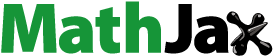
Abstract
Background
The up-regulation of telomerase gene expression occurs in numerous cancers such as breast cancer. A recent study used the PLGA-PEG-helenalin complex, and free helenalin, to inhibit the expression of telomerase in the breast cancer cell line. The purpose of this study was to examine whether nano encapsulating helenalin improves the anti-cancer effect of free helenalin in the T47D breast cancer cell line.
Method
The breast cancer cell line (T47D) was grown in the RPMI 1640 medium, supplemented with 10% FBS. The helenalin was encapsulated by the double emulsion method. Then, the drug loading was calculated and its morphology identified by SEM. Other properties of this copolymer were characterized by Fourier transform infrared (FTIR) spectroscopy and H nuclear magnetic resonance (H NMR) spectroscopy. The assessment of drug cytotoxicity on the growth of the breast cancer cell line was carried out through MTT assay. After treating the cells with a given amount of drug, RNA was extracted and cDNA was synthesized. In order to assess the amount of telomerase gene expression, real-time PCR was performed.
Results
With regard to the amount of the drug loaded, IC50 value was significantly decreased in nanocapsulated (NC) helenalin, in comparison with that of free helenalin. This finding has been proved through the decrease of telomerase gene expression by real-time PCR.
Conclusion
In this study, we demonstrated that the NC-helenalin complex is more effective than free helenalin in inhibiting the growth of breast cancer cells.
Introduction
The incidence of breast cancer in the general population is 10%, and it occurs in 22% of women around the world (CitationHankey 1992, CitationTorbbaghan 1999). In breast cancer and in almost all human cancers, the overexpression of telomerase is the most important factor responsible for the growth and continuous progress of the tumor [Citation3, Citation4](CitationBodnar et al. 1998, CitationKim et al. 1994).
While telomerase activity is absent in most normal human somatic cells, it is seen in more than 90% of the cancer cells and immortal cells (CitationKim et al. 1994, CitationShay and Wright 2000). Telomerase consists of two essential subunits. The first part of the protein is Template RNA, which in humans is called hTR (CitationFeng et al. 1995) that is used as a template for the synthesis of telomeres. The second part, called hTERT, is the catalytic part of the enzyme and has reverse transcriptase activity (CitationKilian et al. 1997, CitationLingner et al. 1997, CitationMeyerson et al. 1997). The expression of hTR is seen in high levels in all tissues, despite telomerase activity. In cancer cells, the typical rate of expression is 5 times higher than that in normal cells (CitationAvilion et al. 1996). In contrast, with respect to the mRNA levels of hTERT, there are less than 1 to 5 copies of the telomerase catalytic subunit per cell, and this is closely related to telomerase activity in cells (CitationYi et al. 1999). Generally, hTERT expression in normal cells is inhibited, and it increases in tumor cells. This shows that hTERT is the determining factor in enzyme activity (CitationAvilion et al. 1996). With regard to telomerase, it is widely expressed in cancer cells, and almost no expression is seen in other normal cells. Thus, telomerase is a suitable therapeutic target in human cancer, including breast cancer, because by inhibiting its expression, tumor progression can be affected (CitationZimmermann and Martens 2007, CitationBlackburn 2000). When this enzyme is inhibited in cancer cells, telomeres shorten during cell division and the cancer cells undergo apoptosis, and ultimately cell death (CitationKyo and Inoue 2002, CitationPoole et al. 2001).
Helenalin, a natural sesquiterpene lactone detected in the alcohol extracted from flowers of Arnica chnamissonis and Arnica Montana, has anticancer and antiinflammatory activity. Helenalin expresses its antiinflammatory properties by producing effects on NF-kB (CitationHuang et al. 2001, CitationLyss et al. 1998). Helenalin is now mostly studied for its anticancer effects. Helenalin causes cell death in leukemia, at concentrations of 10–50 μM (CitationDirsch et al. 2001a, CitationDirsch et al. 2001b).
Helenalin has low solubility in water and has a low bioavailability. To solve this problem, various techniques are used. One of these techniques is the use of nanoparticles. More research on nanoparticles as drug carriers is required. In addition to the increase in drug absorption and bioavailability, the drug is also protected. Nanoparticles include dendrimers, polymers, micelles and liposomes (CitationAli et al. 2011).
Poly (lactic-CO-glycolic acid) (PLGA) is a biodegradable polymer, and is FDA approved, because it is hydrolyzed to monomeric metabolites, lactic acid and glycolic acid.
The encapsulation of anticancer drugs in biodegradable PLGA nanoparticles may pose advantages over other delivery systems, including liposomes. A few of those advantages are well known and have been demonstrated in previous studies: a wide variety of drugs, from those that are extremely hydrophobic to those that are highly hydrophilic (CitationKennedy et al. 2010), can be encapsulated in PLGA nanoparticles, the drug release rates can be modified to particular applications (CitationGrill et al. 2009), and loading and size can be easily manipulated to provide further control over drug delivery (CitationJeong et al. 2000). However, it has been established as being surprisingly difficult to produce surface-modified, drug-encapsulated PLGA particles. For example, the addition of poly(ethylene glycol) (PEG) to nanoparticle surfaces is known to increase circulation time by inhibition of nonspecific protein adsorption, opsonization, and subsequent clearance. This has been well demonstrated with PEGylated liposomes (Yuan et al. 2000, CitationAkbarzadeh et al. 2012a, Citation2012b, Citation2012c, Citation2013, CitationValizadeh et al. 2012), but a wide variety of attempts to similarly modify PLGA nanoparticles have yet to yield comparable results (CitationAkbarzadeh et al. 2012d, CitationMollazade et al. 2013, CitationNejati-Koshki et al. 2013, CitationRezaei-Sadabady et al. 2013, Fallahzadeh et al. 2000). The difficulty in production of PEGylated PLGA particles has been speculated to be the result of insufficient or non-robust surface attachment of PEG. Effective PEGylation of particles implies a high-density coating with PEG, which has been difficult to achieve. The difficulty associated with surface-modification of PLGA particles has been the lack of functional chemical groups on the aliphatic polyester backbone of the polymer. A variety of techniques have been developed for PEGylation of PLGA nanoparticles, such as adsorption, incorporation of polymer conjugates, or covalent connection via amino or carboxyl-terminated PLGA, but these methods suffer from drawbacks such as low density or decreased presentation over time. Recently we have developed a method for surface modification of drug-encapsulated PLGA particles that yields a vigorous and high-density attachment of ligands to the particle surface (CitationEbrahimnezhad et al. 2013, CitationPourhassan-Moghaddam et al. 2013, CitationAhmadi et al. 2014, CitationDavaran et al. 2013, CitationGhasemali et al. 2013).
In this report, we have shown the use of this new technique to produce PEGylated PLGA particles, and have examined their clinical efficacy for the safe and effective delivery of the anticancer agent helenalin (CitationSadat et al. 2014, CitationDavaran et al. 2014, CitationKouhi et al. 2014, CitationAbbasi et al. 2014b, Citation2014b CitationPourhassan-Moghaddam et al. 2014).
Materials and methods
Materials
D, L-lactide and glycolide were purchased from Sigma-Aldrich (St Louis, MO) and recrystallized with ethyl acetate. Stannous octoate (Sn (Oct)2: Stannous 2-ethylhexanoate), PEG (molecular weight 2000), and dimethyl sulfoxide were purchased from Sigma-Aldrich. Adriamaycin hydrochloride was purchased from Sigma-Aldrich. The drug-loading capacity and release behavior were determined using an ultraviolet visible 2550 spectrometer (Shimadzu, Tokyo, Japan). Infrared spectra were recorded in real-time with a PerkinElmer series FTIR. The magnetic property was measured on a vibrating sample magnetometer (Meghnatis Daghigh Kavir, Iran) at room temperature. 1H NMR spectra were recorded in real time with a Brucker DRX 300 spectrometer operated at 400 mHz. The average molecular weight was obtained by gel permeation chromatography (GPC) performed in dichloromethane (CH2Cl2) with a Waters Associates Model ALC/gel permeation chromatography 244 apparatus. The samples were homogenized using a homogenizer (SilentCrusher M, Heidolph Instruments GmbH, Schwabach, Germany). The organic phase was evaporated by rotary (Rotary Evaporators, Heidolph Instruments, Hei-VAP series).
Preparation of PLGA-PEG triblock copolymer
PLGA- PEG2000 copolymer as an initiator was synthesized by a melt polymerization process under vacuum, using stannous octoate [Sn(Oct)2: stannous 2-ethylhexanoate] as a catalyst. DL-lactide (3.6 g), glycolide (0.86 g), and PEG2000 (2 g) (40% w/w), taken in a bottleneck flask, were heated to 140°C under a nitrogen atmosphere for complete melting. The molar ratio of DL-lactide and glycolide was 3:1. Then, 0.05% (w/w) stannous octoate was added, and the temperature of the reaction mixture was raised to 180°C. The temperature was maintained for 3 h. The polymerization was carried out under vacuum. The copolymer was recovered by dissolution in methylene chloride followed by precipitation in ice-cold diethyl ether. A triblock copolymer of PLGA-PEG was prepared by ring opening polymerization of DL-lactide and glycolide in the presence of PEG2000 (CitationBlasco 2001, CitationShay and Woodring 2005, CitationAbbasi et al. 2014d, CitationEatemadi et al. 2014a,Citationb, CitationHosseininasab et al. 2014, CitationDavoudi et al. 2014, Anganeh et al. 2000, CitationAlimirzalu et al. 2014).
Preparation of helenalin-encapsulated PLGA-PEG nanoparticles
Helenalin-encapsulated PLGA-PEG nanoparticles were prepared using the double emulsion method (w/o/w) employed by Song et al. with small modifications. An aqueous solution of helenalin, at a concentration of 5 mg/5 mL, was emulsified in 3 mL dichloromethane, in which 100 mg of the copolymer had been dissolved, using a probe homogenizer or sonicator at 20,000 rpm for 60 s. This w/o emulsion was transferred to 10 mL of an aqueous solution of polyvinyl alcohol (1%), and the mixture was probe-homogenized at 72,000 rpm for one minute. The w/o/w emulsion formed was gently stirred at room temperature until evaporation of the organic phase was completed, or the organic phase was evaporated (Heidolph Instruments). The nanoparticles were purified by applying two cycles of centrifugation (12,000 rpm for 15 min in a Biofuge 28 RS, Heraeus centrifuge) and reconstituted with deionized and distilled water. The nanoparticles were finally filtered through a 1.2 mm filter (Millipore, Bedford, MA). In order to increase helenalin entrapment in the nanoparticles, the external aqueous phase used during the second emulsification step was saturated with helenalin. Blank nanoparticles were also prepared by the same method, without adding helenalin at any stage of the preparation. The content and efficiency of helenalin encapsulation in PLGA-PEG nanoparticles were determined by the disintegration of nanoparticles in dichloromethane (CitationKarnoosh-Yamchi et al. 2014, CitationAlizadeh et al. 2014a,Citationb, CitationNejati-Koshki et al. 2014, CitationAbbasi et al. 2014a,Citationc, CitationEbrahimi et al. 2014, CitationGhalhar et al. 2014, CitationDaraee et al. 2014a). The helenalin concentrations were determined spectrophotometrically at 280 nm. Drug encapsulation efficiency was calculated using the following equations:
Encapsulation Efficiency (%) = [amount of Adriamaycin drug in mg/amount of added drug in mg] × 100%.
Cell culture and cytotoxicity
The human breast cancer cell lines, T47D, were purchased from the Pasteur Institute cell bank of Iran, and were cultured in RPMI1640 supplemented with 10% FBS, 2 mM L-glutamine, and antibiotics (80 mg per 1 l penicillin G, 50 mg per liter streptomycin) and 2 g Na2HCo3 per liter at 37°C in a 5% CO2 incubator.
Cell cytotoxicity was assessed by the 3-(4, 5-dimethylthiazol-2-yl)-2, 5 diphenyl tetrazolium bromide (MTT) assay. Cells (2000 cells/well) were seeded into a 96-well plate for 24 h. NC-helenalin was added at a particular concentration (0 nM/ml–2 nM/ml) and the cells were incubated for 24, 48 and 72 h. After incubation, 50 μL of 2 mg/ml MTT (Sigma Co., Germany) was dissolved in PBS, added to the cells, and allowed to stand for 4 h at 37°C. The absorbance at 570 nm was read using an ELISA plate reader (Bio-Tek Instruments). The NC and DMSO were used as control. This process was repeated three times (triplicate) (CitationTabatabaei Mirakabad et al. 2014, CitationDaraee et al. 2014b, CitationNasrabadi et al. 2014, CitationChung et al. 2014, CitationFekri Aval et al. 2014).
Cell treatment
Cells were treated with NC-helenalin at various concentrations for 24, 48 and 72 h, after calculating the IC50. After this period, cells were harvested, and the entire process given was used as control, with 10% DMSO, without the NC− helenalin complex.
RNA extraction and cDNA synthesis
In order to extract RNA, we used a Trizol kit that needs approximately one million cells in each flask. To do this, the micro tubes and tips should be RNase-free. To ensure the purity of the extracted RNA, it was measured at a wavelength of 260–280 nm using a spectrophotometer. To ensure the extraction of RNA, we subjected it to electrophoresis in agarose gel (2%), and observed its specific band. cDNA synthesis was contacted based on the protocols proposed in the Fermentas kit. In this method, we used the RT enzyme and oligo (dt) polymers.
Real time PCR
In this technique, cDNA is amplified with particular primers of the telomerase gene and the internal control gene (β-actin).
Corbett 6000 was the instrument used to perform real time PCR.
The information related to the sequence and length of primers and the accession numbers are shown in .
Table I. Forward (F) and reverse (R) primer sequences, and product size base pairs (bp) of the Housekeeping genes β-actin and telomerase.
In order to analyze the data and quantitatively assess gene expression, we first calculated the value for cycle threshold (ct) for each sample, and since it was duplicated, we calculated the mean of the ct values.
Statistical analysis
To compare the significance of differences in the levels of gene expression, treated and control cells were subjected to the ANOVA test. All statistical analyses were carried out using SPSS statistical software, version 14. This study is significant because the P value obtained is lower than 0.05.
Results
Measurement and characterization of nanoparticles
FT-IR spectroscopy
The FT-IR spectrum is consistent with the structure of the expected copolymer. FT-IR spectroscopy was used to show the structure of PLGA-PEG copolymer nanoparticles. From the IR spectra presented in , the absorption band at 3509.9 cm− 1 is attributed to the terminal hydroxyl groups in the copolymer from which the PEG homopolymer has been removed. The bands at 3010 cm− 1 and 2955 cm− 1 and 2885 cm− 1 are due to the C–H stretch of CH, a strong band at 1762.6 cm− 1 is assigned to the C = O stretch. The absorption at 1186–1089.6 cm− 1 is due to the C–O stretch ().
Table II. FT-IR bands of PLGA-PEG copolymer.
1H NMR spectrum of PEG-PLGA copolymer
The basic chemical structure of the PEG-PLGA copolymer is confirmed by the 1H NMR spectra that were recorded at RT with a Brucker DRX 300 spectrometer operating at 400 MHz. The chemical shift (δ) was measured in ppm using tetramethylsilane (TMS) as an internal reference (). One of the striking features is a large peak at 3.65 ppm, corresponding to the methylene groups of the PEG. Overlapping doublets at 1.55 ppm are attributed to the methyl groups of the repeating units of D-lactic acid and L-lactic acid. The multiple peaks at 5.2 and 4.8 ppm correspond to the lactic acid CH and the glycolic acid CH respectively, with the high complexity of the two peaks resulting from different D-lactic, L-lactic, and glycolic acid sequences in the polymer backbone ().
GPC chromatogram of the PEG-PLGA copolymer
Molecular weights and the molecular weight distribution of the copolymer obtained were determined by means of gel permeation chromatography (GPC). The average molecular weight was 16400. The unimodal mass distribution excluded the presence of PEG2000 and PEG4000 or PLGA)Mw: 16400, Mn: 7131, Mz: 20300, Mp: 6850).
Physicochemical characterization of nanoparticles
In order to investigate the physicochemical characterization of nanoparticles prepared by the double emulsion method (w/o/w), the nanoparticles were observed by scanning election microscopy (SEM) (). It was seen from these micrographs that the nanoparticles of helenalin entrapped in PLGA–PEG were spherical in shape and uniform, with the size ranging from 30 nm–60 nm. The encapsulation efficiency values achieved for helenalin were influenced by the presence of PEG with different molecular weights in PLGA chains (). The values of the zeta potential were obviously affected by the presence of different PEG chains of different molecular weights. Higher negative values were obtained for PLGA-PEG nanoparticles (-33.2 mV).
Table III. The values of encapsulation efficiency achieved for helenalin and the presence of PEG- PLGA with different molecular weights.
Size and size distribution (SEM)
The surface morphologies of nanospheres during the incubation period were observed by SEM (VEGA/TESCAN). The SEM nanographs of helenalin-loaded PLGA–PEG copolymer nanoparticles are shown in . Observing the photograph, it can be seen that after encapsulation and the formation of helenalin-loaded PLGA–PEG copolymers, the size of the particles was changed, and ranged between 35 nm–85 nm, and the dispersion of particles was greatly improved, which can be explained by the electrostatic repulsive force and steric hindrance between the copolymer chains on the encapsulated nanoparticles. The samples were coated with gold particles.
Figure 4. (A) The comparison of the cytotoxic effect of free helenalin (left) and NC-helenalin (right) on T47D cells over a 24-h exposure. This graph shows that both free helenalin and NC-helenalin have not IC50 in the 24-h exposure on T47D cells, within the concentration limits. (B) The comparison of the cytotoxic effect of free helenalin (left) and NC-helenalin (right) on T47D cells over a 48-h exposure. This graph shows that free helenalin has no IC50 in the 48 h exposure for T47D cells within the concentration limits, but NC-helenalin has IC50 in 48 h (0.16 μM). (C) A comparison of the cytotoxic effect of free helenalin (left) and NC-helenalin (right) on T47D cells over a 72-h exposure. This graph shows that the IC50 of free helenalin is about 1.3 μM and that of NC-helenalin is about 0.14 μM, over a 72-h exposure for T47D cells.
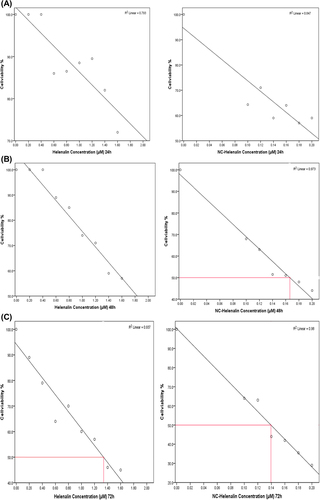
Drug loading and determination of helenalin entrapment efficiency
The anticancer drug helenalin was used for the drug loading and release studies. In brief, 20 mg of lyophilized nanoparticles and 5 mg of helenalin were dispersed in phosphate-buffered solution. The solution was stirred at 4°C for 3 days, to allow helenalin to be entrapped within the nanoparticle network. This value was then compared with the total amount of helenalin, to determine the helenalin-loading efficiency of the nanoparticles. The amount of non-entrapped helenalin in aqueous phase was determined using an ultraviolet 2550 (λex 280 nm and λem 485 nm) spectrophotometer (Shimadzu). This procedure permits the analysis of a helenalin solution with removal of most interfering substances (CitationKouhi et al. 2014). The amount of helenalin entrapped within the nanoparticles was calculated by the difference between the total amount used to prepare the nanoparticles and the amount of helenalin present in the aqueous phase, using the following formula:
In vitro cytotoxicity study (MTT assay)
In the cytotoxicity assay, T47D cell lines were treated with free helenalin and increasing concentration of NC-helenalin (1–1.8 μM) for 3 days (24, 48 and 72 h). The value of inhibitory concentration 50 (IC50) was then calculated for each case.
Significant differences in the growth pattern were found between control and cells treated with free helenalin or NC-helenalin.
The IC50 value of free helenalin was 1.3 μM for 72 h. The IC50 value of NC-helenalin was 0.16 μM for 48 h, and 0.14 μM for 72 h, respectively.
The , and C represent the comparison of the IC50 values of free helenalin and NC-helenalin with respect to time.
Real-time PCR for hTERT gene expression
Quantitative gene expression (hTERT) was examined by real-time PCR assay in the T47D cell lines after treatment with free helenalin and NC-helenalin, and compared with controls.
The housekeeping gene (β actin) was used as a reference gene. Each sample was duplicated twice.
The relative levels of hTERT gene expression were calculated through the ∆∆CT method.
With regard to the data analysis shown in and B, free helenalin, with a concentration of 13 nM in 72 h, and NC-helenalin, with a concentration of 1.6 nM in 72 h, showed significantly decreased gene expression.
Discussion
Today, the most important issue in cancer therapy is drug delivery, to eliminate cancer cells in a manner that has the lowest side effect on healthy cells.
Although various methods such as chemotherapy, hormone therapy and gene therapy are used for treating cancer (CitationKennedy et al. 2010), the best method, which has been popular in recent years, is the use of nanoparticles for the delivery of drugs to cancer tissue. This is more effective than using free drugs, and due to the use of low dose of drug, the toxicity is decreased. Nano carriers have several advantages, including the fact that they can take more of the drug, and they will protect and enhance the solubility of the drug, while avoiding toxicity to normal cells (CitationGrill et al. 2009). For this purpose, this study focused on the use of polymers as nanoparticles. The speed with which nanoparticles undergo degradation in organisms has some advantages, since it quickly eliminates polymers in the body (CitationJeong et al. 2000).
One of the reasons for choosing PLGA-PEG is that it sits in the aquatic environment easily and sits at the level of the nanoparticle, while PLGA of this copolymer sits in the hydrophobic part of the nanoparticle (Yuan et al. 2000).
In this study, after sufficient growth, the breast cancer cell line (T47D) in the RPMI 1640 medium was exposed to various concentrations of free helenalin and NC-helenalin, for different time durations (24, 48, and 72 h).
After calculating IC50, the T47d cell line, with different concentrations of free helenalin and NC-helenalin, was treated with control. Then, hTERT gene expression was assessed by real-time PCR.
The data obtained from MTT analysis indicates that IC50 of free helenalin in the T47D cell line is time-dependent, but IC50 in NC-helenalin is both dose-dependent and time-dependent.
The assessment of hTERT gene expression by real-time PCR showed that hTERT gene expression decreases on exposure to both free helenalin and NC-helenalin, when concentration and time are increased. The greatest decrease of gene expression on exposure to free helenalin is seen at a concentration of 13 nM for 72 h, and the greatest decrease of gene expression on exposure to NC-helenalin occurs at a concentration of 1.4 nM for 72 h.
The expression of the hTERT gene in the control sample shows that nanoparticles generally do not have any toxic effects on cell cultures.
To load drugs, the different polymers of PLGA-PEG 2000, 3000 and 2000 were used in previous studies. PLGA-PEG 2000 was found to have the largest drug load (CitationAkbarzadeh et al. 2012a), and that why it was used to form the nanoparticles.
With regard to the amount of drug loaded and the results obtained from MTT and real-time PCR studies,we found that by using nano particles made with the PLGA-PEG polymer as drug carriers with a free drug (helenalin) concentration (0.1 nM), approximately the same result can be obtained to cure cancer in in vitro.
Conclusion
This study showed that helenalin has a significant inhibitory effect on the growth of breast cancer cell line (T47D). Using nanocapsules, the dose of helenalin administered could be reduced, without reducing its therapeutic effect. Therefore, encapsulated helenalin preserved its full function.
The polymer which been used as a nanoparticle has the least toxic effect on cells, while on the other hand, it carries the greatest drug load.
This nanocapsule can be used in the future as a suitable carrier in target therapy for cure of breast cancer.
Authors’ contributions
MR conceived of the study and participated in its design and coordination. NZ and A.A participated in the sequence alignment and drafted the manuscript. All authors read and approved the final manuscript.
Acknowledgments
The authors are grateful to the financial support from Iran National Science Foundation, Drug Applied Research Center, Tabriz university of Medical Sciences, and The Department of Medicinal Chemistry, Tabriz University of Medical Sciences. The authors thank the Department of Medical Nanotechnology, Faculty of Advanced Medical Science of Tabriz University, for all support provided.
Declaration of interest
The authors report no declarations of interest. The authors alone are responsible for the content and writing of the paper.
This work is funded by the 2012 Yeungnam University Research Grant.
References
- Abbasi E, Akbarzadeh A, Kouhi M, Milani M. 2014a. Graphene: synthesis, bio-applications, and properties. Artif Cells Nanomed Biotechnol. 30:1–7.
- Abbasi E, Aval SF, Akbarzadeh A, Milani M, Nasrabadi HT, Hanifepour Y, et al. 2014b. Dendrimers: synthesis, applications, and properties. Nanoscale Res Lett. 9:247.
- Abbasi E, Milani M, Fekri Aval S, Kouhi M, Akbarzadeh A, Nasrabadi HT, et al. 2014c. Silver nanoparticles: Synthesis, properties, bio-applications and limitations. Crit Rev Microbiol. (Epub ahead of print).
- Abbasi E, Milani M, Fekri Aval S, Kouhi M, Akbarzadeh A, Tayefi Nasrabadi H. 2014d. Silver nanoparticles: synthesis, properties, bio-applications and limitations. Crit Rev Microbiol(Epub ahead of print).
- Ahmadi A, Shirazi H, Pourbagher N, Akbarzadeh A, Omidfar K 2014. An electrochemical immunosensor for digoxin using core-shell gold coated magnetic nanoparticles as labels. Mol Biol Rep. 41:1659–1668.
- Akbarzadeh A, Mikaeili H, Asgari D, Zarghami N, Mohammad R, Davaran S. 2012b. Preparation and in-vitro evaluation of doxorubicin-loaded Fe3O4 magnetic nanoparticles modified with biocompatible copolymers Int J Nanomedicine 7:511–526.
- Akbarzadeh A, Rezaei-Sadabady R, Davaran S, Joo SW, Zarghami N, Hanifehpour Y, et al. 2013. Liposome: classification, preparation, and applications. Nanoscale Res Lett 8:102.
- Akbarzadeh A, Samiei M, Davaran S. 2012a. Magnetic nanoparticles: preparation, physical properties and applications in biomedicine Nanoscale Res Lett 7:144–157.
- Akbarzadeh A, Samiei M, Joo SW, Anzaby M, Hanifehpour Y, Nasrabadi HT, Davaran S 2012d. Synthesis, characterization and in vitro studies of doxorubicin-loaded magnetic nanoparticles grafted to smart copolymers on A549 lung cancer cell line J Nanobiotechnol 10:46–58.
- Akbarzadeh A, Zarghami N, Mikaeili H, Asgari D, Goganian AM, Khiabani HK, Davaran S. 2012c. Synthesis, characterization and in vitro evaluation of novel polymer-coated magnetic nanoparticles for controlled delivery of doxorubicin. Int J Nanotechnol Sci Environ 5:13–25.
- Ali I, Rahis-Uddin, Salim K, Rather MA, Wani WA, Haque A. 2011. Advances in nano drugs for cancer chemotherapy. Curr Cancer Drug Targets. 11:135–146.
- Alimirzalu S, Akbarzadeh A, Abbasian M, Alimohammadi S, Davaran S, Hanifehpour Y, et al. 2014. Synthesis and study of physicochemical characteristics of Fe3O4 magnetic nanocomposites based on poly(Nisopropylacrylamide) for anti-cancer drugs delivery. Asian Pac J Cancer Prev, 15:49–54.
- Alizadeh E, Akbarzadeh A, Eslaminejad MB, Barzegar A, Hashemzadeh S, Nejati-Koshki K, Zarghami N. 2014a. Up-regulation of Liver enriched Transcription Factors (HNF4a and HNF6) and Liver Specific MicroRNA (miR-122) by Inhibition of Let-7b in Mesenchymal Stem Cells. Chem Biol Drug Des 85:600–608.
- Alizadeh E, Zarghami N, Eslaminejad MB, Akbarzadeh A, Barzegar A, Mohammadi SA. 2014b. The effect of dimethyl sulfoxide (DMSO) on hepatic differentiation of mesenchymal stem cells. Artif Cells Nanomed Biotechnol. 30:1–8.
- Anganeh MT, Sadat Tabatabaei Mirakabad F, Izadi M, Zeighamian V, Badrzadeh F, Salehi R, et al 2014. The comparison between effects of free curcumin and curcumin loaded PLGA-PEG on telomerase and TRF1 expressions in calu-6 lung cancer cell line. Int J Biosci. 4:134–145.
- Avilion AA, Piatyszek MA, Gupta J, Shay JW, Bacchetti S, Greider CW. 1996. Human telomerase RNA and telomerase activity in immortal cell lines and tumor tissues. Cancer Res. 56:645–650.
- Blackburn E. 2000. The end of the (DNA) line. Nat Struct Biol. 7: 847–850.
- Blasco MA. 2001. Telomerase in cancer therapy. J Biomed Biotechnol. 1:3–4.
- Bodnar AG, Ouellette M, Frolkis M, Holt SE, Chiu CP, Morin GB, et al. 1998. Extension of life-span by introduction of telomerase into normal human cells. Science. 279:396–352.
- Chung JH, Kim YK, Kim KH, Kwon TY, Vaezmomeni SZ, Samiei M, et al. 2014. Synthesis, characterization, biocompatibility of hydroxyapatite-natural polymers nanocomposites for dentistry applications. Artif Cells Nanomed Biotechnol. 1–8.
- Daraee H, Eatemadi A, Abbasi E, Fekri Aval S, Kouhi M, Akbarzadeh A. 2014a. Application of gold nanoparticles in biomedical and drug delivery. Artif Cells Nanomed Biotechnol. 1–13.
- Daraee H, Etemadi A, Kouhi M, Alimirzalu S, Akbarzadeh A. 2014b. Application of liposomes in medicine and drug delivery. Artif Cells Nanomed Biotechnol. 1–11.
- Davaran S, Akbarzadeh A, Nejati-Koshki K, Alimohammadi S, Ghamari MF, Soghrati MM, et al 2013. In vitro studies of NIPAAM-MAA-VP copolymer-coated magnetic nanoparticles for controlled anticancer drug release J Encapsulation Adsorpt Sci 3:108–115.
- Davaran S, Rezaei A, Alimohammadi S, Khandaghi AA, Nejati- Koshki K, Nasrabadi HT, Akbarzadeh A 2014. Synthesis and physicochemical characterization of biodegradable star-shaped poly lactide-co-glycolide– β-cyclodextrin copolymer nanoparticles containing albumin Adv Nanopart 3:14–22.
- Davoudi Z, Akbarzadeh A, Rahmatiyamchi M, Movassaghpour AA, Alipour M, Nejati-Koshki K, et al. 2014. Molecular target therapy of AKT and NF-kB signaling pathways and multidrug resistance by specific cell penetrating inhibitor peptides in HL-60 cells. Asian Pac J Cancer Prev. 15:4353.
- Dirsch VM, Stuppner H, Vollmar AM. 2001a. Cytotoxic sesquiterpene lactones mediate their death-inducing effect in leukemia T cells by triggering apoptosis. Planta Med. 67:557–559.
- Dirsch VM, Stuppner H, Vollmar AM. 2001b. Helenalin triggers a CD95 death receptor-independent apoptosis that is not affected by over expression of Bcl-x(L) or Bcl-2. Cancer Res. 61:5817–5823.
- Eatemadi A, Daraee H, Karimkhanloo H, Kouhi M, Zarghami N, Akbarzadeh A, et al. 2014a. Carbon nanotubes: properties, synthesis, purification, and medical applications. Nanoscale Res Lett. 9:1–13.
- Eatemadi A, Daraee H, Zarghami N, Yar HM, Akbarzadeh A, Hanifehpour Y. 2014b. Nanofiber; synthesis and biomedical applications. Artif Cells Nanomed Biotechnol. (Epub ahead of print).
- Ebrahimi E, Akbarzadeh A, Abbasi E, Khandaghi AA, Abasalizadeh F, Davaran S. 2014. Novel drug delivery system based on doxorubicin-encapsulated magnetic nanoparticles modified with PLGA-PEG1000 copolymer. Artif Cells Nanomed Biotechnol.(Epub ahead of print).
- Ebrahimnezhad Z, Zarghami N, Keyhani M, Amirsaadat S, Akbarzadeh A, Rahmati M, et al. 2013. Inhibition of hTERT gene expression by silibinin-loaded PLGA-PEG-Fe3O4 in T47D breast cancer cell line. BioImpacts 3:67–74.
- Fallahzadeh S, Bahrami H, Akbarzadeh A, Tayarani M. 2014. High-isolation dual-frequency operation patch antenna using spiral defected microstrip structure. Antennas and Wireless Propagation Letters. IEEE 9:122–124.
- Fekri Aval S, Akbarzadeh A, Yamchi MR, Zarghami F, Nejati-Koshki K, Zarghami N. 2014. Gene silencing effect of SiRNA-magnetic modified with biodegradable copolymer nanoparticles on hTERT gene expression in lung cancer cell line. Artif Cells Nanomed Biotechnol. 1–6.
- Feng J, Funk WD, Wang SS, Weinrich SL, Avilion AA, Chiu CP, et al. 1995. The RNA component of human telomerase. Science. 269:1236–1241.
- Ghalhar MG, Akbarzadeh A, Rahmati M, Mellatyar H, Dariushnejad H, Zarghami N, Barkhordari A. 2014. Comparison of inhibitory effects of 17-AAG nanoparticles and free 17-AAG on HSP90 gene expression in breast cancer. Asian Pac J Cancer Prev. 15:7113–7118.
- Ghasemali S, Nejati-Koshki K, Akbarzadeh A, Tafsiri E, Zarghami N, Rahmati-Yamchi M, Alizadeh E, et al. 2013. Study of inhibitory effect of β-cyclodextrin-helenalin complex on HTERT gene expression in T47D breast cancer cell line by real time quantitative PCR (q-PCR) Asian Pac J Cancer Prev 14:6949–6953.
- Grill AE, Johnston NW, Sadhukha T, Panyam J. 2009. A review of select recent patents on novel nanocarriers. Recent Pat Drug Deliver Formul 3:137–142.
- Hankey. 1992. Cancer statistics review 1973–1989. National Cancer Institute, NIH Pub. 92–2789:1–8.
- Hosseininasab S, Pashaei‐Asl R, Khandaghi AA, Nasrabadi HT, Nejati‐Koshki K, Akbarzadeh A, et al. 2014. Synthesis, characterization, and In vitro studies of PLGA‐PEG nanoparticles for oral Insulin delivery. Chem Biol Drug Des. 84:307–315.
- Huang PR, Yeh YM, Wang TC. 2001. Potent inhibition of human telomerase by helenalin. Cancer Lett. 227:169–174.
- Jeong JH, Lim DW, Han DK, Park TG. 2000. Synthesis, characterization and protein adsorption behaviors of PLAGA/PEG di-block co-polymer blend films. Colloids Surf B Biointerfaces. 18:371–379.
- Karnoosh-Yamchi J, Mobasseri M, Akbarzadeh A, Davaran S, Ostad-Rahimi AR, Hamishehkar H, et al. 2014. Preparation of pH sensitive insulin-loaded nano hydrogels and evaluation of insulin releasing in different pH conditions. Mol Biol Rep. 41: 6705–6712.
- Kennedy S, Geradts J, Bydlon T, Brown JQ, Gallagher J, Junker M, et al. 2010. Optical breast cancer margin assessment: an observational study of the effects of tissue heterogeneity on optical contrast. Breast Cancer Res. 12:1–36.
- Kilian A, Bowtell DD, Abud HE, Hime GR, Venter DJ, Keese PK, et al. 1997. Isolation of a candidate human telomerase catalytic subunit gene, which reveals complex splicing patterns in different cell types. Hum Mol Genet. 6:2011–2019.
- Kim NW, Piatyszek MA, Prowse KR, Harley CB, West MD, Ho PL, et al. 1994. Specific association of human telomerase activity with immortal cells and cancer. Science. 266:2011–2015.
- Kouhi M, Vahedi A, Akbarzadeh A, Hanifehpour Y, Joo SW. 2014. Investigation of quadratic electro-optic effects and electro absorption process in GaN/AlGaN spherical quantum dot. Nanoscale Res Lett. 9:131–136.
- Kyo S, Inoue M. 2002. Complex regulatory mechanisms of telomerase activity in normal and cancer cells: how can we apply them for cancer therapy? Oncogene. 21:688–697.
- Lingner J, Hughes TR, Shevchenko A, Mann M, Lundblad V, Cech TR. 1997. Reverse transcriptase motifs in the catalytic subunit of telomerase. Science. 276:561–567.
- Lyss G, Knorre A, Schmidt TJ, Pahl HL, Merfort I. 1998. The anti- inflammatory sesquiterpene lactone Helenalin inhibits the transcription factor NF-kappa B by directly targeting p65. J Biol Chem 273:33508–33516.
- Meyerson M, Counter CM, Eaton EN, Ellisen LW, Steiner P, Caddle SD. 1997. hEST2, the putative human telomerase catalytic subunit gene, is unregulated in tumor cells and during immortalization. Cell. 90:785–795.
- Mollazade M, Nejati-Koshki K, Akbarzadeh A, Zarghami N, Nasiri M, Jahanban-Esfahlan R, Alibakhshi A. 2013. PAMAM dendrimers arugment inhibitory effect of curcumin on cancer cell proliferation: possible inhibition of telomerase. Asian Pac J Cancer Prev 14:6925–6928.
- Nasrabadi HT, Abbasi E, Davaran S, Kouhi M, Akbarzadeh A. 2014. Bimetallic nanoparticles: Preparation, properties, and biomedical applications. Artif Cells Nanomed Biotechnol. 1–5.
- Nejati-Koshki K, Akbarzadeh A, Pourhasan-Moghadam M, Abhari A, Dariushnejad H. 2013. Inhibition of leptin and leptin receptor gene expression by silibinin-curcumin combination. Asian Pac J Cancer Prev 14:6595–6599.
- Nejati-Koshki K, Mesgari M, Ebrahimi E, Abbasalizadeh F, Fekri Aval S, Khandaghi AA, et al. 2014. Synthesis and in vitro study of cisplatin-loaded Fe3O4 nanoparticles modified with PLGA-PEG6000 copolymers in treatment of lung cancer. J Microencapsul. 31:815–823.
- Poole JC, Andrews LG, Tollefsbol TO. 2001. Activity, function, and gene regulation of the catalytic subunit of telomerase (hTERT). Gene. 269:1–12.
- Pourhassan-Moghaddam M, Rahmati-Yamchi M, Akbarzadeh A, Daraee H, Nejati-Koshki K, Hanifehpour Y, Joo SW. 2013. Protein detection through different platforms of immuno-loop-mediated isothermal amplification. Nanoscale Res Lett. 8:485.
- Pourhassan-Moghaddam M, Zarghami N, Mohsenifar A, Rahmati-Yamchi M, Gholizadeh D, Akbarzadeh A, et al. 2014. Watercress-based gold nanoparticles: biosynthesis, mechanism of formation and study of their biocompatibility in vitro. IET Dig Lib 4:5.
- Rezaei-Sadabady R, Zarghami N, Barzegar A, Eidi A, Akbarzadeh A, Rezaei-Tavirani M. 2013. Studies of the relationship between structure and antioxidant activity in interesting systems, ncluding tyrosol, hydroxytyrosol derivatives indicated by quantum chemical calculations. Soft 2:13–18.
- Sadat Tabatabaei Mirakabad F, Akbarzadeh A, Zarghami N, Zeighamian V, Rahimzadeh A, Alimohammadi S 2014. PLGA-based nanoparticles as cancer drug delivery systems Asian Pac J Cancer Prev. 15:517–535.
- Shay JW, Woodring WE. 2005. Senescence and immortalization: role of telomeres and telomerase. Carcinogenesis. 26:867–874.
- Shay JW, Wright WE. 2000. Hayflick, his limit, and cellular aging. Nat Rev Mol Cell Boil. 1:72–76.
- Tabatabaei Mirakabad FS, Akbarzadeh A, Milani M, Zarghami N, Taheri-Anganeh M, Zeighamian V, et al. 2014. A Comparison between the cytotoxic effects of pure curcumin and curcumin-loaded PLGA-PEG nanoparticles on the MCF-7 human breast cancer cell line. Artif Cell Nanomed Biotechnol. 1–8.
- Torbbaghan S. 1999. Annual report of Tehran University of medical sciences district cancer registry (TUMS-DCR). The cancer institute publication, No. 7, 43.
- Valizadeh A, Mikaeili H, Samiei M, Farkhani SM, Zarghami N, Kouhi M, et al. 2012. Quantum dots: synthesis, bioapplications, and toxicity. Nanoscale Res Lett 7:276.
- Yi X, Tesmer VM, Savre-Train I, Shay JW, Wright WE. 1999. Both transcriptional and posttranscriptional mechanisms regulate human telomerase template RNA levels. Mol Cell Boil. 19:3989–3997.
- Yuan M, Wang Y, Li X, Xiong C, Deng X. 2014. Polymerization of lactides and lactones. Synthesis, characterization, and application of amino–terminated poly (ethylene glycol)–co–poly ([e]–caprolactone) block copolymer. Macromolecules. 33:1613–1617.
- Zimmermann S, Martens UM. 2007. Telomeres and telomerase as targets for cancer therapy. Cell Mol Life Sci. 64:906–921.