Abstract
This study aims to look into the applicability of a porous TiNi-based shape memory alloy (SMA) scaffold as an incubator for bone marrow mesenchymal cells, hepatocytes, and pancreatic islet cells. The porous TiNi-based SMA used was fabricated using a self-propagating high-temperature synthesis (SHS) technique, in which scaffold blocks measuring 4 × 4 × 10 mm were prepared. In vitro tests were done using mesenchymal stem cells (MSC) isolated from mature bone marrow of CBA/j inbred mice, and cultured in 3 different culture media – Control medium, Osteogenic medium, and Chondrogenic medium. Hepatocytes and islet cells were isolated from the livers and pancreatic glands of Wistar rats respectively, seeded on porous TiNi-based SMA scaffolds, and cultured. The scaffolds were then implanted into the abdominal cavity of Wistar rats and later harvested, at days 7, 14, 21, and 28, post-implantation. SEM imaging was performed with pre-implanted scaffolds at day 0 and harvested scaffolds at days 7, 14, 21, and 28, post-implantation. Based on weight increase percentages, the in vitro study revealed that the osteogenic group showed a 2-fold increase, and the chondrogenic group showed a 1.33-fold increase, compared to the control group. The in vivo study, on the other hand, showed that from day 7 post-implantation, the cellular in-growth gradually invaded the inner porous structure from the periphery towards the center, and at day-28 post-implantation, all pores were closed and completely filled with cells and the extracellular matrix. The results show that porous TiNi-based SMA is a unique biocompatible incubator for cell cultures and can be successfully used for tissue bioengineering and artificial organs.
Introduction
The field of tissue engineering exploits living cells in a variety of ways to restore, maintain, or enhance tissues and organs. To engineer living tissues in vitro, cultured cells are coaxed to grow on bioactive degradable scaffolds that provide the physical and chemical cues to guide their differentiation and assembly into three-dimensional (3D) tissues (CitationGriffith and Naughton 2014). Advances in tissue engineering include the development of porous, biocompatible, three-dimensional scaffolds. Regardless of the application, the scaffold should be biocompatible and imitate both the physical and the biological function of the native extracellular matrix (ECM), as the ECM provides a substrate with specific ligands for cell adhesion, as well as physical support for cells (CitationMurphy and O’Brien 2010). The pore size within a scaffold affects initial cell adhesion. Cell adhesion mediates all subsequent events such as proliferation, migration, and differentiation within the scaffold. Scaffolds must be permeable, with interconnecting pores to facilitate cell growth, migration, and nutrient flow. Therefore, maintaining a balance between the optimal pore size for cell migration and the specific surface area for cell attachment is essential. TiNi-based alloys have a unique thermal shape memory effect, super-elasticity, good corrosion resistance, and high damping properties. Other characteristics include wear characteristics, mechanical properties, and a good biocompatibility (CitationGunther et al. 2014). These properties make them ideal biomaterials, especially in orthopedics. A TiNi-based alloy with a relatively higher elastic modulus and strength can be produced to match the human compact bone (CitationXiong et al. 2008). Recently, the biocompatibility of TiNi-based SMA has been clarified in well-designed studies. Implants using TiNi-based SMA have been developed, and are widely used in cardiovascular and gastrointestinal applications (CitationKang et al. 2002, CitationGunther et al. 2011, CitationDambaev et al. 2012, CitationChoinzonov et al.).
The increase of degenerative diseases and injuries, as well as expensive surgery, brings us to research related to the creation of scaffolds for cell cultures of different bones and cartilage. The actual aims of modern replacement therapy using stem cell technologies are the cultivation of pancreatic islet cells for treatment of diabetes mellitus, and hepatocytes for correction of liver failure (CitationKokorev et al. 2012a, Citation2012b).
In the Research Institute of Medical Materials, porous-permeable materials of TiNi-based alloy were created, for their use as scaffolds for cell cultures of different organs. Porous and cast TiNi-based alloys meet many strict requirements, which are necessary for medical applications: having physical and mechanical properties, being Footnote1non-toxic and non-carcinogenic, having no inflammatory or immune response, etc. Porous TiNi-based SMA can be fabricated using special engineering techniques. A porous TiNi-based SMA retains the unique properties of the solid TiNi-based SMA, and has the additional benefits of porous biomaterials such as low density, high surface area, and high permeability. Advanced techniques enable control over porosity and pore size in the porous TiNi-based SMA (CitationGunther et al. 2000, CitationMuhamedov et al. 2014).
The aim of this study is to research the applicability of a porous TiNi-based SMA scaffold as the incubator for bone marrow mesenchymal cells, hepatocytes, and pancreatic islet cells.
Materials and methods
Porous TiNi-based SMA
A porous TiNi-based SMA was fabricated using a self- propagating high-temperature synthesis (SHS) technique, at the Research Institute of Medical Materials. The pore size in the porous TiNi-based SMA was controlled by adjusting the fabrication conditions. Scaffold blocks (4x4x10 mm) of porous TiNi-based SMA were prepared by electric-discharge (ED) wire-cut. An analysis of the pore structure of the scaffold was performed using Hg-porosimetry, the Axiovert 40 MAT metallographic microscope, and the Quanta 200 3D scanning electron microscope (SEM). Prior to soaking into culture medium, the scaffolds were degreased with 70% ethanol, washed in an ultrasonic bath, and autoclaved at 180oC for 1 h. The initial weight of each scaffold was measured precisely (CitationKang et al. 2002, CitationKim et al. 2004, CitationBansiddhi et al. 2008).
Laboratory animals
All procedures on animals were carefully carried out, with strict adherence to the European Convention for the Protection of Vertebrate Animals used for Experimental and other Scientific Purposes (Strasburg, 1986), and with the European Communities Council Directive 86/609/EEC. CBA/j inbred mice and Wistar rats were used (10-week-old, males)
The mesenchymal stem cell (MSC) growth in scaffolds (in vitro)
MSCs were isolated from mature bone marrow of the CBA/j inbred mice. Bone marrow aspirates were diluted in the expansion medium (DMEM supplemented with 10% FBS, 0.1 mM non-essential amino acids, and 2.5 mg/ml Fungizone) and plated at a density of 2 × 105 cells/cm2 in flasks. Non- adherent cells were removed with subsequent media changes, and the adherent cells (MSCs) were trypsinized on attaining 80% confluence. Three different media were established for differentiation: (a) a control medium (DMEM with 10% FBS, 40mkg/ml gentamycin, and 250mg/l glutamine) for control, (b) an osteogenic medium (control medium supplemented with 50 мg/ml ascorbic acid-2-phosphate, 10 nM dexamethasone, 7 mM в-glycerophosphate and 1 mg/ml rhBMP-2 (bone marrow protein) for osteogenic differentiation, and (c) a chondrogenic medium (control medium supplemented with 0.1 mM non-essential amino acids, 5 mg/ml insulin and 5 ng/m hTGF-в ) for chondrogenic differentiation,.
To investigate the in vitro responses of MSCs on porous TiNi-based SMA scaffolds, the scaffolds were immersed in 50 ml plastic vials, which were filled with three different d ifferentiation media with MSCs at a density of 5 × 106 cells/ml. The scaffolds kept in the plastic vials were cultured at 37°C and 100% humidity with 5% CO2. The medium was replenished twice a week. All cultures were terminated at day 28.
Each of the cultured scaffolds was removed from the culture medium, washed with plenty of distilled water, then dehydrated with 99% ethanol, and dried overnight in an oven at 35°C. The dry weight of each cultured scaffold was measured. The weight increase percentage was calculated with the dry weight of cultured scaffold and the initial weight of the scaffold.
DNA was extracted from the scaffolds in the following manner. The scaffolds, frozen in 3 ml of ddH2O, were thawed and sonicated for 30 s, to release the alkaline phosphatase (ALP) activity and deoxyribonucleic acid (DNA) concentration into the solution. The solution was centrifuged for 5 min at 500g to remove scaffold debris. DNA was extracted from 850 ml of the solution, and the rest was kept for the ALP activity assay. Ninety-five ml of heated 10 X TE buffer with 1.5M NaCl and 5% SDS were added to the DNA portion. Then, the solution was treated with RNase (20мg) and Proteinase K (800мg). The solution was extracted with phenol-chloroform, and DNA was precipitated with isopropanol, washed with 70% ethanol, and dissolved in TE buffer. The amount and purity of DNA per scaffold was determined by absorbance readings at 260nm and 280nm in a UV/VIS spectrophotometer (Cecil Instruments, Cambridge, England) using quartz microcuvettes (Hellma, Müllheim, Germany).
Alkaline phosphatase activity (osteoblastic phenotypic marker) was evaluated using the p-nitrophenol method with the rest of the centrifuged solution. The rate of p-nitrophenol production is proportional to the amount of ALP in the solution. A standard dilution series of p-nitrophenol (Sigma-Aldrich, Brøndby, Denmark) was prepared, with concentrations ranging from 0 to 0.5mM. In a 96-well plate, 40 ml of the solution or a standard dilution of p-nitrophenol was added to 60 ml of 2-amino-2-methyl-1-propanol buffer (Sigma-Aldrich, Brøndby, Denmark). Then, 100 ml of 4 g/l p-nitrophenyl phosphate (Sigma-Aldrich, Brøndby, Denmark) was added. The reaction was stopped after a 10-min incubation at 37°C, by the addition of 2N NaOH at 100 ml/well. Alkaline phosphatase activity was calculated after measuring the absorbance of the p-nitrophenol product formed, at 405 nm on a micro-plate reader (Bio-Rad, Hercules, CA).
Hepatocyte and pancreatic islet cell growth in scaffolds (in vivo)
Hepatocytes were isolated from livers of Wistar rats by a modified Seglen's method (CitationShen et al. 2012). Isolated hepatocytes were seeded at a final concentration of 15 × 106 cells/ml on a porous TiNi-based SMA scaffold, and cultured at 37оС in a 100% humidified atmosphere of 5% CO2 for 24 h. Then, the scaffolds were implanted into the abdominal cavity of Wistar rats under ketamine anesthesia (1mg/10g dose). The implanted scaffolds were harvested respectively at days 7, 14, 21 and 28 post-implantation.
Islet cells were isolated from the pancreatic glands of Wistar rats by a modified Dexter's method (CitationStull et al. 2012). The isolated islet cells were seeded at a final concentration of 15 × 106 cells/ml on porous TiNi-based SMA scaffolds and cultured at 37°С in 100% humidified atmosphere of 5% CO2 for 24 h. Then, the scaffolds were implanted into the abdominal cavity of Wistar rats under ketamine anesthesia (1mg/10g dose). Implanted scaffolds were harvested respectively at week day 7, 14, 21 and 28 post-implantation.
SEM imaging was performed, with the pre-implanted scaffold at day-0 and harvested scaffolds respectively at day 7, 14, 21 and 28 post-implantation. The scaffolds were washed with phosphate-buffered saline (PBS) and fixed with 2.5% glutaraldehyde solution for an hour, after which the scaffolds were triply flushed with PBS for 15 min and fixed in 1% osmium tetroxide solution (SIGMA) for an hour, followed by triple flushes with PBS for 15 min. Finally, after dehydration by a serial change of ethanol concentration (30, 50, 70, 90 and 100% strength) for 15 min in each solution, each sample was dried. SEM imaging was performed in a Quanta 200 3D SEM (FEI Co. Japan), under a 30 kV operating voltage.
Statistical analysis
The results are expressed as mean ± standard deviation, with n = 10. Error bars in figures represent standard deviations. Differences between experimental groups were analyzed according to a paired nonparametric Mann-Whitney’s U-test, with p < 0.05 considered statistically significant.
Results
The structure of the porous TiNi-based SMA scaffold
The SEM examination indicated that the porous TiNi-based SMA scaffold has interconnected pores () and rough pore walls with a microporous surface (). The implant surface was smooth, with the exception of the cut edge. Actual pore size was determined by measuring the maximum and minimum diameters of a pore on the SEM photos of the surface in several areas of each implant. The measured mean pore sizes of the structure of porous TiNi-based SMA were 323 ± 89мm (n = 00). The mean porosity (evaluated by Hg-porosimetry) was 55.3 ± 6.7% (n = 00).
The mesenchymal stem cell (MSC) growth in scaffolds (in vitro)
Based on weight increase percentages, the osteogenic group showed a 2-fold increase, and the chondrogenic group showed a 1.33-fold increase (), compared to the control group.
Figure 2. The weight increase percentage calculated with the dry weight of cultured scaffold and the initial weight of the scaffold.
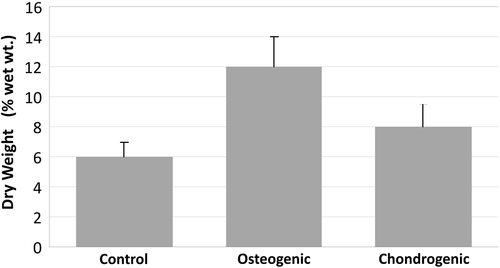
The highest amount of DNA content was detected in the chondrogenic group. Compared to the control group, the chondrogenic group showed a 1.8-fold increase in amount of DNA. Compared to the osteogenic group, the chondrogenic group showed a 5.5-fold increase ().
Based on alkaline phosphatase activity, the highest amount was detected in the osteogenic group. Compared to the control group, the osteogenic group showed a 4.2-fold increase in ALP activity. Compared to the chondrogenic group, the osteogenic group showed a 4.8-fold increase ().
Hepatocyte and pancreatic islet cell growth in scaffolds (in vivo)
In the pre-implanted scaffold at day-0, cells were in the process of active attachment and proliferation. Along the network of interconnected pores, cells were fully spread and fixed on the scaffold walls, which has a lot of small pores measuring less than 3μm in size ().
Figure 5. SEM images showing pancreas islet cells seeded on porous TiNi-based SMA scaffold before implantation: cells fully spread along the network of interconnected pores (a), cells fixed on rough pore walls with micro-porous surface (b).
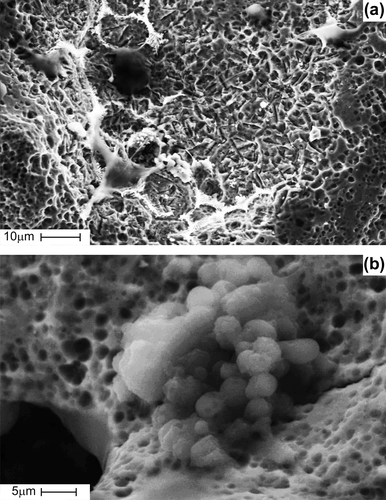
At day-7 post-implantation, cells were in the process of further proliferation, with synthesizing extracellular matrix and forming spatial incrustations of various shapes and sizes (). Cells were observed to spread across the pores. Relatively small pores were fully filled with cells and extracellular matrix ().
Figure 6. SEM images showing time-periodic pancreas islet cell ingrowth seeded on porous TiNi-based SMA scaffold: at day 7 post-implantation, cells in the process of further proliferation with synthesizing extracellular matrix and forming the spatial pseudopodium (a); at day 7 post-implantation, cells spreading across the pores and relatively small pores fully filled with cells and extracellular matrix (b); at day 14 post-implantation gradual cellular ingrowth from the periphery towards the center (c); at day 28 post-implantation, scaffold entirely filled with cells and extracellular matrix (d).
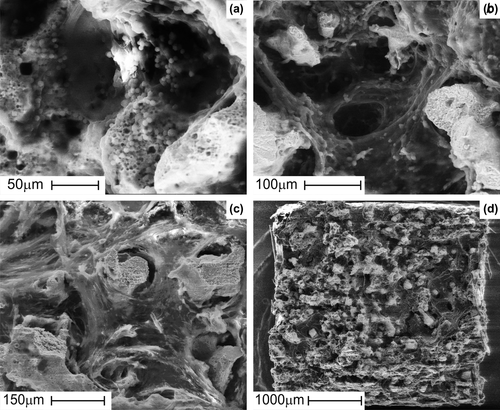
Beginning from day-7 post-implantation, the cellular ingrowth gradually invaded the inner porous structure, from the periphery towards the center. At day-28 post-implantation, all pores were closed and completely filled with cells and extracellular matrix.
Discussion
TiNi-based SMA exhibits unusual mechanical properties which include super-elasticity and shape-memory effects that enable multifunctional application involving high recovery strain, high strength, as well as relatively low Young's modulus (CitationGunther et al. 2014, CitationBansiddhi et al. 2008). Its compressibility and porous structure are beneficial for the in-growth of bone mineralization tissue and fibrous tissue, which makes the fixation of implants much more natural and reliable. The good shape memory effect (SME) and unique volume memory effect can simplify the process of implanting medical implants and reduce the pain suffered by patients (CitationBingyun 1998).
Porous TiNi-based SMA can be fabricated using the self-propagating high-temperature synthesis technique. This advanced technique enables control over porosity and pore size in the porous TiNi-based SMA. This material is a whole porous material with interconnected pores, and retains the unique properties of a solid TiNi-based SMA such as super-elasticity, high damping properties, and good corrosion resistance. Although the fabrication of a porous TiNi-based SMA was somewhat different, the material properties measured have a similar hysteresis pattern in the stress-strain curve. Like other titanium alloys, this material forms a titanium oxide (TiO2) film on the surface, which gives good corrosion resistance. Furthermore, the corrosion resistance can be explained by the fact that Ti is more readily oxidized than Ni (CitationEs-Souni et al. 2005). Although the biocompatibility has been earlier questioned, this alloy has been tested on human osteoblasts, and showed that there is good affinity and biocompatibility between the two (CitationChung et al. 2002). Some of the negative effects mentioned are linked to Nickel. Nickel (Ni) is known to be extremely poor in terms of biocompatibility, showing negative impacts virtually across the board in a range of bioassays. Other studies published, both in vivo and in vitro, show good biocompatibility (CitationEs-Souni et al. 2005).
When combined, pore interconnectivity and super-elasticity give the porous TiNi-based SMA pump-like and capillary properties. Porous TiNi-based SMA can absorb the surrounding fluid and provides a framework where cells can migrate and grow. These properties are ideal and mandatory to promote tissue growth, which are advantages not seen in other porous biomaterials. Porosity also plays a role in decreasing the elastic modulus of the implant, and such an effect minimizes the stress-shielding effect resulting from the elastic modulus mismatch between the bone and implant (CitationAydogmus and Bor 2011).
By manipulating and adjusting the size and ratio of the titanium and nickel powder, the pore size and porosity in a porous TiNi-based SMA implant can be controlled. Pore size and shape are also controllable as these macroporous structures are replicas of polymer sponge templates (CitationLi et al. 2014). The porosity of a material can influence its mechanical properties such as strength, strain and super-elasticity. Porous TiNi-based SMA can be manufactured to closely resemble bone, by controlling the porosity (CitationXiong et al. 2008). TiNi-based alloys also display a mechanical behavior similar to that of bone, showing strain recovery of around 2%, therefore exhibiting better mechanical compatibility compared to other rival porous materials (CitationAydogmus and Bor 2011). The mean pore size for porous TiNi-based SMA used in the study was 323 ± 89мm (n = 00), and the porosity was 55.3 ± 6.7% (n = 00). These dimensions are very similar to those of real bone (CitationGyunter et al. 2000).
Mesenchymal stem cells (MSC) have marked plasticity and potential for multiplication, both of which make them an ideal cell source for musculoskeletal tissue engineering. Mesenchymal stem cells in the limb, which give rise to cartilage and bone in vivo, can be manipulated in vitro. Furthermore, these cells have a lineage progression of separate, individual steps, whether it be the chondrogenic or the osteogenic pathway (CitationCaplan 1991, CitationTuan et al. 2002). Currently, bone marrow aspirate is considered to be the most accessible and enriched source of MSC (CitationTuan et al. 2002).
In the in vitro study with MSCs, both the osteogenic and chondrogenic groups showed significantly higher increase in the dry weight of cultured scaffold than the control group. ALP activity was the most prominent in the osteogenic group, while the DNA content was the highest in the chondrogenic group.
In , the highest weight increase is demonstrated in the osteogenic group. However, in , the highest amount of DNA content was detected in the chondrogenic group. Chondrogenic conditions were associated with enhanced cell proliferation, while other reports indicate that TGF-b stimulated proliferation in other culture systems (CitationMartin et al. 2001). Alternatively, high proliferation may be associated with hypertrophy, a cell behaviour reported for chondrogenic differentiation of MSCs (CitationWinter et al. 2003).
These results indicate that the porous TiNi-based SMA can be used as an incubator for MSCs, for differentiation into both osteogenic and chondrogenic cell lines. Considering the multipotency of MSCs, there is the distinct possibility that the porous TiNi-based SMA is also biocompatible with other cell lineages including the adipogenic, neurogenic, and myogenic cell lineage.
Regarding the in vivo study with hepatocytes and pancreatic islet cells, SEM images confirm that the porous TiNi-based SMA has an optimal porous surface for initial cellular adhesion that mediates all subsequent events such as proliferation, migration, and differentiation. While it was an allogenic cell implantation, consistent cell growth was observed in the scaffolds even if it did not take a long period of time for porous structure of the scaffold to be filled with tissue. It is well known that the life cycle of allogenic cells which were implanted in vivo without scaffold is considerably shortened by the immune responses of the host. Scaffolds allow the cells to create their own extracellular integrating environment, and also act as a temporary barrier to protect them from immune system attack. Therefore, the functional life cycle of cells implanted on scaffolds can be extended, and therapeutic functions of implanted cells can be maintained for much longer.
These findings may lead to the conclusion that the porous TiNi-based SMA is a unique biocompatible incubator for cell cultures, and can be prosperously used for tissue bioengineering and artificial organs. As far as special properties and good biocompatibility are concerned, it is evident that porous TiNi-based SMA has a potential to be a clinical success in several applications in the future.
Acknowledgments
The authors gratefully acknowledge the support for this research provided by the Tomsk State University Competitiveness Improvement Program, Research Institute of Pharmacology (Tomsk, Russia), and Kang & Park Medical Ltd. (Chungcheongbukdo, O-Song, South Korea). Their support is greatly appreciated.
Declaration of interest
The authors report no declarations of interest. The authors alone are responsible for the content and writing of the paper.
Notes
1 biochemical factors – removed
References
- Aydogmus T, Bor A 2011. Production and characterization of porous TiNi shape memory alloys. Turkish J Eng Env Sci. 35:69–82.
- Bansiddhi A, Sargeant TD, Stupp SI, Dunand DC 2008Porous NiTi for bone implants: a review. Acta Biomater. 4:773–782.
- Bingyun LI 1998Microstructure and superelasticity of porous NiTi alloy. Sci China. 42.
- Caplan AI. 1991. Mesenchymal stem cells. J Orthop Res. 9.
- Choinzonov EL, Gunther VE, Muhamedov MR, et al. Medical materials and shape memory implants: In 14 vol. / Shape memory implants in oncology. Tomsk: MIC; 13:336 p.
- Chung DH, Kwon SY, Chung JW, Kim JC, Kang JW, Rhee SK. 2002The biocompatibility of human osteoblasts to porous coated Nickel-Titanium Alloy with thermal shape memory. J Korean Ortho Res Soc. 5.
- Dambaev GTs, Gunther VE, Ziganshin RV, et al. 2012. Medical materials and shape memory implants: In 14 vol. / Shape memory implants in surgery. Tomsk: MIC, 11:398 p.
- Es-Souni M, Es-Souni M, Fischer-Brandies H. 2005 Assessing the biocompatibility of NiTi shape memory alloys used in medical applications. Anal Bioanal Chem. 381:557–567.
- Griffith LG, Naughton G. 2014. Tissue engineering – current challenges and expanding opportunities. Science AAAS. 295:1009.
- Gunther VE, Chekalkin TL, Kim JS, Kim J-S, Hodorenko VN. 2014. Martensite deformation and phenomenon of hysteretic shape change in TiNi-based alloys. Adv Mater Lett. 5:629–633.
- Gunther VE, Dambaev GTs, Sysoliatin PG, Ziganshin R, Kornilov N, Mirgazizov M, et al. 2000. Delay Law and New Class of Materials and Implants in Medicine. Northampton MA: STT,432p.
- Gunther VE, Hodorenko VN, Chekalkin TL, et al. 2011. Medical materials and shape memory implants: In 14 vol. / Shape memory medical materials. Tomsk: MIC, 1. 534 p.
- Gyunter VE, Khodorenko VN, Monogenov AN, Yasenchuk Yu F. 2000. Effect of deformation on the permeability of porous titanium-nickel alloys. Tech Phys Lett. 26:320–322.
- Kang SB, Yoon KS, Kim JS, Nam TH, Gjunter VE. 2002. In vivo result of porous TiNi shape memory alloy: bone response and growth. Mater Trans.43:1045–1048.
- Kim JS, Kang JH, Kang SB, Yoon KS, Kwon YS. 2004. Porous TiNi biomaterial by selfpropagating high-temperature synthesis. Adv Eng Mater. 6.
- Kokorev OV, Hodorenko VN, Anikeev SG, Dambaev GTs, Gunther VE. 2012. Features of using porous-permeable incubators from titanium nickel as carriers of cell cultures of the pancreas. Siberian Med J. 1:130–136.
- Kokorev OV, Hodorenko VN, Anikeev SG, Dambaev GTs, Gunther VE. 2014. Complex research of gepatocytes development in porouspermeable TiNi-based incubators. Bull New Med Technol. 19:32–36.
- Li J, Yang H, Wang H, Ruan J. 2014. Low elastic modulus titanium-nickel scaffolds for bone implants. Mater Sci Eng C. 34:110–4.
- Martin I, Shastri VP, Padera RF, Yang J, Mackay AJ, Langer R, et al. 2001. Selective differentiation of mammalian bone marrow stromal cells cultured on three-dimensional polymer foams. J Biomed Mater Res. 55:229–235.
- Muhamedov M, Kulbakin D, Gunther V, et al. 2014. Sparing surgery with the use of TiNi-based endografts in larynx cancer patients. J Surg Oncol. doi:https://doi.org/10.1002/jso.23779 (in press).
- Murphy CM, O’Brien FJ, 2010, Understanding the effect of mean pore size on cell activity in collagen-glycosaminoglycan scaffolds. Cell Adh Migr. 4:377–381
- Shen L, Hillebrand A, Wang DQH, Liu M. Isolation and primary culture of rat hepatic cells. 2012. J Vis Exp. 64:e3917.
- Stull ND, Breite A, McCarthy R, Tersey SA, Mirmira RG. 2012. Mouse islet of langerhans isolation using a combination of purified collagenase and neutral protease. J Vis Exp. 67:e4137.
- Tuan RS, Boland G, Tuli R. 2002. Adult mesenchymal stem cells and cell-based tissue engineering. Arthritis Res Therapy. 5:32–45.
- Winter A, Breit S, Parsch D, Benz K, Steck E, Hauner H, et al. 2003. Cartilage-like gene expression in differentiated human stem cell spheroids: a comparison of bone marrow-derived and adipose tissue-derived stromal cells. Arthritis Rheum. 48: 418–429.
- Xiong JY, Li YC, Wang HJ, Hodgson PD, Wen CE. 2008. Titanium-nickel shape memory alloy foams for bone tissue engineering. J Mech Behav Biomed Mater. 269–273.