Abstract
Context
The size of nanoparticles plays a pivotal role in determining the gene delivery efficiency.
Objective
A focus on the studies done to investigate the effect of nanoparticles size on biological aspects of gene delivery.
Methods
A through literature survey has been done regarding studies done to investigate the effect of nanoparticles size on uptake, transfection efficiency and biodistribution has been cited in the present review.
Results and conclusion
The gene delivery efficacy may depend on conjugation of several factors such as the chemical structure of polymers, cell type, and nanoparticle size, composition and interaction with cells.
Introduction
Nanoparticles are defined as particles with the size in the range of few nanometers to 1000 nm. These nanoparticles can be distinguished as: (i) nanospheres which comprises of solid sphere where the drugs can either be entrapped inside the matrix or bound onto the surface and (ii) nanocapsules which consists of a solid outer covering and inner liquid core, the drug can either be encapsulated inside the core or simply attached to the outer surface (; CitationNimesh et al. 2011). Nanoparticles have found ample applications in several areas including electronics, cosmetics, food, pharmaceuticals, etc. The nanoparticles can be used to encapsulate various kinds of drugs, proteins, enzymes, DNA, and siRNA, and allow longer circulation time due to their hydrophilic nature, which masks recognition by the reticuloendothelial system (RES) cells. Owing to their small size, nanoparticles are better suited to traverse across the cell membranes and are capable of improving the pharmacokinetics, pharmacodynamics, and biodistribution of the therapeutic molecules.
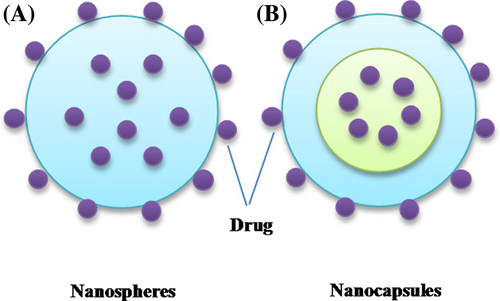
Gene delivery is one of the most important applications of nanoparticles which consist of transport of therapeutically relevant DNA to in vitro or in vivo targets. Amongst the proposed nanoparticles, polymeric nanoparticles have emerged as promising candidates, due to safety and ease of manipulation. Polymeric nanoparticles can be prepared either from pre-existing polymers or via the process of polymerization of monomeric units. Cationic polymers electrostatically interact with the anionic DNA to form condensed complexes called polyplexes, also referred as nanoparticles due to their nanometer size. The ratio between the nitrogen of amine in polymers to that of the phosphate in DNA is called N/P ratio. The N/P ratio significantly influences the size, surface charge, and stability of nanoparticles which further determines their effect on other biological aspects such as uptake and transfection efficiency. Gold nanoparticles (AuNPs) have also been utilized for gene delivery due to their low toxicity and ease of surface functionalization that in turn allows for selective tuning of surface properties such as charge and hydrophobicity. AuNPs chemically modified with primary and quaternary amine moieties were used to deliver plasmid DNA (pDNA), exhibited more efficient intracellular delivery than the conventional transfection agents (CitationSandhu et al. 2002, CitationNiidome et al. 2004). Generally, DNA is attached to AuNPs through non covalent interactions, but there are several reports where DNA modified with a thiol moiety is directly attached to the nanoparticle surface (CitationThomas and Klibanov 2003, CitationHuang et al. 2009). Additionally, AuNPs were suggested to be functionalized with various antibodies, peptides, and small molecular ligands to achieve a target cell-specific uptake, endosomal escape, and nuclear localization for efficient gene delivery. The size of nanoparticles is one of the most critical factors which determine their in vitro and in vivo fate. In the present review, we focus on the studies done to investigate the effect of nanoparticles size on various biological aspects of gene delivery, such as uptake, transfection efficiency, cytotoxicity, and biodistribution.
Effects of nanoparticles size on physicochemical and biological properties
Gene delivery to mammalian cells employing nanoparticles is a well-orchestrated multi-step process. The key steps involved are: condensation of DNA, the cellular uptake of complexes, escape from degradation vesicles, intracellular movement or ‘trafficking’, nuclear translocation and finally unpacking followed by translation (). The major rate-limiting step depends upon the nature of the polymer or size of the nanoparticles employed.
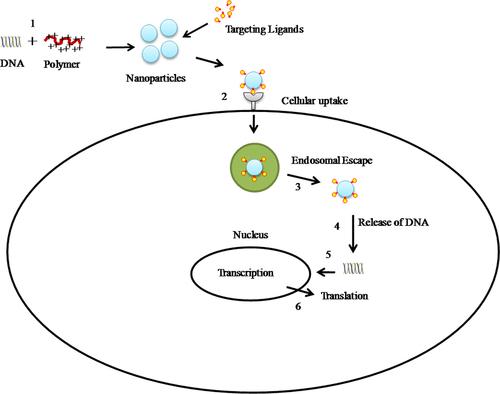
Cellular internalization of nanoparticles
Uptake of therapeutic molecules with molecular weight (MW) above 1 kDa is selectively inhibited by the plasma membrane of the cells and cellular internalization is strictly limited to the endocytic route. The interaction between the extracellular macromolecules and cell surface is controlled by anionic proteoglycans that plays a significant role in cellular uptake of cationic gene delivery vectors (CitationYanagishita and Hascall 1992, CitationMislick and Baldeschwieler 1996, CitationKichler et al. 2006). Cationic nanoparticles interact with the anionic cell membranes via electrostatic forces of attraction, thereby leading to entry of nanoparticles by process called endocytosis. Endocytosis of nanoparticles is a tightly regulated process that involves three major steps. The process initiates with engulfment of nanoparticles in membrane invaginations that squeeze off to form membrane-bound vesicles, termed as endosomes (or phagosomes during phagocytosis of molecules). The endosomes that originates due to engulfment, at different sites of the cell membrane are heterogeneous in nature and possess distinct endocytic components. The next step consists of payload delivery by endosomes to different specialized vesicles, which facilitates sorting of payload toward different destinations. The final step involves delivery of payload to various intracellular compartments, recycled to the extracellular milieu or delivered across cells; this process is termed as transcytosis. The mechanism of endocytosis can be broadly categorized into—phagocytosis (uptake of solid or large particles) and pinocytosis (uptake of liquids and various solutes; ). On the basis of proteins involved in different endocytic pathways, pinocytosis is divided into clathrin-dependent endocytosis (or clathrin-mediated endocytosis) and clathrin-independent endocytosis. The clathrin-independent mechanisms are further divided into (1) caveolae-mediated endocytosis, (2) clathrin- and caveolae-independent endocytosis, and (3) macropinocytosis (CitationConner and Schmid 2003). Clathrin- and caveolae-independent mechanism are subdivided into Arf6-dependent, Cdc42-dependent, flotillin-dependent, and RhoA-dependent endocytosis (CitationMayor and Pagano 2007).
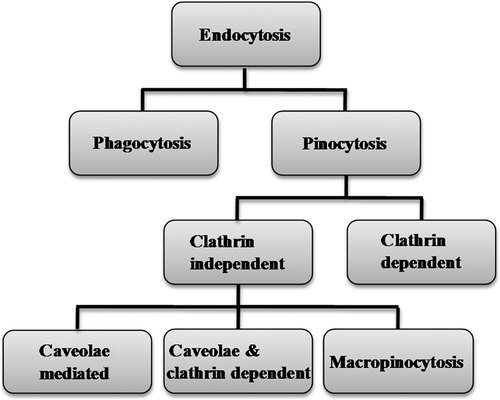
Study of the effect of particle size on the internalization pathway and subsequent intracellular fate in non- phagocytic B16 cells revealed that latex particles of size up to 500 nm were internalized by cells via an energy-dependent process (CitationRejman et al. 2004). Uptake efficiency was suppressed by depletion of cholesterol, with the increase in size of nanoparticles from 50 to 500 nm. Further, disruption of microtubules significantly perturbed the processing of the smaller particles, whereas the uptake of 500 nm size nanoparticles remained unaffected. Investigation of pathways of internalization suggested strong dependence on the particle size (; CitationRejman et al. 2004). Nanoparticles of size less than 200 nm were internalized through clathrin-dependent endocytosis. However, when the size of nanoparticles was increased above 200 nm, caveolae-mediated internalization mechanism became evident, and remained the predominant entry pathway for nanoparticles of size 500 nm. At these conditions, the sequestration of nanoparticles through lysosomes was no longer observed (CitationRejman et al. 2004).
Table I. Effect of nanoparticles size on internalization.
A preferential particle uptake mediated through a clathrin- and caveolae-independent mechanism was observed with the reduction in size of carboxyl-modified fluorescent polystyrene nanoparticles (from 43 to 24 nm) (CitationLai et al. 2007). Internalization of nanoparticles in the membrane bound vesicles was an energy-dependent process. This pathway leads to particle trafficking via non-degradative/non-acidic vesicles, thereby resulting in rapid localization of nanoparticles on the periphery of the nucleus (CitationLai et al. 2007). Further, particle tracking suggested that non-acidic vesicles undergo long-range displacements along microtubules at a much lower frequency compared to that of endo/lysosomal vesicles originating by clathrin-mediated endocytosis (CitationLai et al. 2008). A distinct fusion and budding patterns were also observed with non-acidic vesicles. A prolonged retention time near the nucleus that was due to the slower transport and non-acidic nature of non-acidic vesicles following non-clathrin– and non-caveolae–mediated endocytosis, may further enhance delivery to the nucleus (CitationLai et al. 2008).
Size also governs the efficacy of uptake of nanoparticles by the cells. In a study being conducted in Caco-2 cell lines, it was shown that 100 nm-sized nanoparticles of poly (D,L-lactide-co-glycolide) (PLGA) were better taken up, about 2.5-fold higher uptake in comparison to 1μm-sized and 6-fold more uptake if compared to 10 μm microparticles (CitationGratton et al. 2008). Not only this, in rat in situ intestinal loop model 15–250 fold higher uptake efficiency is being reported with 100 nm nanoparticles as compared to the large-sized microparticles (CitationGregoriadis 1988). Cell type also decides the maximum size for nanoparticles that can be taken in. For example, hepatic cell lines, namely, Hep G2 and Hepa 1–6 can internalized nanoparticles of sizes 93 and 220 nm whereas other cell lines such as HUVEC, HNX 14C, and ECV 30 efficiently internalize particles much larger in size, upto 1μm, even though grown to full confluency (CitationHe et al. 2010). In another study done in human arterial smooth muscle cells (HAVSMCs) with various nanoparticle formulations, it was shown that 380 nm-sized nanoparticles were better internalized than 520 nm ones. Increased hydrophlicity of 380 nm nanoparticles were attributed toward higher uptake efficiency. 300 nm nanoparticles contained 5% polyvinyl alcohol (PVA) while 520 nm nanoparticles had only 0.5% PVA (CitationHuang et al. 2009).
Size of the particles is one of the most significant factors in the process of uptake of nanoparticles by mucosal and epithelial tissue, followed by intracellular trafficking (CitationPanyam and Labhasetwar 2003). Small-size nanoparticles (∼100 nm) have been observed to have more than 3-fold greater arterial uptake compared to large nanoparticles (∼275 nm) in an ex vivo canine carotid artery model (CitationShu and Zhu 2000, CitationSong et al. 1998). The smaller nanoparticles easily penetrated through the submucosal layers while the larger size micron-particles were mostly localized in the epithelial lining. The polystyrene particles demonstrated interaction with the dendritic cells throughout the tested diameter range of 40 nm–15 μm in a time- and concentration-dependent manner (CitationFoged et al. 2005). After 24h of incubation, only a small number of dendritic cells shown to be associated with 4.5 μm particles while approximately 30% of the cells interacted with 500 nm particles and more than 60% interacted with 100 nm particles. The study suggested that optimal particle diameter for fast and efficient acquisition by a substantial percentage of the dendritic cells was 500 nm and below (CitationFoged et al. 2005).
Although, the small size of nanoparticles may be beneficial for rapid entry into cells, there is no size cut-off limit up to at least 5 μm to gain cellular entry through pinocytosis (CitationGratton et al. 2008). The Particle Replication In Non-wetting Templates (PRINT) technique was used to fabricate micro- and nanoparticles from cationic, cross-linked polyethylene glycol (PEG) hydrogels and employed to investigate the interdependent effect of size, shape, and surface charge (zeta potential) on their internalization by human cervical carcinoma epithelial (HeLa) cells. Uptake studies with nanoparticles revealed that HeLa cells internalized all three different types of cylindrical nanoparticles with diameters of 200, 100, and 150 nm with aspect ratios (i.e., length-to-diameter ratio) of 1, 3, and 3, respectively (CitationGratton et al. 2008). The internalization of high-aspect-ratio nanoparticles (d = 150 nm, h = 450 nm) by HeLa cells was approximately four times faster than the more symmetric low-aspect-ratio particles (d = 200 nm, h = 200 nm). Further, the internalization of nanoparticles of diameter 100 nm, an aspect ratio of 3, was significantly lower than the larger nanoparticles of a diameter of 150 nm with the same aspect ratio. This high uptake was attributed to multivalent cationic interactions with cells due to the higher-aspect-ratio particles as they contribute larger surface areas for contact with the cell membrane. It was deduced that the uptake kinetics of nanoparticles by HeLa was remarkably influenced by the size as well as the volume of the particles (CitationGratton et al. 2008).
Investigation of herceptin functionalized AuNPs ranging from 14 to 100 nm, suggested that the maximal uptake by ErbB2 over-expressing human breast cancer SK-BR-3 cells occurred in between 25 and 50 nm range (CitationJiang et al. 2008). Uptake kinetics data showed that 40- to 50-nm nanoparticles form the critical cut-off size for receptor-mediated internalization. This optimal size for particle uptake was attributed to the direct balance between multivalent cross-linking of membrane receptors and the process of membrane wrapping involved in receptor-mediated endocytosis (CitationJiang et al. 2008). The uptake studies of 14-, 50-, and 74-nm AuNPs in Hela cells showed that the kinetics of uptake as well as the saturation concentration differed with the various sized nanoparticles (CitationChithrani and Chan 2007, CitationChithrani et al. 2006). The nanoparticles with size of 50-nm resulted in maximal uptake suggesting that there exists optimal size for efficient nanomaterial uptake into cells.
Transfection efficiency
Cationic polymers condense anionic DNA molecules into well-defined particles in nanometer range which facilitate cellular uptake through endocytosis, the subsequent DNA release, trafficking, and fate inside the cell. Nanoparticles protect DNA from both endocytotic processing and cytoplasmic nucleases activity followed by dissociation of the condensed complexes either before or after entering the nucleus. Access to nucleus has been suggested to occur through nuclear pores (which are ∼10 nm in diameter) or during cell division (CitationPante and Aebi 1996). The transfection efficiency of DNA delivered to nucleus further depends on the composition of the gene expression system. The transfection efficiency may depend on several factors such as the chemical structure of polycations, cell type, and nanoparticle size, composition and interaction with cells.
Transfection efficiency is dependent on several parameters and one much important criteria is the size of particles used to form polymer/DNA complexes. Several studies that are contradicting one another have been done to study the effect of size on efficiency of nanoparticles-mediated transfection. When 30–60 nm polyethylenimine (PEI)/DNA nanoparticles were compared with aggregated nanoparticles, with average size 300–600 nm, the later showed higher transfection, about 100–500 fold better luciferase gene expression in Neuro2α neuroblastoma cells and erythromyeloid K562 cells () (CitationMayer et al. 2005).
Table II. Effect of nanoparticles size on transfection efficiency.
Also, transfection studies in B16F10 melanoma cells showed that large-size nanoparticles showed 10-fold higher efficacy with PEI/DNA nanoparticles prepared at 5 μg of DNA, while it was 100-fold with 2.5 μg of DNA (CitationOgris et al. 1998). Larger size (> 500 nm) transferrin-PEI800 (PEI MW 800 Da)/DNA complexes showed higher luciferase gene-transfer level compared to that of the smaller size complexes prepared under low ionic conditions (40–100 nm; CitationOgris et al. 2001).With an increase in the amount of poly(γ-glutamic acid) (γ-PGA) incorporation in chitosan/DNA complexes, the size of nanoparticles increased significantly from approximately 140 to 204 nm, with increase in ratio of γ-PGA to that of chitosan from 0 to 6 and transfection efficacy improved by up to 4-fold (CitationPeng et al. 2009). The higher transfection efficacy exhibited by the large particles results from the conjunction of several features (i) they sediment onto the cell surface more rapidly than the small particles, thereby increasing the contact with the cells which further induces cellular internalization (ii) since they contain a large proportion of free cationic polymers in addition to those complexed with DNA, they destabilize the membrane favoring their entry into cells, and (iii) their endosomolytic activity is far higher than that of the small particles (CitationOgris et al. 1998).
On the other hand, higher transfection efficiency was exhibited by transferrin-polylysine/DNA complexes of size 80–100 nm, as compared to larger DNA complexes (CitationWagner et al. 1991). The influence of particle sizes on DNA delivery and subsequent gene expression was more pronounced when the particles are allowed to grow for 30 min; wherein Mg2+ inclusion resulted in remarkable decrease in the particles size from 2.5 μm to 500 nm and enhanced gene expression by 40-fold (CitationChowdhury et al. 2004). Further, complexes of linear poly(amidoamine)/DNA of size 200 nm at N/P ratio of 5 showed better transfection efficacy than the complexes of 600 nm size at same N/P ratio (CitationJones et al. 2000). Optimization studies revealed of best transfection efficiency of the poly ((2-dimethylamino) ethyl methacrylate) (PDMAEMA)/pDNA particles at PDMAEMA/plasmid ratio of 3 (w/w) possessing a slightly positively charged particles with a narrow size distribution and average diameter of 150 nm. However, PDMAEMA/pDNA ratios less than 1.5 (w/w) resulted in large particles with high polydispersity index that showed lower transfection efficacy (CitationCherng et al. 1996). The low transfection efficacy of large-sized particles was attributed with the volume being too large to be taken up by the cells.
Recently, the influence of particle size on cell transfection was investigated using AuNPs combined with PEI (CitationCebrián et al. 2011). Two different sizes of Au-PEI nanoparticles of approximately 6 and 70 nm were complexed with pDNA carrying reporter or suicide genes to prepare Au-PEI/DNA complexes, and transfection efficacy investigated in human osteosarcoma Saos-2 cells. Results revealed efficient transfection with complexes derived from approximately 6 nm Au-PEI nanoparticles as compared to approximately 70 nm Au-PEI nanoparticles. Further investigation showed presence of large aggregates of approximately 70 nm nanoparticles in endocytic vesicles of cells while smaller nanoparticles showed lower agglomeration and effective endosomal escape (CitationCebrián et al. 2011). Co-localization studies have given evidences of presence of DNA inside the cell nucleus after 2h of transfection with approximately 6 nm Au-PEI nanoparticles that remained present in the sub-cellular compartment up to 3 days (CitationCebrián et al. 2011).
Transfection efficiency studies with fractioned PLGA nanoparticles showed that the small-sized nanoparticles (∼70 nm) showed a 27-fold higher transfection than the large-sized nanoparticles (∼200 nm) or the unfractionated nanoparticles in COS-7 cell line, while a 4-fold higher transfection was observed in HEK-293 cell line (CitationPrabha et al. 2002). The higher transfection efficacy of small-sized nanoparticles was hypothesized to be due to the difference in the total number of particles present in the cells for the same mass of nanoparticles being taken up by the cells. Further, on the basis of the mean particle diameter of the two fractions of nanoparticles and the cellular uptake data, the number of the small-sized nanoparticles taken up by the cells was estimated to be approximately 20-fold greater than the large-sized nanoparticles (350 × 108 vs. 17 × 108per mg cell protein for small- and large-sized nanoparticles, respectively; CitationMuller 1991).
Mixing of oligonucleotides (ODNs) with protamine free base solution spontaneously formed nanoparticles with a diameter of 150–170 nm (CitationJunghans et al. 2000). However, complexation of human serum albumin (HSA) to protamine followed by condensation of ODNs leads to formation of nanoparticles (HSA-Prot/DNA) of size 230–320 nm (CitationLochmann et al. 2005). These large-sized nanoparticles showed antisense effect of about 35% in a functional assay as well as on the protein level (western blot; CitationWeyermann et al. 2005). However, substitution of the protamine free base by protamine sulfate in HSA-Prot/DNA nanoparticles lead to drastic reduction in size, with nanoparticles of only around 40 nm in diameter with efficient transfection efficacy (CitationMayer et al. 2005).
The DNA/transferrin-PEI and DNA/transferrin-PEI/PEG complexes generated under conditions of low salt (HEPES-buffered glucose, HBG) appeared as spherical particles with a diameter of 40 nm (CitationOgris et al. 1999). Non-PEGylated complexes formed aggregates with plasma proteins; in contrast, PEG-modified complexes remain small and un-aggregated and showed efficient transfection efficiency in human cell lines K562 and Neuro2α (CitationOgris et al. 1999). Modification of PEI by linking cholesteryl chloroformate (PEI-Chol) to the secondary amino groups of BPEI (MW: 1.8 and 10 kDa) and complexation of pDNA yielded complexes in the range 110–205 nm, while PEI of 1.8 kDa, gave particle size of 384 ± 300 nm (CitationWang et al. 2002). The in vitro transfection with small-size complexes in Jurkat cells showed high levels of expressed green fluorescent protein (GFP) with much less toxicity in contrast to larger complexes (CitationWang et al. 2002). Acetylation of PEI (25 kDa) increased the polyplex diameter by two- to three-fold and the gene delivery efficacy increased by up to 21-fold compared to unmodified PEI, both in the presence and absence of serum (CitationForrest et al. 2004).
Cytotoxicity
Because nanoparticles employed for gene delivery are engineered to interact with the cells, facilitate internalization and release DNA to mediate gene expression, it is imperative to evaluate that these enhancements are not causing adverse effects. Moreover, all the nanoparticles whether prepared from natural or synthetic polymer, plain or modified undergo degradation inside the cells and may induce certain biological responses such as disruption of organelle integrity or alter gene expression. The in vitro studies with nanoparticles involve less stringent toxicological characterization, while in vivo application would require thorough understanding of the kinetics and mechanism of nanoparticles-induced toxicology. A plethora of reports has been published reporting in vitro cytotoxicity studies of nanoparticles using different cell lines, varying incubation times, and colorimetric assays. A wide range of nanoparticle concentrations and exposure times, have been employed in cytotoxicity assays which renders difficulty in establishing the physiological relevance. Additionally, several different cell lines as well as culture conditions have been investigated which further add to the complexity of generated data and making comparisons between the available studies difficult.
Nanoparticles size has been found to considerably influence its cytotoxicity. Exposure of different size PLGA nanoparticles (60, 100, and 200 nm) to murine macrophage cells (RAW264.7) and human bronchial epithelial cells (BEAS-2B) for 24h showed size-dependent cytotoxicity and smaller particles tend to trigger more damage (; CitationXiong et al. 2013). The smaller particles (60 and 100 nm) at concentration of 10 to 100 μg/ml, triggered more release of cytokine TNF-α in RAW264.7 cells when compared with control. However the 200 nm-sized nanoparticles did not trigger significant release of TNF-α up to concentration of 300 μg/ml (CitationXiong et al. 2013); it is because, smaller nanoparticles have larger surface area and higher percentage of molecules exposed on particle surface to interact with surrounding biomolecules. The protein adsorption assay as a measure of toxicity correlated well with the surface area of the PLGA nanoparticles (CitationXiong et al. 2013). CitationDeng et al. (2011) observed that the interaction between poly (acrylic acid)-coated AuNPs and fibrinogens would induce the unfolding of protein fibrinogen, which resulted in the activation of Mac-1 reception and inflammation response. The binding of AuNPs with fibrinogens was influenced by the size with smaller nanoparticles (5 nm) showed more fibrinogen binding than with bigger particles (20 nm), and the adsorption was proportional to the surface area of nanoparticles (CitationDeng et al. 2011).
Table III. Effect of nanoparticles size on cytotoxicity.
A systematic study of water soluble AuNPs in size range from 0.8 to 15 nm prepared by stabilization with triphenylphosphine showed size-dependent toxicity in a variety of cell types with barrier and phagocyte function (CitationPan et al. 2007). AuNPs of 1.4 nm showed IC50 values (inhibitory concentrations that effected 50% growth inhibition) ranging from 30 to 56 μM depending on cell lines tested, such as connective tissue fibroblasts, epithelial cells, macrophages, and melanoma cells. On the contrary, AuNPs of 15 nm in size and Tauredon (gold thiomalate) were non-toxic up to 60-fold and 100-fold higher concentrations, respectively. Moreover, 1.4-nm AuNPs mainly caused cell death by necrosis within12h while almost similar size 1.2 nm AuNPs lead to programmed cell death by apoptosis (CitationPan et al. 2007).
Pharmacokinetics and biodistribution
Particle size is one of the most critical factors determining the in vivo fate of nanoparticles, such as retention in the blood, bypass various biological barriers, and diffusion through tissues. Particle size also dictates the uptake and removal by reticuloendothelial system (RES) cells, specifically in the liver and spleen. Also, nanoparticles size will facilitate the internalization by the target cells. As evident from the literature, for most in vivo applications, nanoparticles less than 100 nm appears to be a prerequisite for efficient access to vascular fenestrations and effective diffusion through tissues. Moreover, the characteristics of target cells may influence the optimal size for internalization and further decide the preferred uptake mechanisms that can be endocytosis, pinocytosis, or phagocytosis. However, small or large particles may gain access to target cells through different intracellular pathways, which may result in completely distinct fates that could be transfection or degradation. The complexes should also remain soluble and stable, escape aggregation in the blood, or prevent exposure of DNA to degrading enzymes within the blood or inter-tissue fluid. Furthermore, complexes should be fabricated so as to minimize interaction with blood components, such as plasma, complement components, RES cells (e.g., macrophages), extracellular matrix and other nontarget cells (CitationGregoriadis 1988).
The effectiveness of the PEG steric barrier is influenced by the composition of the PEGylated nanoparticles and the size that further dictates the biodistribution of the nanoparticles (; CitationStolnik et al. 2001, CitationMosqueira et al. 2001, CitationAvgoustakis et al. 2003). An increase in the PEG/PLA or PEG/PLGA ratio was observed to initially increase but later decrease the blood circulation time of PLA-PEG or PLGA-PEG diblock nanoparticles prepared by the solvent displacement method (CitationAvgoustakis et al. 2003, CitationStolnik et al. 2001). This pronounced effect was attributed to the size of the nanoparticles that was sufficiently low to permit the nanoparticles to reach tissues at relatively high PEG/PLA or PEG/PLGA ratios, with PEG still forms an effective steric barrier to opsonization on the surface of the nanoparticles, while the larger nanoparticles cannot reach (those having relatively low PEG/PLA or PEG/PLGA ratios) the target tissue. This explanation was later justified by the observation that the very small particles can pass through the sinusoidal fenestrations in the liver and gain access to the parenchymal cells of the liver (CitationStolnik et al. 2001). Increasing the PLGA-PEG content of the PLGA/PLGA-PEG mixture, or when PLGA(45)-PEG(5) was replaced by PLGA(5)–PEG(5), a decrease in the size of the nanoparticles was observed from approximately 145 nm to approximately 60–85 nm (CitationBeletsi et al. 2005). The blood residence of the PLGA/PLGA(45)-PEG(5) nanoparticles increased as their PLGA-PEG content was increased, reaching maximum blood longevity at 100% PLGA(45)-PEG(5).
Table IV. Effect of nanoparticles size on pharmacokinetics and biodistribution.
Three sizes (about 80, 170, and 240 nm) of poly methoxy polyethyleneglycol cyanoacrylate-co-n-hexadecyl cyanoacrylate (PEG-PHDCA) nanoparticles loaded with recombinant human tumor necrosis factor-α (rHuTNF-α), incubated with serum protein for 2h, showed a significant correlation between particle size and protein absorption (CitationFang et al. 2006). Protein absorption on 80,171, and 243 nm size nanoparticles was found to be 6, 23, and 34%, respectively, while on non-PEGylated PHDCA of all size it was approximately 55%. The effect of protein absorption on different sized nanoparticles was also confirmed with the analysis of nanoparticle uptake by murine macrophages and blood clearance kinetics. The uptake of PEG-PHDCA nanoparticles was size dependent at after 1 and 2h of incubation with highest uptake for 240 nm followed by 170 and 80 nm. Blood clearance of the smaller nanoparticles was approximately 2-fold slower than the larger nanoparticles (CitationFang et al. 2006). Moreover, the influence of particle size on the elimination half-lives of rHuTNF-α loaded in PEG-PHDCA nanoparticles was consistent with that on the serum protein absorption and macrophage uptake with 80 nm nanoparticles having the longest circulation time. The results suggest that it might be due to a higher surface PEG density (brush-like) on the surface of smaller nanoparticles (CitationFang et al. 2006).
PEG-modified poly-ϵ-caprolactone nanoparticles were developed for systemic delivery of tamoxifen in breast cancer (CitationShenoy and Amiji 2005). This study observed that PEG modification not only reduced particle size and aggregation, but significantly enhanced the circulation time, allowing approximately 18% of the injected dose to accumulate in the tumor mass within 1h, in an in vivo model of human breast adenocarcinoma, in contrast to the 5% tumor accumulation of the nanoparticles lacking PEG (CitationShenoy and Amiji 2005). To elucidate the effects of particle size on cellular uptake and biodistribution of polymeric nanoparticles, rhodamine B (RhB) labeled carboxymethyl chitosan-grafted nanoparticles (RhB-CMCNP) and chitosan hydrochloride-grafted nanoparticles (RhB-CHNP) were developed with well-defined particle sizes of 150–500 nm (CitationHe et al. 2010). Slight particle size differences had significant implications in the cellular uptake of nanoparticles. In vivo biodistribution data suggested that nanoparticles with slight negative charges and particle size of 150 nm were tended to accumulate in tumor cells more efficiently (CitationHe et al. 2010).
In vivo studies with block copolymer micelles (BCMs) in athymic mice bearing human breast cancer xenografts that express differential levels of EGF receptors revealed size-dependent distribution (CitationLee et al. 2010). BCMs of 25 nm size were rapidly cleared from the plasma compared to 60 nm size leading to approximately 2-fold decrease in total tumor accumulation of the latter. Further, non targeted BCMs of 25 nm diffused from the blood vessels following extravasation, while nontargeted BCMs of 60 nm remained in the perivascular regions. Targeting of BCMs with EGF resulted in remarkable nuclear uptake of 25 nm BCMs in vitro and in vivo via active transport (CitationLee et al. 2010).
Intravenous administration of AuNPs with a diameter of 10, 50, 100, and 250 nm in rat via tail vein resulted in size- dependent distribution (CitationDe Jong et al. 2008). AuNPs of size 10 nm were found to localize in various organs including blood, liver, spleen, kidney, testis, thymus, heart, lung, and brain, while the larger particles were only detected in blood, liver, and spleen (CitationDe Jong et al. 2008). Biodistribution studies with AuNPs of 20, 40, and 80 nm coated with thioctic acid terminated PEG (MW: 5 kDa) in nude mice bearing subcutaneous A431 squamous tumors showed that the 20-nm AuNPs exhibited the lowest uptake by RES cells and the slowest clearance from the body (CitationZhang et al. 2009). Moreover, the 20-nm AuNPs yielded remarkably high tumor uptake and extravasation from the tumor blood vessels as compared to 40- and 80-nm AuNPs.
Conclusions
On the basis of available data, it can be summarized that the size of nanoparticles significantly influences the pathway of cellular entry, transfection efficiency, cytotoxicity, and in vivo biodistribution. The clathrin-mediated endocytosis pathway shows an upper size limit of approximately 200 nm for internalization, and above this size caveolae-mediated endocytosis predominates. Polymeric nanoparticles with size of 100 nm or less have been effectively internalized in a variety of cell lines and 50 nm appears to be optimal size for uptake of AuNPs. However, there are conflicting reports on the effect of particle size on nanoparticle-mediated transfection. Some studies reported higher transfection efficiency with nanoparticles of smaller size (< 200 nm) while others showed efficacy of larger size (> 200 nm) nanoparticles. Further, association of high cytotoxicity with the small-sized nanoparticles could be due to the fact that the smaller nanoparticles have larger surface area and higher percentage of molecules exposed on particle surface to interact with cells. Furthermore, the small-sized nanoparticles, specially, those with surface modified with biocompatible polymers, such PEG, have been shown to have prolonged blood circulation time and those attached with targeting moieties have better penetration. Overall, it can be deduced that nanoparticles with sizes less than 100 nm, coated with biocompatible polymers and conjugated to targeting ligands, will emerge as successful gene delivery vectors with clinical relevance.
Acknowledgments
The authors gratefully acknowledge the help rendered by Rajesh Ranjan (IRIC, University of Montreal, Montreal) for helping with literature access.
Declaration of interest
The authors report no declaration of interest. The authors alone are responsible for the content and writing of the paper.
This work was supported by Science & Engineering Research Board (SERB), Department of Science and Technology (SERC Fast Track Proposals for Young Scientists Scheme), and Government of India.
References
- Avgoustakis K, Beletsi A, Panagi Z, Klepetsanis P, Livaniou E, Evangelatos G, Ithakissios DS. 2003. Effect of copolymer composition on the physicochemical characteristics, in vitro stability, and biodistribution of PLGA-mPEG nanoparticles. Int J Pharm. 259:115–127.
- Beletsi A, Panagi Z, Avgoustakis K. 2005. Biodistribution properties of nanoparticles based on mixtures of PLGA with PLGA-PEG diblock copolymers. Int J Pharm. 298:233–241.
- Cebrián V, Martín-Saavedra F, Yagüe C, Arruebo M, Santamaría J, Vilaboa N. 2011. Size-dependent transfection efficiency of PEI-coated gold nanoparticles. Acta Biomater. 7:3645–3655.
- Cherng JY, van de Wetering P, Talsma H, Crommelin DJ, Hennink WE. 1996. Effect of size and serum proteins on transfection efficiency of poly ((2-dimethylamino)ethyl methacrylate)-plasmid nanoparticles. Pharm Res. 13:1038–42.
- Chithrani BD, Chan WC. 2007. Elucidating the mechanism of cellular uptake and removal of protein-coated gold nanoparticles of different sizes and shapes. Nano Lett. 7:1542–1550.
- Chithrani BD, Ghazani AA, Chan WC. 2006. Determining the size and shape dependence of gold nanoparticle uptake into mammalian cells. Nano Lett. 6:662–8.
- Chowdhury EH, Kunou M, Nagaoka M, Kundu AK, Hoshiba T, Akaike T. 2004. High-efficiency gene delivery for expression in mammalian cells by nanoprecipitates of Ca–Mg phosphate. Gene. 341:77–82.
- Conner SD, Schmid SL. 2003. Regulated portals of entry into the cell. Nature. 422:37–44.
- De Jong WH, Hagens WI, Krystek P, Burger MC, Sips AJ, Geertsma RE. 2008. Particle size-dependent organ distribution of gold nanoparticles after intravenous administration. Biomaterials. 29:1912–1919.
- Deng ZJ, Liang M, Monteiro M, Toth I, Minchin RF. 2011. Nanoparticle-induced unfolding of fibrinogen promotes Mac-1 receptor activation and inflammation. Nat Nanotechnol. 6:39–44.
- Fang C, Shi B, Pei YY, Hong MH, Wu J, Chen HZ. 2006. In vivo tumor targeting of tumor necrosis factor-alpha-loaded stealth nanoparticles: effect of MePEG molecular weight and particle size. Eur J Pharm Sci. 27:27–36.
- Foged C, Brodin B, Frokjaer S, Sundblad A. 2005. Particle size and surface charge affect particle uptake by human dendritic cells in an in vitro model. Int J Pharm. 298:315–322.
- Forrest ML, Meister GE, Koerber JT, Pack DW. 2004. Partial acetylation of polyethylenimine enhances in vitro gene delivery. Pharm Res. 21:365–371.
- Gratton SE, Ropp PA, Pohlhaus PD, Luft JC, Madden VJ, Napier ME, Desimone JM. 2008. The effect of particle design on cellular internalization pathways. Proc Natl Acad Sci U S A. 105:11613–11618.
- Gregoriadis G (Ed.) 1988. Liposomes as Drug Carriers: Recent Trends and Progress New York: Wiley.
- He C, Hu Y, Yin L, Tang C, Yin C. 2010. Effects of particle size and surface charge on cellular uptake and biodistribution of polymeric nanoparticles. Biomaterials. 31:3657–3666.
- Huang HC, Barua S, Kay DB, Rege K. 2009. Simultaneous enhancement of photothermal stability and gene delivery efficacy of gold nanorods using polyelectrolytes. ACS Nano. 3:2941–2952.
- Jiang W, Kimbetty YS, Rutka JT, Chanwarren CW. 2008. Nanoparticle-mediated cellular response is size-dependent. Nat Nano. 3:145–150.
- Jones NA, Hill IRC, Stolnik S, Bignotti F, Davis SS, Garnett MC. 2000. Polymer chemical structure is a key determinant of physicochemical and colloidal properties of polymer–DNA complexes for gene delivery. Biochim Biophys Acta. 1517:1–18.
- Junghans M, Kreuter J, Zimmer A. 2000. Antisense delivery using protamine-oligonucleotide particles. Nucleic Acids Res. 28:E45.
- Kichler A, Mason AJ, Bechinger B. 2006. Cationic amphipathic histidine-rich peptides for gene delivery. Biochim Biophys Acta. 1758:301–307.
- Lai SK, Hida K, Chen C, Hanes J. 2008. Characterization of the intracellular dynamics of a non-degradative pathway accessed by polymer nanoparticles. J Control Release. 125:107–111.
- Lai SK, Hida K, Man ST, Chen C, Machamer C, Schroer TA, Hanes J. 2007. Privileged delivery of polymer nanoparticles to the perinuclear region of live cells via a non-clathrin, non-degradative pathway. Biomaterials. 28:2876–2884.
- Lee H, Fonge H, Hoang B, Reilly RM, Allen C. 2010. The effects of particle size and molecular targeting on the intratumoral and subcellular distribution of polymeric nanoparticles. Mol Pharm. 7:1195–1208.
- Lochmann D, Weyermann J, Georgens C, Prassl R, Zimmer A. 2005. Albumin-protamine-oligonucleotide nanoparticles as a new antisense delivery system. Part. 1:physicochemical characterization. Eur J Pharm Biopharm. 59:419–429.
- Mayer G, Vogel V, Weyermann J, Lochmann D, van den Broek JA, Tziatzios C, et al. 2005. Oligonucleotide-protamine-albumin nanoparticles: protamine sulfate causes drastic size reduction. J Control Release. 106:181–187.
- Mayor S, Pagano RE. 2007. Pathways of clathrin-independent endocytosis. Nat Rev Mol Cell Biol. 8:603–612.
- Mislick KA, Baldeschwieler JD. 1996. Evidence for the role of proteoglycans in cation-mediated gene transfer. Proc Natl Acad Sci U S A. 93:12349–12354.
- Mosqueira VC, Legrand P, Morgat JL, Vert M, Mysiakine E, Gref R, et al. 2001. Biodistribution of long-circulating PEG-grafted nanocapsules in mice: effects of PEG chain length and density. Pharm Res. 18:1411–1419.
- Muller RH (Ed.) 1991. Colloidal Carriers for Controlled Drug Delivery and Targeting. Boca Raton: CRC press.
- Niidome T, Nakashima K, Takahashi H, Niidome Y. 2004. Preparation of primary amine-modified gold nanoparticles and their transfection ability into cultivated cells. Chem Commun. (17): 1978–1979.
- Nimesh S, Gupta N, Chandra R. 2011. Cationic polymer based nanocarriers for delivery of therapeutic nucleic acids. J Biomed Nanotechnol. 7:504–520.
- Ogris M, Brunner S, Schuller S, Kircheis R, Wagner E. 1999. PEGylated DNA/transferrin-PEI complexes: reduced interaction with blood components, extended circulation in blood and potential for systemic gene delivery. Gene Ther. 6:595–605.
- Ogris M, Steinlein P, Carotta S, Brunner S, Wagner E. 2001. DNA/polyethylenimine transfection particles: influence of ligands, polymer size, and PEGylation on internalization and gene expression. AAPS PharmSci. 3:E21.
- Ogris M, Steinlein P, Kursa M, Mechtler K, Kircheis R, Wagner E. 1998. The size of DNA/transferrin-PEI complexes is an important factor for gene expression in cultured cells. Gene Ther. 5: 1425–1433.
- Pan Y, Neuss S, Leifert A, Fischler M, Wen F, Simon U, et al. 2007. Size-dependent cytotoxicity of gold nanoparticles. Small. 3: 1941–1949.
- Pante N, Aebi U. 1996. Molecular dissection of the nuclear pore complex. Crit Rev Biochem Mol Biol. 31:153–199.
- Panyam J, Labhasetwar V. 2003. Biodegradable nanoparticles for drug and gene delivery to cells and tissue. Adv Drug Deliv Rev. 55: 329–347.
- Peng SF, Yang MJ, Su CJ, Chen HL, Lee PW, Wei MC, Sung HW. 2009. Effects of incorporation of poly(gamma-glutamic acid) in chitosan/DNA complex nanoparticles on cellular uptake and transfection efficiency. Biomaterials. 30:1797–1808.
- Prabha S, Zhou WZ, Panyam J, Labhasetwar V. 2002. Size-dependency of nanoparticle-mediated gene transfection: studies with fractionated nanoparticles. Int J Pharm. 244:105–115.
- Rejman J, Oberle V, Zuhorn IS, Hoekstra D. 2004. Size-dependent internalization of particles via the pathways of clathrin- and caveolae-mediated endocytosis. Biochem J. 377:159–169.
- Sandhu KK, Mcintosh CM, Simard JM, Smith SW, Rotello VM. 2002. Gold nanoparticle-mediated transfection of mammalian cells. Bioconjug Chem. 13:3–6.
- Shenoy DB, Amiji MM. 2005. Poly(ethylene oxide)-modified poly(epsilon-caprolactone) nanoparticles for targeted delivery of tamoxifen in breast cancer. Int J Pharm. 293:261–270.
- Shu XZ, Zhu KJ. 2000. A novel approach to prepare tripolyphosphate/chitosan complex beads for controlled release drug delivery. Int J Pharm. 201:51–58.
- Song C, Labhasetwar V, Cui X, Underwood T, Levy RJ. 1998. Arterial uptake of biodegradable nanoparticles for intravascular local drug delivery: results with an acute dog model. J Control Release. 54: 201–211.
- Stolnik S, Heald CR, Neal J, Garnett MC, Davis SS, Illum L, et al. 2001. Polylactide-poly(ethylene glycol) micellar-like particles as potential drug carriers: production, colloidal properties and biological performance. J Drug Target. 9:361–378.
- Thomas M, Klibanov AM. 2003. Conjugation to gold nanoparticles enhances polyethylenimine's transfer of plasmid DNA into mammalian cells. Proc Natl Acad Sci U S A. 100:9138–9143.
- Wagner E, Cotten M, Foisner R, Birnstiel ML. 1991. Transferrin- polycation-DNA complexes: the effect of polycations on the structure of the complex and DNA delivery to cells. Proc Natl Acad Sci U S A. 88:4255–4259.
- Wang DA, Narang AS, Kotb M, Gaber AO, Miller DD, Kim SW, Mahato RI. 2002. Novel branched poly(ethylenimine)-cholesterol water-soluble lipopolymers for gene delivery. Biomacromolecules. 3:1197–1207.
- Weyermann J, Lochmann D, Georgens C, Zimmer A. 2005. Albumin-protamine-oligonucleotide-nanoparticles as a new antisense delivery system. Part. 2:cellular uptake and effect. Eur J Pharm Biopharm. 59:431–438.
- Xiong S, George S, Yu H, Damoiseaux R, France B, Ng KW, Loo JS. 2013. Size influences the cytotoxicity of poly (lactic-co-glycolic acid) (PLGA) and titanium dioxide (TiO(2)) nanoparticles. Arch Toxicol. 87:1075–1086.
- Yanagishita M, Hascall VC. 1992. Cell surface heparan sulfate proteoglycans. J Biol Chem. 267:9451–9454.
- Zhang G, Yang Z, Lu W, Zhang R, Huang Q, Tian M, et al. 2009. Influence of anchoring ligands and particle size on the colloidal stability and in vivo biodistribution of polyethylene glycol-coated gold nanoparticles in tumor-xenografted mice. Biomaterials. 30:1928–1936.