Abstract
Iron oxide nanoparticles coated with gelatin trough two-step desolvation method and characterized. SEM analyse showed that the nanoparticles are spherical and TEM image showed that the iron oxide nanoparticles encapsulated with gelatin. Also FTIR, TG and magnetization analyses exhibited that iron oxide nanoparticles encapsulated as well. Drug loading by adsorption studied under various conditions as different temperature, contact time and initial cisplatin concentration. Also, Langmuir, Freundlich and Dubinin-Raduskevich adsorption isotherm curves were constracted and constants were calculated. In vitro drug release was performed at pH 5 and 7.4 and hyperthermic drug release investigated at 42°C and compared with non-hyperthermic drug release.
Introduction
Gelatin is a soluble protein compound obtained by partial hydrolysis of collagen, the main fibrous protein constituent in bones, cartilage and skins; therefore, the source, age of the animal and type of collagen are all intrinsic factors influencing the properties of gelatins. Two types of gelatin obtainable, depending on the pre-treatment procedure and are known commercially as type A gelatin (isoelectric point at pH ˜8–9) and type B gelatin (isoelectric point at pH ˜4–5) obtained under acidic and alkaline pre-treatment conditions, respectively (Gomez-Guillen et al. Citation2011). Gelatin is a raw material to form nanoparticles beacuse of its biocompatibility and biodegradability. Furthermore, the many functional groups of gelatin, including carboxyl and amine, make it an ideal candidate for surface modification to conjugate biomolecules (Tseng et al. Citation2007).
Approximately 1.6 million new cases of lung cancer are diagnosed each year throughout the world. In many countries, the mortality related to lung cancer continues to rise. The outcomes for patients with all stages of lung cancer have improved in recent years. The use of systemic therapy in conjunction with local therapy has led to improved cure rates in both resectable and unresectable patient groups. For patients with advanced stage disease, modest but real improvements in overall survival and quality of life have been achieved with systemic chemotherapy (Ramalingam et al. Citation2011). Cisplatin is among the most widely used and broadly active cytotoxic anti-cancer drug. Cisplatin enters the cells by mechanism that remain incompletely defined. Once inside cells, cisplatin undergoes aquation hydrolysis to form identical active species (Perez Citation1998). Cisplatin reacts with many potential target molecules, including genomic DNA, RNA and protein. Approximately 1% of intracelluler cisplatin reacts with genomic DNA yielding a variety of intra- and inter-strand mono-adducts and cross-links, the most common being an intra-strand cross-link between adjacent guanines. Considerable evidence indicates that cisplatin can kill cells by apoptosis. Internucleosomal DNA cleavage and ultrastructural change characteristics of apoptosis have been observed following cisplatin in murine L1210 leukaemia and Chinese hamster ovary cell lines and in proliferating rat hepatoma cells, but not in proliferating thymocytes (Perez Citation1998). Nevertheless, its high anti-tumor activity is frequently hampered by relevant side effects, in particular nephrotoxicity, neurotoxicity and ototoxicity. Great efforts have been made to reduce these side effects by means of biological and pharmacological strategies also aiming to permit higher doses of drug, which could be of particular benefit from a therapeutic point of view (Cafaggi et al. Citation2007).
The use of stimuli-responsive nanocarriers offers an interesting opportunity for drug and gene delivery where the delivery system becomes an active participant, rather than passive vehicle, in the optimization of theraphy. For specific targeting, a possible strategy is physical targeting of drugs and genes by external stimuli (magnetic field, ultrasound, light and heat). An interesting example is targeted delivery of iron oxide nanoparticles using magnetic field. Upon the administration, the drug-immobilized magnetic carrier can accumulate at targeted site under the direction of external magnetic field (Ganta et al. Citation2008). For targeted drug delivery system, another approach was made by Babincová M. and collegues. In their work, a novel platform has been developed for combined cancer chemotheraphy and hyperthermia based on iron oxide nanoparticles. The capabilities of this system for heating and controlled drug release were investigated (Babincova et al. Citation2008).
The aims of this work include synthesis of magnetic gelatin nanoparticles, loading cisplatin onto nanoparticles by adsorption and investigating the adsorption parameters on drug loading. By designing this drug carrier system, we intend to control nanopaticular system by external magnetic field in order to target tumor region and to release the drug at this site in combination with hyperthermia and pH response resulting with the increased therapeutic index and decreased side effects.
Materials and method
Materials
Gelatin type B was purchased from Sigma, glutaraldehyde (GA) was obtained from Merck and cisplatin (CDDP) was supplied commercially. Iron oxide nanoparticles’ (IONPs) were synthesized as previously reported (Doan et al. Citation2008). All the other reagents were of analytical grade.
Synthesis of gelatin nanoparticles by two-step desolvation method
Gelatin nanoparticles (GNPs) were synthesized by the combination of the methods described by (Gaihre et al. Citation2008, Coester et al. Citation2006, Ofokansi et al. Citation2010). Briefly, 5% gelatin solution was prepared by dissolving gelatin type B at 37°C. 1 mL of acetone was added rapidly into the 1 mL of gelatin solution. The resulting precipitate was collected and redissolved at 37°C. 2 mL of acetone was added dropwise (1 mL/min) to the redissolved gelatin solution at pH 12. The resulting nanoparticles were cross-linked by adding 12 μL GA (4%) and stirring at 480 rpm for 24 hours. Finally, resulting nanoparticles were washed with distilled water for three times by centrifugation at 13,000 rpm to remove acetone and residual GA.
Effect of acetone flow rate was investigated to estimate GNPs’ formation yield. For this purpose, 0.7–1.7 mL/minute acetone flow rate was varied and the procedure was applied as mentioned above. Non-desolvated protein in supernatants was defined via modified Lowry protein measurement assay (KomsaPenkova et al. Citation1996) and desolvation efficiency was calculated using the Formula 1.
Synthesis of magnetic gelatin nanoparticles (MGNP's)
Magnetic gelatin nanoparticles (MGNPs) were prepared by two-step desolvation as previously described with a minor modification in the second desolvation step. 1 mL of acetone was added rapidly to 1 mL of 5% gelatin solution. The resulting precipitate was redissolved with 0.5 mL ultrapure water at 37°C, pH adjusted to 12 and 0.5 mL (4 mg/mL) IONP's solution added. Then, 2 mL of acetone was added dropwise (1 mL/min) to redissolved IONP-dispersed gelatin solution. The resulting nanoparticles were cross-linked by adding 12 μL GA (4%) and stirred at 480 rpm for 24 hours. Finally, resulting nanoparticles were washed with distilled water for three times by centrifugation at 13,000 rpm to remove acetone and residual GA.
Adsorption of CDDP onto the MGNP's
CDDP loading onto the MGNPs’ was achieved according to Tseng et al. with a minor modification (Ding et al. Citation2011). Cisplatin solution diluted to 350 μg/mL concentration with ultrapure water and MGNP's dissolved in this drug solution. It was allowed to react at 37°C for 24 hours. After incubation, samples were centrifugated at 13,000 rpm. Unbound CDDP amount was measured spectrophotometrically using the method described by Anilanmert et al. (Citation2001). CDDP-loaded MGNPs were named as CDDP-MGNP's. CDDP adsorption yield was calculated using the Formula 2.
Adsorption isotherms and thermodynamic modelling
The experiments were performed to observe the effect of concentration and contact time at constant pH and temperature. MGNPs were taken in 2 mL eppendorf tubes containing 1.5 mL each of different CDDP concentrations. These eppendorf tubes were then shaken in hot water bath at 25°C for 24 hours. Then, samples were centrifugated at 13,000 rpm and unbound CDDP amount was calculated. ΔG of the adsorption was calculated by using Langmuir constant Q0. For Dubinin–Raduskhevich isotherm CDDP concentration varied from 50–400 μg/mL, for Freundlich and Langmuir isotherm CDDP concentration varied from 25–400 μg/mL.
Adsorpion isotherms of CDDP were studied. Dubinin-Radushkevich, Freundlich and Langmuir isotherms were applied and constants were calculated.
Characterization
Scanning electron microscope (SEM, Philips XL-30S FEG and FEI Quanta250 FEG) imaging analysis of freeze-dried MGNP's was performed in Izmir Institute of Technology and transmission electron microscopy (TEM, JEOL JEM-2100F 200 kV) was performed in Mugla University. In order to determine the chemical form, dried powder of plain gelatin type B, GNP's, IONP's and MGNP's were examined by Fourier-transform Infrared spectroscopy (FTIR, Perkin Elmer FTIR Spectrum One-B Spectrometer). Thermogravimetical analyses of plain gelatin, GNPs’, IONPs’ and MGNPs’ were performed with Perkin Elmer Diamond TG/DTA in order to compare durability of formulations. Magnetism of IONPs’ and MGNPs’ was estimated using magnetometer (Lake Shore Vibrometric Sample Magnetometer) under 1000 Gauss (G) magnetic field in Izmir Institute of Technology.
In vitro drug release study
The in vitro drug release studies of CDDP-MGNP's were carried out at 37°C in the hot water bath with constant shaking for 24 hours and free CDDP's release was used as a control.
Briefly, CDDP-MGNP's dispersed in PBS (pH 5, 6 and 7.4) was placed in dialysis tubing (12.000 MWCO) and dialysed against 15 mL PBS (pH 5, 6 and 7.4, respectively) for 24 hours. At regular intervals of time, all of the receiver solution were withdrawn and 15 mL solution was placed (Yang et al. Citation2008). Released amount of CDDP was determined from the receiver solution spectrophotometrically and cumulative release was calculated with the Formula 3.
In vitro drug release from CDDP-MGNP's using heat-induced hyperthermia
The in vitro drug release using heat-induced hyperthermia studies of CDDP-MGNP's was carried out at 42°C in the hot water bath with constant shaking for 24 hours and free CDDP's release was used as a control.
Briefly, CDDP-MGNP's dispersed in PBS (pH 5, 6 and 7.4) was placed in dialysis tubing (12.000 MWCO) and dialysed against 15 mL PBS (pH 5, 6 and 7.4, respectively) for 24 hours. At regular interval of time all of the receiver solution were withdrawn and 15 mL solution was placed (Yang et al. Citation2008). Released amount of CDDP was determined from the receiver solution spectrophotometrically and cumulative release was calculated using the Formula 3.
Results and discussion
Synthesis of GNP's by two-step desolvation method and characterization
Desolvation of gelatin with organic solvents followed by cross-linking with GA is a commonly used and simple method for the preparation of GNPs’ (Leo et al. Citation1997, Balthasar et al. Citation2005, Nahar et al. Citation2008). In the present study, gelatin nanoparticles were synthesised by two-step desolvation method. Since, commercial gelatin shows a wide range of molecular weight and the presence of a low molecular weight fraction in the solution gives broad size distribution and aggregation of nanoparticles. Two-step desolvation method allows to remove the low molecular weight fraction in the first desolvation step. The GA concentration and temperature were chosen based on the earlier studies. GA was added as a cross-linking agent to prevent the redissolving of gelatin nanoparticles. According to Gaihre and coworkers, when GA is added to the aqueous dispersion of the composite nanoparticles, the carboxylic acid residues and amine groups react with the cross-linking agent. 12 μL of 4% GA solution was found to be enough for good sized and better stability gelatin nanoparticles (Gaihre et al. 2008). Temperature effect on formulation explained by high viscosity of gelatin in room temperature. Higher temperture was found to be preferable for the formation of GNP's because triple-helical structure of gelatin uncoiles as the temperature arises (Nahar et al. 2008). Gelatine has been used in many studies such as a drug, matrix and nucleotide carrier (Leo et al. Citation1997, Ofokansi et al. Citation2010, Zwiorek et al. Citation2008, Nahar et al. Citation2008). The particle size of designed nanoparticular system must be significiantly smaller than 5 μm, without forming aggregates, to ensure that the particles do not cause an embolism, since the smallest capillaries in the body are 5–6 μm in diameter (Singh and Lillard Citation2009). Also it is important to reach high nanoparticle formation efficieny from starting material.
Gelatin concentration (% w/v), pH and acetone flow rate (ml/min) were evaluated for GNP's. GNP's protein content was determined indirectly by using Modified Lowry curve's equation y = 2.026x with 0.9906 correlation constant.
Desolvation efficiency was above 83 ± 5.0% for 5% concentration gelatin solution which is correlated with the earlier studies. Gelatin is a natural polymer with carboxyl, amine, and amide functional groups present in the polymer chain. So it is expected to be negatively charged in alkaline conditions and to show higher formation efficiency. At pH 12, nanoparticle formation efficiency was about 83.4 ± 6.2. These results demonstrate compliance with earlier studies (Taheri Qazvini and Zinatloo Citation2011, Jahanshahi et al. 2008). Aceton flow rate effect was not a well-researched parameter which is researched in the scope of this study. Desolvating method is a modification of a salting-out technique. In the salting-out, for protein precipitation, organic solvents may be used. As known, with the rapid addition of organic solvent or salting-out agent, hydrophobic sites of proteins emerge and interact with each other rapidly so that proteins precipitate as aggregates suddenly. It means with rapid addition of organic solvent, particle size grows rapidly that size control becomes unavailable. The nanoparticle formation yield was between 79.5–83.3 ± 3.8% which means acetone flow rate does not affect the nanoparticle formation yield. For 0.7, 1.0, 1.3 and 1.7 mL/min flow rates, the sizes were 122.0 ± 5.6, 136.0 ± 4.4, 237.0 ± 7.8 and 234.0 ± 9.3 nm, respectively. Particle size increased with increased acetone flow rate as expected. Based on the results, 1 mL/min of acetone flow rate was chosen owing to both size and nanoparticle formation yield. Results were shown in .
Table I. Acetone flow rate effect on both GNP formation yield and particle size.
Afterwards the optimization of empty GNP's, SEM images were taken. Due to the SEM images, GNP's are homogenous and spherical shaped. TG and FTIR analyses were studied both for plain gelatin and GNP's which are given in the and .
Synthesis of MGNP's and characterization
MGNP's were systhesized as described in section 2.3. According to the Gaihre B. and co-workers study, for effective targeting, the percentage of IONPs in the composite nanoparticles plays an important role and with increasing percentage of IONPs in gelatin solution there is no significiant change in the hydrodynamic size of the nanoparticles (Gaihre et al. 2008). It means, gelatin encapsulates the IONPs with apparent percentage, there is no need to increase the percentage of IONPs. Increasing the IONPs percentage also increases the gelatin solutions’ viscosity which leads to aggregation of gelatin as a gel but not in form of nanoparticles (Gaihre et al. 2008).
Different IONP concentrations were performed in this study and decided the optimum concentration based on the magnetization. The magnetization curves of IONPs’ and optimum MGNPs’ are shown in . IONPs showed 45.3 emu/g response and MGNPs showed 20.2 emu/g response in 0.1 T magnetic field, respectively. The decrease in magnetic response also indicates the encapsulation of IONPs into GNPs. 4 mg/mL IOP's containing MGNPs were chosen based on the magnetization.
FTIR analyses were performed for plain gelatin, GNPs, IONPs and MGNPs. Plain gelatin showed characteristic amide I and II bands at 1635 cm− 1 and 1541 cm− 1, respectively. Similarly, the broad band was due to H-bonded O–H and N–H stretching vibrations occured in the region of 3200–3600 cm− 1. IONP's show characteristic strong peaks between 600 and 500 cm− 1. IONP's, FTIR data showed the characteristic peaks in 627 cm− 1 and 575 cm− 1. MGNPs showed both gelatin's and IONPs characteristic peaks. However, the amide I and II bands in gelatin shifted to 1656–1634 cm− 1 and 1563–1532 cm− 1, respectively in GNPs-MGNPs. The shifting of amide I and II band indicates the electrostatic interaction between amide bond and the IONPs. Furthermore, due to the metalic attachment to O–H groups, O–H bonding extents and the broadness of the 3600–3200 cm− 1 regions band decreases.
Indeed, the organic molecules believed to be chemisorbed into IONPs surface through the carbonyl and amino groups which prevents the leaching of IONPs from nanoparticles and ensures the effective loading (Gaihre et al. 2008).
TG analyse showed major weight loss has occured in two stages for all samples. The first weight loss of all samples is observed at ˜100–150°C due to evaporation of phisically adsorbed water on the surface of samples. The second weight loss for plain gelatin, IONPs, GNPs and MGNPs was observed between 250 and 300°C with 25.39%, 93.49%, 83.07% and 86.91% residue left after thermal degradation, respectively. The nanoparticle formation from plain gelatin increased its thermal stability. Increased thermal stability of GNPs and MGNPs may allow to the thermal processes like drug release with heat-induced hyperthermia.
Also, TEM images of MGNPs showed in . As seen in the figure, IONP's encapsulated in the GNPs succesfully and nanoparticle size is about 300 nm. Internal structure of MGNPs can be visualized because the gelatin molecules can not absorb the electron beam and hence become invisible while IONPs can absorb the electron beam and appear as dark spots.
Adsorption of CDDP onto MGNPs
In present work, adsorption temperature was studied and adsorption time was chosen based on the earlier study (Ding et al. 2011). Maximum adsorption occured at 25°C with 94.6 ± 5.0 yield. CDDP is a small molecule which has metal core and with increasing temperature, owing to the increased molecule motion, the interaction time with MGNPs’ decreases. Also at high temperatures MGNPs may expand due to the swelling property of gelatin and it may result with decreased surface area for adsorption.
Adsorption time were performed at 25°C in the range of 3–48 hours. As seen in the adsorption increased with contact time. With increasing contact time, CDDP molecules have enhanced chance for interaction with MGNPs. Maximum adsorption occured at 48 hours with 94.6 ± 5.0 yield and 62.1 ± 4.9 yield occured at 24 hours. But at 48 hours, there is contamination risk for MGNPs hence 24 hours is chosen for further studies.
The effect of CDDP concentration study was performed at 25°C for 24 hours. As seen in , data revealed that maximum adsorption was occured at 350 μg/mL concentration and above this concentration, desorption was occurred.
Table II. CDDP concentration, contact time and temperature effect on CDDP adsorption onto MGNPs.
Langmuir, Freundlich and Dubinin-Raduskevich isotherms were studied, constants were calculated and isotherm models were given below.
Langmiur adsorption isotherm explaines the monolayer coverage of the sorbate on a sorbent surface at a constant temperature. The assumption is that the forces exerted by chemically unsaturated surface atoms do not extend further than diameter of one sorbed molecule (Ofokansi et al. Citation2010). The linear Langmuir curve's equation is:
Ceq is the concentration of CDDP solution (mol/L) at equilibrium, Qeq is the amount of CDDP adsorbed per unit weight of MGNP (mol/g). Q0 gives the theoretical monolayer adsorption capacity (mol/g) and b is the Langmiur constant which is related with the energy of adsorption (L/mol). A plot of Ceq/Qeq versus Ceq yields a straight line with slope 1/Q0 and intercept b.
To identify the favourability of the adsorption, dimensionless constant seperation factor R is expressed as:
RL value indicates the state of adsorption as follows; RL > 1, unfavourable; RL = 1, linear; 0 < RL < 1, favourable; RL = 0, irreversible (Mittal et al. Citation2008).
In the present study, the theoretical monolayer adsorption capacity was found 40.0 mol/g and Langmiur constant was found 122.2 L/mol. RL value was 0.021 which is between 0 and 1 means the adsorption of CDDP onto MGNPs’ is favourable.
The changes in the standard Gibb's free energy were calculated using Langmuir isotherm constant b. The equation was shown below (Mittal et al. Citation2008)
The negative standard Gibb's free energy values indicate the feasibility of the process and its spontaneous nature. The standard Gibb's free energy determined as − 11.9 kJ/mol. This value represents the adsorption occured spontaneously and process was feasible.
The Freundlich isotherm is an empirical equation based on a heterogenous surface. A linear form of the Freundlich expression will yield the constant KF and n.
Therefore, a plot of lnqeq versus lnCe enables the constant KF and exponent n can be determined. KF can be defined as adsorption of distribution coefficient and represents the quantity of CDDP adsorbed onto MGNPs’ for an equilibrium concentration. The slope 1/n, ranging between 0 and 1 is a measure of adsorpton intensity of surface heterogenity, becoming more heterogeneous as its value gets closer to the zero. If the adsorption intensity, n is less than 1, it indicates that adsorption intensity is good over the entire range of concentration studied, but if the n value is more than 1, it means that adsorption intensity is favourable at high concentration but much less at lower concentration (Ngah and Fatinathan Citation2008).
In our work, KF, n and 1/n values were determined as 0.002 mol1–1/n L1/n g− 1, 2.3 and 0.4, respectively. The value n is more than 1 which means the adsorption intensity is good at high concentrations and the value 1/n indicates that the surface of MGNPs’ is more likely to be heterogenous.
The Dubinin-Raduskevich isotherm does not assume a homogenous surface or constant sorption potential. The linear Dubinin-Raduskevich equation is,
ln qe = ln qm – βϵ2(8)
Where qe is the amount of CDDP adsorbed at equilibrium, β is a constant related to the adsorption energy, qm is the theoretical saturation capacity, ϵ is the Polanyi potential, that is equal to RT(1 + 1/Ce). The slope of the plot of ln qe versus ϵ2 gives β (mol2/kJ2) and the intercept yields the sorption capacity, qm (mol/g) (Akçay et al. Citation2009). The sorption free energy could be further calculated as follows,
E = – (2β)21/2(9)
The E value can be used for estimating the type of adsorption process. Two main types of adsorption may occur: physical and chemical adsorption. In physical adsorption equilibrium is, usually, rapidly attained and easily reversible, because the energy requirements are small (usually no more than 4.2 kJ/mol). The chemical adsorption is specific and involves forces which are much stronger than those in physical adsorption. So the sorption energy for chemical adsorption should be between 8 and 16 kJ/mol which also indicates that the adsorption follows ion-exchange mechanism (Ramalingam et al. 2011, Rastogi et al. Citation2011, Singh and Lillard 2009).
Obtained data revealed that theoritical saturation capacity is 0.0003 mol/g and β is − 0.0041. The value E calculated as 11.0 kJ/mol which is between 8 and 16 kJ/mol. The result shows the adsorption was chemical with ion-exchange mechanism. CDDP adsorption onto MGNPs’ is not easily reversible.
According to the correlation constants ®, the best isotherm model fits to the CDDP adsorption onto MGNPs’ is both Freundlich and Dubinin-Raduskevich isotherm models with 0.9886 and 0.9802 correlation constants, respectively. Both models can be used to explain CDDP adsorption onto MGNP's adsorption process. All the adsorption isotherm graphics can be seen in and constants can be seen in .
Table III. Langmiur, Freundlich and Dubinin-Raduskevich isotherms constants for adsorption of CDDP by MGNPs.
Figure 5. Langmiur isotherm (A), Freundlich isotherm (B) and Dubinin-Raduskevich isotherm (C) of CDDP adsorption.
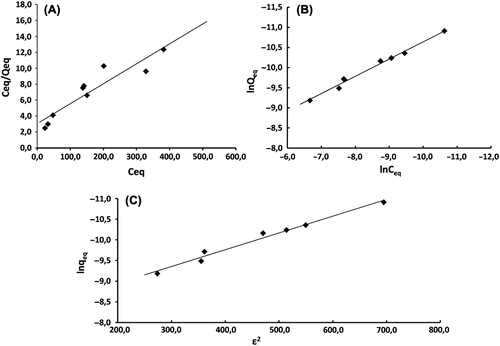
Adsorption process was performed at neutral pH which means the – NH3 groups of cisplatin could be positively charged and –COOH groups of gelatin nanoparticles could be negatively charged. Based on the isotherm constants, adsorption may occur between the –COOH groups of gelatin nanoparticles and the –NH3 groups of cisplatin using ion-exange mechanism.
In vitro drug release studies
The drug release behavior of MGNPs’ was investigated using dialysis membrane in pH 5 and 7.4 PBS buffers both at 37°C and 42°C. shows the release profile of CDDP from MGNPs’ and free CDDP.
Figure 6. (A) CDDP release from MGNPs’ in different pH (10 mM PBS) and (B) free CDDP release in different pH (10 mM PBS).
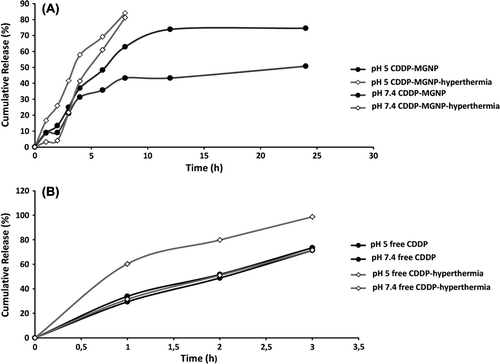
As seen in the the cumulative release of free cisplatin reached 71.4% at pH 7.4 and 73.5% at pH 5 in 3 hours which means free cisplatin release is not pH dependent. On the other hand, the cumulative release of CDDP from MGNPs’ was slower and pH dependent. At pH 5 in 3 hours only the 25% of CDDP was released from MGNPs’ and at the end of the 24 hours 74.6% was released. At pH 7.4 in 3 hours 21.1% of CDDP released from MGNPs’ and 50.7% CDDP released after 24 hours. However, because of the magnetic property of nanoparticles, it is expected that the targeting of MGNPs’ takes 30 minutes (Lubbe et al. Citation2001). Herewith it is much important to compare the first hour of CDDP release profiles at pH 7.4. The data exhibited that in the first hour of CDDP release from MGNPs’ at 7.4 was 9.1% but free CDDP's release was 29.4%. The faster release of CDDP from MGNPs’ at acidic medium can be explained with the surface charge of the MGNPs’. At acidic medium MGNPs’ surface charge expected to be positive which leads the weakening the interaction between CDDP and MGNPs’ (Gaihre et al. Citation2009).
Owing to the swelling properties of gelatin and IOP content of the CDDP-loaded nanoparticles, it is expected to increase the release rate at the hyperthermic temperature (42°C) (Rastogi et al. 2011). After 8 hours, the CDDP release rate from MGNPs’ was 1.3 fold higher at pH 5 compared to the non-hyperthermic media and 1.8 fold higher at pH 7.4, respectively. The increased release rate should be involved with the increased molecule motion of IOPs’ and swelling properties of gelatin at higher temperatures. With the increased molecular motion the interactions can be more easily distrupted and accelerated CDDP release could occur. Yet the release rate of the free CDDP on hyperthermic media was not changed for physiological pH but at pH 5 the CDDP release increased for 1.3 fold due to the reactivity of CDDP at acidic media. This is in accordance with data, the use of CDDP-MGNPs’ could lessen the undesirable side effects of CDDP in consequance of slower release rate of drug while reaching the tumor tissue.
Conclusion
In this paper, magnetic gelatin nanoparticles were synthesized with a simple method and characterized with SEM, TEM, FTIR, TG and magnetization analyses. FTIR and TG analyses showed the modification in each step from plain gelatin to gelatin nanoparticles and magnetic gelatin nanoparticles. SEM image of nanoparticles showed the spherical shape of nanoparticles and TEM images demonstrated the encapsulation of iron oxide nanoparticles into the gelatin nanoparticles. Magnetization analyse indicated that after encapsulation of iron oxide nanoparticles into the gelatin nanoparticles, obtained magnetic gelatin nanoparticles, still have a magnetic response in 0.1 T magnetic field with 20.2 emu/g response value. One of the anti-cancer agent cisplatin loaded onto magnetic gelatin nanoparticles with adsorption process. It is important to discover the adsorption mechanism which could be explained with isotherm models. Thereof, three different isotherm models carried out in the scope of this study. According to the Dubinin- Raduskevich isotherm the energy of adsorption process was 11 kJ/mol means the adsorption occurred with ion-exchange mechanism and based on the Langmiur constant, b, Gibb's free energy of the system was − 11.5 kJ/mol that indicates the process was spontaneously occurred. In vitro drug release studies showed controlled and slower release compared to the free cisplatin drug. With all the obtained data, it is expected to have a potential of cisplatin-loaded magnetic nanoparticles to be used as a chemotheraputic agent with decreased undesirable side effects and controllability with external magnetic field.
Acknowledgement
We would like to thank for financial support received from the Research Foundation of Ege University (11 FEN 074).
Declaration of interest
The authors report no declarations of interest. The authors alone are responsible for the content and wirting of the paper.
References
- Akçay G, Kılınç E, Akçay M. 2009. The equilibrium and kinetics studies of flurbiprofen adsorption onto tetrabutylammonium montmorillonite (TBAM). Colloids Surf A Physicochem Eng Asp. 335:189–193.
- Anılanmert B, Yalcın G, Arıoz F, Dolen E. 2001. The spectrophotometric determination of cisplatin in urine, using o-phenylenediamine as derivatizing agent. Anal Lett. 34:113–123.
- Babıncova M, Altanerova V, Altaner C, Bergemann C, Babınec P. 2008. In vitro analysis of cisplatin functionalized magnetic nanoparticles in combined cancer chemotherapy and electromagnetic hyperthermia. IEEE Trans Nanobioscience. 7:15–19.
- Balthasar S, Mıchaelıs K, Dınauer N, Von Brıesen H, Kreuter J, Langer K. 2005. Preparation and characterisation of antibody modified gelatin nanoparticles as drug carrier system for uptake in lymphocytes. Biomaterials. 26:2723–2732.
- Cafaggi S, Russo E, Stefanı R, Leardı R, Cavıglıolı G, Parodı B, . 2007. Preparation and evaluation of nanoparticles made of chitosan or N-trimethyl chitosan and a cisplatin-alginate complex. J Control Release. 121:110–123.
- Coester C, Nayyar P, Samuel J. 2006. In vitro uptake of gelatin nanoparticles by murine dendritic cells and their intracellular localisation. Eur J Pharm Biopharm. 62:306–314.
- Dıng D, Zhu Z, Lıu Q, Wang J, Hu Y, Jıang X, Lıu B. 2011. Cisplatin-loaded gelatin-poly(acrylic acid) nanoparticles: Synthesis, antitumor efficiency in vivo and penetration in tumors. Eur J Pharm Biopharm. 79:142–149.
- Doan TKD, Haı TH, Phuc LH, Long BD, Vınh LK, Truc PN. 2008. Preparation and characterization of magnetic nanoparticles with chitosan coating. APCTP-ASEAN Workshop on Advanced Materials Science and Nanotechnology (AMSN08), Sep 15–21 2008 Nha Trang City, VIETNAM.
- Gaıhre B, Aryal S, Khıl MS, Kım HY. 2008. Encapsulation of Fe3O4 in gelatin nanoparticles: Effect of different parameters on size and stability of the colloidal dispersion. J Microencapsul. 25:21–30.
- Gaıhre B, Khıl MS, Lee DR, Kım HY. 2009. Gelatin-coated magnetic iron oxide nanoparticles as carrier system: Drug loading and in vitro drug release study. Int J Pharm. 365:180–189.
- Ganta S, Devalapally H, Shahıwala A, Amıjı M. 2008. A review of stimuli-responsive nanocarriers for drug and gene delivery. J Control Release. 126:187–204.
- Gomez-Guıllen MC, Gımenez B, Lopez-Caballero ME, Montero MP. 2011. Functional and bioactive properties of collagen and gelatin from alternative sources: a review. Food Hydrocoll25:1813–1827.
- Jahanshahı M, Sanatı MH, Hajızadeh S, Babaeı Z. 2008. Gelatin nanoparticle fabrication and optimization of the particle size. Phys Status Solidi A. 205:2898–2902.
- Komsapenkova R, Spırova R, Bechev B. 1996. Modification of Lowry's method for collagen concentration measurement. J Biochem Biophys Methods. 32:33–43.
- Leo E, Vandellı MA, Cameronı R, Fornı F. 1997. Doxorubicin-loaded gelatin nanoparticles stabilized by glutaraldehyde: Involvement of the drug in the cross-linking process. Int J Pharm. 155:75–82.
- Lubbe AS, Alexıou C, Bergemann C. 2001. Clinical applications of magnetic drug targeting. J Surg Res. 95:200–206.
- Mıttal A, Gajbe V, Mıttal J. 2008. Removal and recovery of hazardous triphenylmethane dye, Methyl Violet through adsorption over granulated waste materials. J Hazard Mater. 150:364–375.
- Nahar M, Mıshra D, Dubey V, Jaın NK. 2008. Development, characterization, and toxicity evaluation of amphotericin B-loaded gelatin nanoparticles. Nanomed Nanotechnol Biol Med. 4:252–261.
- Ngah WSW, Fatınathan S. 2008. Adsorption of Cu(II) ions in aqueous solution using chitosan beads, chitosan-GLA beads and chitosan-alginate beads. Chem Eng J. 143:62–72.
- Ofokansı K, Wınter G, Frıcker G, Coester C. 2010. Matrix-loaded biodegradable gelatin nanoparticles as new approach to improve drug loading and delivery. Eur J Pharm Biopharm. 76:1–9.
- Perez RP. 1998. Cellular and molecular determinants of cisplatin resistance. Eur J Cancer. 34:1535–1542.
- Ramalıngam SS, Owonıkoko TK, Khurı FR. 2011. Lung cancer: new biological insights and recent therapeutic advances. CA Cancer J Clin. 61:91–112.
- Rastogı R, Gulatı N, Kotnala RK, Sharma U, Jayasundar R, Koul V. 2011. Evaluation of folate conjugated pegylated thermosensitive magnetic nanocomposites for tumor imaging and therapy. Colloids Surf B Biointerfaces. 82:160–167.
- Sıngh R, Lıllard JW. 2009. Nanoparticle-based targeted drug delivery. Exp Mol Pathol. 86:215–223.
- Taherı Qazvını N, Zınatloo S. 2011. Synthesis and characterization of gelatin nanoparticles using CDI/NHS as a non-toxic cross-linking system. J Mater Sci Mater Med. 22:63–69.
- Tseng CL, Wang TW, Dong GC, Wu SYH, Young TH, Shıeh MJ, . 2007. Development of gelatin nanoparticles with biotinylated EGF conjugation for lung cancer targeting. Biomaterials. 28:3996–4005.
- Yang XQ, Chen YH, Yuan RX, Chen GH, Blanco E, Gao JM, Shuaı XT. 2008. Folate-encoded and Fe3O4-loaded polymeric micelles for dual targeting of cancer cells. Polymer. 49:3477–3485.
- Zwıorek K, Bourquın C, Battıany J, Wınter G, Endres S, Hartmann G, Coester C. 2008. Delivery by cationic gelatin nanoparticles strongly increases the immunostimulatory effects of CpG oligonucleotides. Pharm Res. 25:551–562.