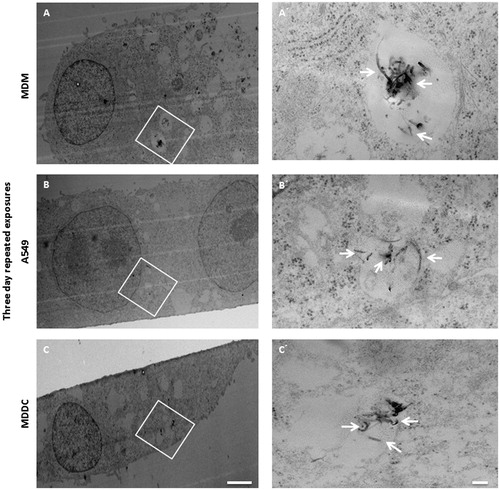
Cytotoxicity and lung cell morphology
Repeated exposures of MWCNTs did not show evidence of increased cytotoxicity in either scenario (). Similar results were gained for cells exposed repetitively to DQ12. Subsequently, single exposure of MWCNTs did not induce any cytotoxic reactions at any of the concentrations tested (A′ and Supplementary Figure 4(A)). Potential effects on cells exposed to Pluronic F127 were also examined in order to detect if the dispersant itself can influence cell functionality. Pluronic did not elicit cytotoxicity neither in single nor in repeated exposure scenarios ().
Figure 4. Cytotoxicity and cellular morphology, following repeated or single exposures. Cytotoxicity as estimated by quantification of LDH release in cell culture medium after (A) repeated and (A′) single exposures (shown relative to negative control). Confocal laser scanning microscopy images of triple cell-co-cultures exposed (B) repetitively and (C) single time to 0.39 µg/cm2 MWCNTs (scale bars: 20 μm). Red color shows F-actin (cytoskeleton), blue color shows DNA (cell nuclei). Data are presented as the mean ± standard error of the mean (SEM) (n = 3). Dashed lines (- - - ) represent the level of the negative control. *Indicates statistical significance compared to the negative control (p < 0.05), for the single and one day repeated exposure. #Represents a significant increase (p < 0.05) compared to the negative control for the three day exposure scenario.
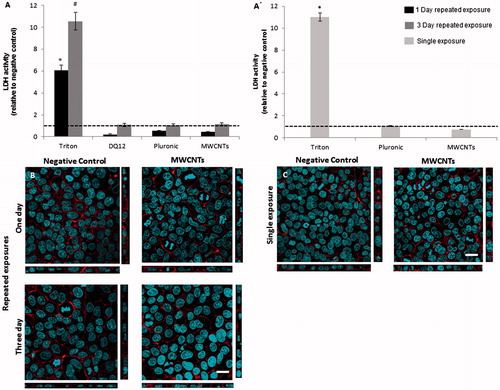
Possible alterations in cell morphology, i.e. F-actin cytoskeleton and cell nuclei, due to single and repeated MWCNTs exposures were analyzed by LSM. In accordance to the cytotoxicity results, no morphological changes were observed in cells exposed repetitively to MWCNTs for one or three days, when compared to the negative control (). Similarly, single exposures at different nanofibre concentrations did not affect cell morphology ( and Supplementary Figure 4C). Additionally, no alterations were observed in cells exposed to Pluronic F127 at 160 ppm (Supplementary Figure 5).
Oxidative stress
One day repeated exposures of triple co-cultures to MWCNTs did not induce oxidative stress, compared to the negative control. However, cells exposed repeatedly to MWCNTs for three days did show a decrease in GSH levels, although not statistically significant (p > 0.05) (). Similar effects were also observed for repeated DQ12 exposures, which also showed no statistical significance, yet the biologically significance should not be underestimated. Similar to the findings for the repeated exposures, single exposures did not elicit significant effects on GSH levels within 24 h ( and Supplementary Figure 4B). Interestingly, despite not being statistically significant (p < 0.05), a biologically relevant decrease in intracellular reduced GSH was observed following exposure of Pluronic F127 in both repeated and single exposure scenarios.
Figure 5. Biochemical response of triple co-cultures after repeated and single exposures to MWCNTs. Oxidative stress status (A) after repeated MWCNTs exposures either one (black) or three days (grey) and (A′) after single CNT exposure (light grey). Quantification of Interleukin 1-beta (IL-1β), after repeated (B) as well as single (B′) cell treatment. Tumor necrosis factor α (TNF-α) release, following (C) repeated or (C′) single MWCNTs aerosolisations. Interleukin 8 (IL-8) secretion 24 h after (D) repeated or (D′) acute exposures. Dashed lines (- - -) represent the level of the negative control. Data are presented as the mean ± standard error of the mean (SEM) of at least three independent experiments. Values were considered significantly different compared to the negative control with p < 0.05 (*single and one day, #three day exposure).
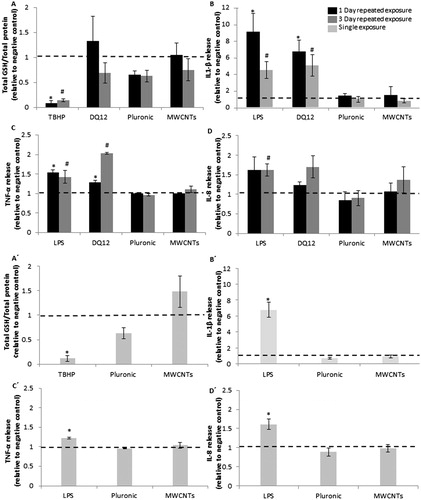
(Pro-)Inflammatory response
Repeated exposure of MWCNTs in both scenarios did not induce any significant effect on IL-1β and TNF-α secretion nor did they elicit an increase in IL-8 compared to control (). Similar effects were also noted following single MWCNT exposures ( and Supplementary Figures 4D–F). As expected though, cells exposed repeatedly to DQ12 did show, in both the one and three day repeated exposure settings, a significant increase (p < 0.05) in the release of both IL-1β and TNF-α, as well as a biological relevant increase (p = 0.056 for the three day repeated exposure) in the secretion of IL-8, cells exposed to Pluronic F127 also showed no alteration in the immune response in both single and repeated exposure scenarios ().
Discussion
Due to their exceptional physicochemical characteristics and the increased interest for potential applications in the industrial and biomedical field, CNTs are nowadays being produced on a large scale, thus potential human exposure to these unique nanomaterials increases (Donaldson et al., Citation2006). However, their close resemblance to other fibers regarding their high aspect ratio and biopersistence heightens public concerns on the widespread use of these materials. Due to the inevitable exposure of CNTs to humans, owing to their use in such applications, it is thus imperative to gain an understanding into how this nanomaterial interacts with the human body.
In this regard, the aim of this study was to (i) realistically mimic inhalation of CNT in vitro by using a well-established and reliable exposure system to expose lung cell cultures cultivated at the ALI to MWCNTs aerosols and to (ii) compare single MWCNTs exposures to repeated exposures.
Recently, it was demonstrated that the ALICE, as an efficient nebulisation system for spherical NPs (Lenz et al., Citation2009), is also applicable for the aerosolisation of fibre shaped cellulose nanocrystals (CNCs) (Endes et al., Citation2013, 2014). Therefore, in order to have a more realistic system mimicking the inhalation of MWCNTs we have used the ALICE to nebulise well-characterised and dispersed MWCNTs (Thurnherr et al., Citation2009). Using this approach it was possible to show a reproducible, spatial homogeneous and dose-dependent deposition on the cell surface. Moreover, visualisation and characterisation of the deposited MWCNTs after the nebulisation process, in comparison with the characterisation of the stock suspension showed no damage to be caused to the morphology of CNTs during the aerosol production. Consequently, the ALICE can be considered, in addition to CNCs, as an efficient method in mimicking the inhalation of nanofibres in the form of MWCNTs.
The MWCNTs used in this study are among today’s most highly produced CNT materials and thus require immediate knowledge on the potential adverse effects they can induce by inhalation. MWCNTs have been used in other studies where they were reported to induce cytotoxicity, genotoxicity and (pro-)inflammatory responses (Johnston et al., Citation2010). However, in those studies, MWCNTs were applied in suspension. One drawback of the suspension exposure is that the deposited dose of the material on the cell surface cannot be easily determined but requires complicated computational models (Hinderliter et al., Citation2010; Teeguarden et al., Citation2007). Another limitation is that the biological response of particles could be affected by the aggregation of nanoparticles and the interactions between them and the cell culture medium. In this study, the use of a sophisticated 3D model of the epithelial airway barrier cultured at the ALI allowed for the direct exposure of cells to an aerosol, thus overcoming such artefacts.
The deposited doses for the current study were selected to be in the range of 0.24–2.4 µg/cm2 for occupationally relevant doses recently reported in studies in accordance to the permissible exposure limit of 1 μg/m3 suggested by NIOSH (NIOSH, Citation2013; Porter et al., Citation2010; Siegrist et al., Citation2014). Sargent et al. (Citation2014) have performed an inhalation study with mice and exposed them with 5 mg/m3 MWCNTs for 5 h per day, 5 d a week for 15 d and calculated a total deposited material concentration in the lungs to 0.06 µg/cm2 mimicking a two week human occupational exposure scenario. Another study has modelled the alveolar mass retention of a full working lifetime exposure to 1 mg/m3 aerosol concentration of CNT is ranging from 12.4 to 46.5 µg/cm2 (Gangwal et al., Citation2011). In summary therefore, doses used in the present study adequately reflect human exposure to MWCNT over several weeks.
The testing strategy for single inhalation exposures was previously validated by Endes et al. (Citation2014), in terms of biological sensitivity by applying DQ12 as a positive particulate aerosol control for (pro-)inflammatory responses. DQ12 demonstrated the applicability of ALICE nebulisations by causing significant (pro-)inflammatory reactions when exposed to the triple cell co-culture at the air–liquid interface. These findings highlight the effectiveness of the presented in vitro systems (i.e. combination of the ALICE and 3D co-culture model) being used.
Prior to assessing the potential cellular impact of MWCNTs, it is essential to study the CNT–cell interaction. Investigation via conventional TEM revealed that following single exposure, MWCNTs were detected mostly as single tubes inside cellular vesicles, predominantly within MDM, but also in the epithelial (A549) cells. These results are in support of the findings of Gasser et al. (Citation2012) who also reported that MDM actively internalise MWCNTs as agglomerates and single tubes located in cytoplasm as well as inside vesicles after 30 μg/ml exposure in suspension. In another recent study, MWCNTs coated with Pluronic F127 were also internalised by A549 cells after suspension exposure (Ali-Boucetta et al., Citation2011). Moreover, Clift et al. (Citation2011a) showed that in a similar triple cell co-culture model (where the 16HBe14o- bronchial epithelial cell line was used) after MWCNTs 30 μg/ml exposure in suspension, that the CNTs where only observed in vesicles inside MDM and not in the epithelial cells or MDDC.
Observation of the cellular biochemical response following acute exposure to different concentrations of MWCNTs showed that no cytotoxic reactions or no changes in the cell morphology occurred. These findings support those recently reported by Clift et al. (Citation2014), when comparing the effects of MWCNTs to different submerged cell culture systems, including the triple co-culture of the epithelial airway barrier. The lack of any cytotoxic response also supports Thurnherr et al. (Citation2009), who showed that the same material caused no apoptosis or necrosis when applied in suspension to Jurkat A3 human leukemic T cells after a 24 h exposure to 30 µg/ml.
Focusing on the ability of MWCNTs to cause inflammation at different concentrations, no effect was observed in the release of (pro-)inflammatory mediators as well as in the intracellular antioxidant GSH compared to the control. Pulskamp et al. (Citation2007) also reported that MWCNTs did not induce (pro-)inflammatory mediators TNF-α and IL-8 in NR8383 and A549 monocultures at concentrations of 5–100 μg/ml. In contrary, Clift et al. (Citation2014) have shown a significant (pro-)inflammatory response with increased production of TNF-α, in triple cell co-cultures cultured in suspension, at concentrations up to 20 µg/ml. Additionally, Ye et al. (Citation2009) illustrated that MWCNTs treatment in monocultures of A549 and BEAS-2A epithelial cells caused changes in IL-8 gene expression, in a dose dependent manner (25–150 μg/ml). The differences in the biological response feature the influence of the exposure method and the biological system used on the CNT characteristics, which can strongly affect cells sensitivity.
It is important to note that the effects observed are relevant to the MWCNTs themselves and not due to the surfactant coating. Clift et al. (Citation2014) showed that Pluronic F127 elicited a significant cytotoxicity and (pro-)inflammatory response only in high doses over 1000 ppm. Recent studies also reported that well dispersed SWCNTs pre-coated with Pluronic showed minimal toxicity compared to aggregated suspensions (Mutlu et al., Citation2010). Interestingly, it was observed that the viability and the functionality of cells were not influenced when exposed to Pluronic F127 (160 ppm), while the levels of intracellular GSH elucidate a notable depletion, which is not observed in case of cells exposed to CNTs. An explanation might be, although the initial concentration of Pluronic (160 ppm) added to CNT solution is known (and was used for testing), it is not possible to know the exact Pluronic concentration that remains in suspension after the preparation process. Recently, Wang et al. (Citation2013) reported that surfactants such us Pluronic F127 and Pluronic F68 can become highly toxic to cells due to degradation, during the sonication process. Another study has demonstrated that Pluronic F127 in concentrations as low as 0.01% can induce cell apoptosis on mouse cortical neurons, while the presence of MWCNTs can reduce toxicity (Bardi et al., Citation2009). It is possible, that adverse effects on cell survival can be caused by alterations on the regulation of cytoplasmic Ca2+ (Sutachan et al., Citation2006). Thus, further research is needed to fully understand the cellular mechanisms in-play following Pluronic exposure.
It is well-accepted, that humans have to deal with chronic occupational exposure to engineered NPs at the workplace (Erdely et al., Citation2013). There is also increasing evidence from several studies in rodents that CNTs are not rapidly degraded but persist in the body for at least up to 90 d (Lam et al., Citation2004; Warheit et al., Citation2004; Yang et al., Citation2007). Kagan et al. (Citation2010) showed that biodegradation of CNTs by the enzyme neutrophil myeloperoxidase in neutrophils results in less pulmonary inflammation, however the exact half-live of CNTs remains still unknown. So far, most publications concerning MWCNTs toxicity in vitro focus upon acute effects. This is paramount, since the rapid response (such as induction of cytokines/chemokines) is essential knowledge in regards to CNT toxicity. Additionally, for a repeated exposure the cells need to survive for a certain time. It has to be considered however, that the maximum survival for cells in incubators might become a limiting factor, and, in addition, the survival of the cells is very much dependent on the cell type and for cell lines growing at the ALI it has been shown that they only remain in a monolayer conformation until day 3 (Blank et al., Citation2006). Recently, a study was published to assess the chronic exposure scenario using the alveolar type II epithelial cell line A549 in suspension (Thurnherr et al., Citation2011). In this study the long-term effects of MWCNTs were compared to acute effects in lung cells (in suspension) experiments. In addition, conventional TEM micrographs of MWCNTs treated A549 cells after long-term single exposure, revealed the presence of MWCNTs, either as single tubes or small agglomerates, mostly surrounded by membranes and in some occasions free in cytoplasm (Thurnherr et al., Citation2011). TEM analysis was also performed in the present study to unambiguously demonstrate MWCNT-uptake in triple cell co-cultures after repeated exposures. The observations for one day repeated exposures were similar to the single exposures were MWCNTs were mostly observed in MDM as well as in epithelial cells. In the three day repeated scenario CNTs were observed in all cell types (MDM, A549 and MDDC), mostly in vesicular structures (although on some occasions were free in the cytosol), indicating the involvement of phagocytic and/or endocytic uptake pathways. Generally, although the cells were exposed in the same CNT doses (1.15 μg/cm2), in the three day repeated exposure higher CNT uptake was observed compared to the one day scenario, indicating that the cells need more time to internalise CNTs at the selected doses, thus the internalisation is more efficient at three days of exposure.
Thurnherr and colleagues (Citation2011) observed that sub-lethal adverse effects were found to be elicited by acute CNT exposure, however, the long-term presence (over 6 months) and accumulation of this material did not have any major impact on cell viability or functionality. In another recent study, Luanpitpong et al. (Citation2014) showed that chronic exposure of submerged human small airway epithelial cells (SAECs) and bronchial BEAS-2B epithelial cells to single-wall CNTs (SWCNTs) for 6 months at 0.02 µg/cm2, induced the formation of cancer stem cells, which may contribute to CNT tumorigenesis. In the present study, it was reported that repeated exposures in both scenarios did not induce any cytotoxic reactions or any alterations in cell morphology, nor did they elucidate any significant increase in IL-1β, TNF-α or IL-8 secretion compared to controls. Cells exposed repeatedly to MWCNTs for three days, however did show a non-significant decrease in GSH levels (p > 0.05). As with acute exposures (Endes et al., Citation2014), the repeated exposure strategy was validated by repeatedly aerosolizing DQ12 crystalline quartz as a positive inflammatory control. Results showed that DQ12 introduced a statistically significant increase in the secretion of the (pro)-inflammatory proteins IL-1β and TNF-α after both repeated exposure scenarios and consequently a biological relevant increase in IL-8, which clearly indicates that (pro-)inflammatory response can be triggered in the system by classical materials known for their inflammatory properties, such as DQ12 (Clouter et al., Citation2001). These findings further confirm the sensitivity of the in vitro system.
In summary, as most existing studies have focused on acute or long-term effects of a single high dose of MWCNTs and have not considered the consequences of a repeated continuous exposure, a comparison with our in vitro results is limited. The outcome of those studies is that most CNTs elicited inflammation, oxidative stress or caused long-term pathological effects such as granulomas and fibrosis (Helland et al., Citation2007) but due to the different CNT material and suspension procedures used, statements on the biological impact of CNTs in this regard remains an obstacle.
Conclusion
Despite increased investigation into the acute toxicity of CNTs there is a clear need to increase our knowledge on their repeated long-term consequences, in combination with an experimental setup which realistically reflects the physiological exposure conditions in the human lung. In the present study, it was possible for the first time to realistically mimic the inhalation of MWCNTs by using a combination of an advanced lung cell model and air–liquid exposure system, providing an effective alternative to animal testing strategies. In conclusion, this study is a major step further to most in vitro toxicity studies, testing not only the potential acute toxicity of MWCNTs but also their potential adverse effects after repeated exposures and extended exposure times, demonstrating that repeated MWCNT exposures to lung cell cultures at the ALI, elicit a limited biological impact over a three day period.
Supplementary material available online
Supplementary Figures 1-5
Supplemental Material.pdf
Download PDF (763.7 KB)Acknowledgements
The authors would like to thank Dr. Craig Poland, Institute of Occupational Medicine (IOM), Edinburgh, UK for the kind gift of the DQ12 sample to MJDC.
Declaration of interest
The authors report no conflict of interest. The authors alone are responsible for the content and writing of the paper.
This study was supported by grants of the Swiss National Science Foundation (#150737 and 320030_138365), an EMPA internal grant, the Adolphe Merkle Foundation and the University of Fribourg.
References
- Ali-Boucetta H, Al-Jamal KT, Müller KH, Li S, Porter AE, Eddaoudi A, et al. 2011. Cellular uptake and cytotoxic impact of chemically functionalized and polymer-coated carbon nanotubes. Small 7:3230–8
- Bardi G, Tognini P, Ciofani G, Raffa V, Costa M, Pizzorusso T. 2009. Pluronic-coated carbon nanotubes do not induce degeneration of cortical neurons in vivo and in vitro. Nanomedicine 5:96–104
- Blank F, Gerber P, Rothen-Rutishauser B, Sakulkhu U, Salaklang J, De Peyer K, et al. 2011. Biomedical nanoparticles modulate specific CD4+ T cell stimulation by inhibition of antigen processing in dendritic cells. Nanotoxicology 5:606–21
- Blank F, Rothen-Rutishauser B, Gehr P. 2007. Dendritic cells and macrophages form a transepithelial network against foreign particulate antigens. Am J Respir Cell Mol Biol 36:669–77
- Blank F, Rothen-Rutishauser B, Schurch S, Gehr P. 2006. An optimised in vitro model of the respiratory tract wall to study the interaction with particles. J Aerosol Med 19:392–405
- Brandenberger C, Rothen-Rutishauser B, Muhlfeld C, Schmid O, Ferron GA, Maier KL, et al. 2009. Effects and uptake of gold nanoparticles deposited at the air–liquid interface of a human epithelial airway model. Toxicol Appl Pharmacol 242:56–65
- Clift MJ, Endes C, Vanhecke D, Wick P, Gehr P, Schins RP, et al. 2014. A comparative study of different in vitro lung cell culture systems to assess the most beneficial tool for screening the potential adverse effects of carbon nanotubes. Toxicol Sci 137:55–64
- Clift MJ, Foster EG, Vanhecke D, Studer D, Wick P, Gehr P, et al. 2011a. Investigating the interaction of cellulose nanofibers derived from cotton with a sophisticated 3D human lung cell co-culture. Biomacromolecules 12:3666–73
- Clift MJ, Frey S, Endes C, Hirsch V, Kuhn DA, Johnston BD, et al. 2013. Assessing the impact of the physical properties of industrially produced carbon nanotubes upon their interaction with human primary macrophages in vitro. Bionanomaterials 14:239–48
- Clift MJ, Gehr P, Rothen-Rutishauser B. 2011b. In vitro testing for nanotoxicology: a valid alternative? Arch Toxicol 85:723–31
- Clouter A, Brown D, Höhr D, Borm P, Donaldson K. 2001. Inflammatory effects of respirable quartz collected in workplaces versus standard DQ12 quartz: particle surface correlates. Toxicol Sci 63:90–8
- De la Zerda A, Zavaleta C, Keren S, Vaithilingam S, Bodapati S, Liu Z, et al. 2008. Carbon nanotubes as photoacoustic molecular imaging agents in living mice. Nat Nanotechnol 3:557–62
- Donaldson K, Aitken R, Tran L, Stone V, Duffin R, Forrest G, Alexander A. 2006. Carbon nanotubes: a review of their properties in relation to pulmonary toxicology and workplace safety. Toxicol Sci 92:5–22
- Endes C, Müller S, Schmid O, Vanhecke D, Foster EJ, Petri-Fink A, et al. 2013. Risk assessment of released cellulose nanocrystals – mimicking inhalatory exposure. J Phys: Conf Ser 429:012008
- Endes C, Schmid O, Kinnear C, Müller S, Camarero Espinosa S, Vanhecke D, et al. 2014. An in vitro testing strategy towards mimicking the inhalation of high aspect ratio nanoparticles. Part Fibre Toxicol 11:40
- Erdely A, Dahm M, Chen BT, Zeidler-Erdely PC, Fernback JE, Birch ME, et al. 2013. Carbon nanotube dosimetry: from workplace exposure assessment to inhalation toxicology. Part Fibre Toxicol 10:53
- Gangwal S, Brown JS, Wang A, Houck KA, Dix DJ, Kavlock RJ, Hubal EA. 2011. Informing selection of nanomaterial concentrations for ToxCast in vitro testing based on occupational exposure potential. Environ Health Perspect 119:1539–46
- Gasser M, Wick P, Clift MJ, Blank F, Diener L, Yan B, et al. 2012. Pulmonary surfactant coating of multi-walled carbon nanotubes (MWCNTs) influences their oxidative and pro-inflammatory potential in vitro. Part Fibre Toxicol 9:17
- Health NIOSH. 2013. Current Intelligence Bulletin 65. Occupational exposure to carbon nanotubes and nanofibres. Cincinnati, OH: U.S. Department of Health and Human Services, Centers for Disease Control, National Institute for Occupational Safety and Health, DHHS (NIOSH) 2013-145
- Helland A, Wick P, Koehler A, Schmid K, Som C. 2007. Reviewing the environmental and human health knowledge base of carbon nanotubes. Environ Health Perspect 115:1125–31
- Herzog F, Clift MJ, Piccapietra F, Behra R, Schmid O, Petri-Fink A, Rothen-Rutishauser B. 2013. Exposure of silver-nanoparticles and silver-ions to lung cells in vitro at the air–liquid interface. Part Fibre Toxicol 10:11
- Hinderliter PM, Minard KR, Orr G, Chrisler WB, Thrall BD, Pounds JG, Teeguarden JG. 2010. ISDD: a computational model of particle sedimentation, diffusion and target cell dosimetry for in vitro toxicity studies. Part Fibre Toxicol 7:36
- Johnston HJ, Hutchison GR, Christensen FM, Peters S, Hankin S, Aschberger K, Stone V. 2010. A critical review of the biological mechanisms underlying the in vivo and in vitro toxicity of carbon nanotubes: the contribution of physico-chemical characteristics. Nanotoxicology 4:207–46
- Kagan VE, Konduru NV, Feng W, Allen BL, Conroy J, Volkov Y, et al. 2010. Carbon nanotubes degraded by neutrophil myeloperoxidase induce less pulmonary inflammation. Nat Nanotechnol 5:354–9
- Kasai T, Gotoh K, Nishizawa T, Sasaki T, Katagiri T, Umeda Y, et al. 2014. Development of a new multi-walled carbon nanotube (MWCNT) aerosol generation and exposure system and confirmation of suitability for conducting a single-exposure inhalation study of MWCNT in rats. Nanotoxicology 8:169–78
- Kostarelos K, Bianco A, Prato M. 2009. Promises, facts and challenges for carbon nanotubes in imaging and therapeutics. Nat Nanotech 4:627–33
- Lam CW, James JT, McCluskey R, Hunter RL. 2004. Pulmonary toxicity of singlewall carbon nanotubes in mice 7 and 90 days after intratracheal instillation. Toxicol Sci 77:126–34
- Lenz AG, Karg E, Lentner B, Dittrich V, Brandenberger C, Rothen-Rutishauser B, et al. 2009. A dose-controlled system for air–liquid interface cell exposure and application to zinc oxide nanoparticles. Part Fibre Toxicol 6:32
- Luanpitpong S, Wang L, Castranova V, Rojanasakul Y. 2014. Induction of stem-like cells with malignant properties by chronic exposure of human lung epithelial cells to single-walled carbon nanotubes. Part Fibre Toxicol 11:22
- Ma-Hock L, Treumann S, Strauss V, Brill S, Luizi F, Mertler M, et al. 2009. Inhalation toxicity of multiwall carbon nanotubes in rats exposed for 3 months. Toxicol Sci 112:468–81
- Maynard AD, Baron PA, Foley M, Shvedova AA, Kisin ER, Castranova V. 2004. Exposure to carbon nanotube material: aerosol release during the handling of unrefined single-walled carbon nanotube material. J Toxicol Environ Health 67:87–107
- McKinney W, Chen B, Frazer D. 2009. Computer controlled multi-walled carbon nanotube inhalation exposure system. Inhal Toxicol 21:1053–61
- Mercer RR, Scabilloni JF, Hubbs AF, Battelli LA, McKinney W, Friend S, et al. 2013. Distribution and fibrotic response following inhalation exposure to multi-walled carbon nanotubes. Part Fibre Toxicol 10:33
- Monteiller C, Tran L, MacNee W, Faux S, Jones A, Miller B, Donaldson K. 2007. The pro-inflammatory effects of low-toxicity low-solubility particles, nanoparticles and fine particles, on epithelial cells in vitro: the role of surface area. Occup Environ Med 64:609–15
- Mutlu GM, Budinger GR, Green AA, Urich D, Soberanes S, Chiarella SE, et al. 2010. Biocompatible nanoscale dispersion of single walled carbon nanotubes minimizes in vivo pulmonary toxicity. Nano Lett 10:1664–70
- Oberdörster G, Oberdörster E, Oberdörster J. 2005. Nanotoxicology: an emerging discipline evolving from studies of ultrafine particles. Environ Health Perspect 113:823–39
- Oberdorster G, Stone V, Donaldson K. 2007. Toxicology of nanoparticles: a historical perspective. Nanotoxicology 1:2–25
- Pauluhn J. 2010. Subchronic 13-week inhalation exposure of rats to multiwalled carbon nanotubes: toxic effects are determined by density of agglomerate structures, not fibrillar structures. Toxicol Sci 113:226–42
- Piccinno F, Gottschalk F, Seeger S, Nowack B. 2012. Industrial production quantities and uses of ten engineered nanomaterials in Europe and the world. J Nanopart Res 14:1109
- Porter DW, Hubbs AF, Chen BT, McKinney W, Mercer RR, Wolfarth MG, et al. 2013. Acute pulmonary dose-responses to inhaled multi-walled carbon nanotubes. Nanotoxicology 7:1179–94
- Porter DW, Hubbs AF, Mercer RR, Wu N, Wolfarth MG, Sriram K, et al. 2010. Mouse pulmonary dose- and time course-responses induced by exposure to multi-walled carbon nanotubes. Toxicology 269:136–47
- Pulskamp K, Diabate S, Krug HF. 2007. Carbon nanotubes show no sign of acute toxicity but induce intracellular reactive oxygen species in dependence on contaminants. Toxicol Lett 168:58–74
- Robertson J. 2004. Realistic applications of CNTs. Mater Today 7:46–52
- Robock K. 1973. Standard quartz DQ12 <5 µm for experimental pneumoconiosis research projects in the Federal Republic of Germany. Am Occup Hyg 16:63–6
- Rothen-Rutishauser B, Mueller L, Blank F, Brandenberger C, Muehlfeld C, Gehr P. 2008. A newly developed in vitro model of the human epithelial airway barrier to study the toxic potential of nanoparticles. ALTEX 25:191–6
- Rothen-Rutishauser BM, Kiama SG, Gehr P. 2005. A three-dimensional cellular model of the human respiratory tract to study the interaction with particles. Am J Respir Cell Mol Biol 32:281–9
- Ryman-Rasmussen JP, Cesta MF, Brody AR, Shipley-Phillips JK, Everitt JI, Tewksbury EW, et al. 2009. Inhaled carbon nanotubes reach the sub-pleural tissue in mice. Nat Nanotechnol 4:747–51
- Sargent LM, Porter DW, Staska LM, Hubbs AF, Lowry DT, Battelli L, et al. 2014. Promotion of lung adenocarcinoma following inhalation exposure to multi-walled carbon nanotubes. Part Fibre Toxicol 11:3
- Schneider CA, Rasband WS, Eliceiri KW. 2012. NIH image to ImageJ: 25 years of image analysis. Nat Methods 9:671–5
- Siegrist KJ, Reynolds SH, Kashon ML, Lowry DT, Dong C, Hubbs AF, et al. 2014. Genotoxicity of multi-walled carbon nanotubes at occupationally relevant doses. Part Fibre Toxicol 11:6
- Snyder-Talkington BN, Schwegler-Berry D, Castranova V, Qian Y, Guo NL. 2013. Multi-walled carbon nanotubes induce human microvascular endothelial cellular effects in an alveolar-capillary co-culture with small airway epithelial cells. Part Fibre Toxicol 10:35
- Sutachan JJ, Montoya GJ, Xu F, Chen D, Blanck TJ, Recio-Pinto E. 2006. Pluronic F-127 affects the regulation of cytoplasmic Ca2+ in neuronal cells. Brain Res 1068:131–7
- Tabet L, Bussy C, Amara N, Setyan A, Grodet A, Rossi MJ, et al. 2009. Adverse effects of industrial multiwalled carbon nanotubes on human pulmonary cells. J Toxicol Environ Health A 72:60--73
- Teeguarden JG, Hinderliter PM, Orr G, Thrall BD, Pounds JG. 2007. Particokinetics in vitro: dosimetry considerations for in vitro nanoparticle toxicity assessments. Toxicol Sci 95:300–12
- Thurnherr T, Brandenberger C, Fischer K, Diener L, Manser P, Maeder-Althaus X, et al. 2011. A comparison of acute and long-term effects of industrial multiwalled carbon nanotubes on human lung and immune cells in vitro. Toxicol Lett 200:176–86
- Thurnherr T, Su DS, Diener L, Weinberg G, Manser P, Pfänder N, et al. 2009. Comprehensive evaluation of in vitro toxicity of three large-scale produced carbon nanotubes on human Jurkat T cells and a comparison to crocidolite asbestos. Nanotoxicology 3:319–38
- Van Berlo D, Clift MJD, Albrecht C, Schins RPF. 2012. Carbon nanotubes: an insight into the mechanisms of their potential genotoxicity. Swiss Med Wkly 142:w13698
- Wang L, Luanpitpong S, Castranova V, Tse W, Lu Y, Pongrakhananon V, Rojanasakul Y. 2011. Carbon nanotubes induce malignant transformation and tumorigenesis of human lung epithelial cells. Nano Lett 11:2796--803
- Wang R, Hughes T, Beck S, Vakil S, Li S, Pantano P, Draper RK. 2013. Generation of toxic degradation products by sonication of Pluronic dispersants: implications for nanotoxicity testing. Part Fibre Toxicol 11:6
- Warheit DB, Laurence BR, Reed KL, Roach DH, Reynolds GA, Webb TR. 2004. Comparative pulmonary toxicity assessment of single-wall carbon nanotubes in rats. Toxicol Sci 77:117–25
- Wick P, Manser P, Limbach LK, Dettlaff-Weglikowska U, Krumeich F, Roth S, et al. 2007. The degree and kind of agglomeration affect carbon nanotube cytotoxicity. Toxicol Lett 168:121–31
- Yang ST, Guo W, Lin Y, Deng XY, Wang HF, Sun HF, et al. 2007. Biodistribution of pristine single-walled carbon nanotubes in vivo. J Phys Chem 111:17761–4
- Ye SF, Wu YH, Hou ZQ, Zhang QQ. 2009. ROS and NF-kappaB are involved in upregulation of IL-8 in A549 cells exposed to multi-walled carbon nanotubes. Biochem Biophys Res Commun 379:643–8
- Zavaleta C, De la Zerda A, Liu Z, Keren S, Cheng Z, Schipper M, et al. 2008. Noninvasive Raman spectroscopy in living mice for evaluation of tumor targeting with carbon nanotubes. Nano Lett 8:2800–5
- Zhang X, Li Q, Holesinger TG, Arendt PN, Huang J, Kirven PD, et al. 2007. Ultrastrong, stiff, and lightweight carbon-nanotube fibers. Adv Mater 19:4198–201
- Zhang Y, Bai Y, Yan B. 2010. Functionalized carbon nanotubes for potential medicinal applications. Drug Discov Today 15:428–35