Abstract
This paper describes the formulation of archaeosomes and the evaluation of their abilities to facilitate in vitro DNA delivery. Lipids of the H.hispanica 2TK2 strain were used in archaeosome formation, which is formulated by mixing H.hispanica 2TK2 lipids with plasmid DNA encoding green fluorescent protein (GFP) or β-galactosidase (β-gal). Archaeosome/pDNA formation and unbound DNA were monitored by agarose gel electrophoresis. The archaeosome formulations were visualized by AFM and TEM. The zeta potential analysis showed the archaeosomes to be electronegative. The composition of archaeosomes and the DNA dose for transient transfection into HEK293 cells were optimized, and the relationship between the structure and activity of archaeosomes in DNA delivery was investigated. By themselves, archaeosomes showed low efficiency for DNA delivery, due to their anionic nature. By formulating archaeosomes with a helper molecule, such as DOTAP, CaCl2, or LiCl, the capability of archaeosomes for gene transfection is significantly enhanced. The transfection profiles of efficient archaeosomes are proved to have a long shelf-life when maintained at room temperature. Thus, the archaeal lipids have the potential to be used as transfection reagents in vitro.
Introduction
Members of the Archaea domain are diverse in their adaptation to extreme environments (CitationLuo and Wasserfallen 2001), one of which is a saline environment. Intracellular and extracellular proteins from halophilic archaea face extremely saline conditions, which trigger them to maintain their stability and functionality at nearly saturated salt concentrations. Therefore, haloarchaeal proteins promote specific adaptations for the inhibition of aggregation and loss of activity in extreme conditions (CitationHutcheon et al. 2005). The archaeal lipids are unique among all known living organisms, in the absence of ester-linked glycerol fatty acid lipids, which comprise mainly C20–C40 isoprenoid-subunit backbones, linked by ether bonds to glycerol and/or nonitol bridge group(s) (CitationHanford and Peeples 2002). Due to their unique lipid structure, archaeal species are thought to be challenging, and several efforts have been made to investigate the physiological properties, chemical structure, and genetic background of those species that have ether lipids.
Among the best characterized archaeal representatives, the mechanisms of gene transfer such as transduction, conjugation, and transformation have been discovered (CitationLuo & Wasserfallen, 2001). Advances in the field of genetic engineering have forced scientists to change the genetic material to fight against diseases. The basic aim of gene therapy is to insert healthy copies of missing or defective genes into the cells. The main problem with gene therapy is the delivery of genes. Different transfer methods have been developed to overcome this problem, one of which is the use of liposomes, and these are widely used for non-viral gene delivery. Archaeosomes are liposomes composed of natural lipids found in Archaea, which have the unique structural features of archaeal lipids (CitationDe Rosa et al. 1986, CitationSprott 1992, CitationLi et al. 2011, CitationSprott et al. 2003, CitationHiga et al. 2012). The membrane-spanning properties of archaeal ether lipids provide rigidity of the vesicle membrane, making these archaeosomes more stable and less permeable than conventional liposomes, which frequently need cholesterol to improve their stability (CitationSzoka and Papahadjopoulos 1980, CitationLichtenberg and Barenholz 1988, CitationJacquemet et al. 2009).
It has been demonstrated that archaeosomes have relatively higher levels of stability when exposed to oxidative stress, high temperatures, alkaline pH, and the action of phospholipases, bile salts, and serum media (CitationChoquet et al. 1994, CitationBenvegnu et al. 2005, CitationBrard et al. 2007, CitationPatel and Sprott 1999, CitationPatel et al. 2000, CitationJacquemet et al. 2009). They also uniquely modulate antigen delivery and dendritic cell activation (CitationSprott et al. 2003, CitationSchöll et al. 2005). Archaeosomes possess strong adjuvant properties (CitationKrishnan et al. 2000), and therefore they are proven to be non-toxic, both in vitro and in vivo (CitationPatel and Sprott 1999, CitationOmri et al. 2003). With regard to these properties, archaeosomes can encapsulate various types of compounds and can be stored in the presence of oxygen without any degradation (CitationPatel and Sprott 1999, CitationPatel et al. 1999). The advanced stability and biocompatibility of archaeosomes are advantageous over conventional liposomes (CitationJacquemet et al. 2009). Therefore, there is a great potential for using archaeosomes in applications for drug, vaccine and nucleic acid delivery.
Archaeosomes prepared from the halophilic archaeon Haloarcula hispanica 2TK2 strain mediate functional transfer of the pEGFP-N1 and pSV-β-Gal plasmid DNA to human embryonic kidney (HEK293) cells. In this study, we report that a combination of a helper molecule and archaeosomes results in a potentiation of transfection efficiency in vitro.
Materials and method
Strain and growth conditions
Cells of the Haloarcula hispanica 2TK2 strain was kindly provided by Dr. Birbir (ATCC 33960). The cells were grown aerobically at 40˚C, in a medium containing 18% NaCl, 2% MgSO4·7H2O, 0.5% yeast extract, 0.3% C6H5Na3O7.2H2O, and 0.2% KCl (modified from CitationGrant et al. 2001). All cultures were harvested in the late stationary phase and stored at − 20˚C as cell pastes.
Isolation of lipids
Cell pellets from 50 ml of culture were suspended in 1 ml of 4 M NaCl and extracted with 3.75 ml of methanol/chloroform (2/1, v/v) for 2 h. The extracts were centrifuged, and pellets were re-extracted with 4.75 ml of methanol/chloroform/water (2/1/0.8, v/v/v) (CitationBligh and Dyer 1959). Then, 2.5 ml of chloroform and 2.5 ml of water were added to the combined supernatants. The samples were centrifuged to achieve phase separation. The organic phase containing total lipids was collected. The polar lipid fraction was precipitated by the addition of cold acetone (CitationChoquet et al. 1992). After adding cold acetone, the polar lipids were collected by centrifugation and dissolved in chloroform. The chloroform phase was dried with a rotary evaporator. The polar lipids were dissolved in a small volume of chloroform (1 mg/ml).
Preparation of archaeosomes
Archaeal polar lipids dispensed in chloroform were dried in a round-bottom flask with a rotary evaporator. The resulting lipid films were hydrated with HEPES buffer (pH 7.4), and archaeosomes were obtained by vortexing and extruding (CitationMayer et al. 1986). The Lipofast Basic (Avestin) injection system, which has a polycarbonate filter with a 100 nm pore size, was used for sizing some of the archaeosomes. The sizes and zeta potentials of archaeosomes were analyzed with a Zetasizer Nano ZS (Malvern Instruments). The samples were stored at + 4°C.
Plasmid DNA purification
The pEGFP-N1 (Clontech) and pSV-β-Gal (Promega, USA) plasmids, coding the green fluorescence protein and β-galactosidase reporters respectively, were used to construct archaeosome-DNA formulations. The pDNA was amplified in Escherichia coli DH5α strain grown in LB (+ kan or amp) liquid media, and purified with a Gold XChange Plasmid Midi Kit (Peqlab, Erlangen, Germany). The quality and quantity of the pDNA were spectrophotometrically detected with a PharmaSpec UV-1700 UV-vis spectrophotometer (Shimadzu).
Plasmid DNA and archaeosome formulations
Two different methods were applied for the formulation of pDNA/archaeosome complex formation, and pDNA encapsulation in archaeosomes. The formation of the archaeosome-pDNA complex was achieved by the addition of 1 μg of plasmid DNA to archaeal lipid solution of volumes that typically ranged from 1 to 10 μl, and the mixture was kept at room temperature for 20 min to allow complex formation to take place. To improve the complex formation with pDNA and archaeosomes, some helper molecules such as DOTAP, CaCl2 or LiCl, chosen considering their anionic nature, were also added into the media. For pDNA encapsulation, pDNA was added in the hydration of the lipid film as archaeosomes were prepared. Archaeosomes were obtained by mixing 100–250 μg of pDNA with archaeosome suspension, equivalent to 40 mg of lipid. The effects of encapsulation and complex formation were investigated by agarose gel electrophoresis.
Physicochemical characterization of archaeosomes
Particle size and zeta potential studies
Archaeosomes and pDNA/archaeosomes were prepared as described above, and were diluted 10-fold in a HEPES buffer solution. Particle size was determined using dynamic light scattering [Zetasizer Nano ZS (Malvern Instruments)]. All measurements were conducted at 27°C and in triplicate.
Agarose gel electrophoresis studies
Electrophoresis studies were conducted on 1 w/w agarose gels containing ethidium bromide in 1xTAE buffer, using a standard protocol (CitationSambrook et al. 1989). Briefly, plasmid DNA, archaeosomes, and archaeosome–pDNA complexes prepared as described above, were mixed with DNA-loading buffer [sucrose 40% (w/v), bromophenol blue 0.25% (w/v), and xylene cyanol 0.25% (w/v)], and subjected to agarose gel electrophoresis for 20 min at 100 Volts. The pDNA on the gel was visualized under UV light and digitally photographed.
Transmission electron microscopy studies
The morphological properties of archaeosomes were analyzed with transmission electron microscopy (TEM). Archaeosome samples were prepared by the negative staining method using formvar-coated grids, and investigated with a Jeol T-100 electron microscope at 60 kV (CitationChrystie 1996). Briefly, the archaeosome solution in HEPES buffer (40–50 μl) was deposited on the formvar-coated grids, and stored at room temperature for 10 min for adsorption on the thin film. The film was rehydrated in 1% ammonium molybdate and left to dry at 4˚C. The size and lamellarity of the liposomes were established by TEM.
Atomic force microscopy studies
The archaeosomes were investigated with an atomic force microscope (AFM). Samples were bound to mica-coated grids with MgCl2, and washed twice with distilled water. Plasmid DNA was diluted to a concentration of 4 μg/ml with distilled water. Samples adsorbed on mica were dried at room temperature for 15 min, and investigated with a Shimadzu SPM 9600 (CitationLiang et al. 2005).
Cell culture and transfection of mammalian cells
The biological evaluation of the pDNA and archaeosome formulations obtained by the different methods was assessed by in vitro transfection in HEK293 (Human Embryonic Kidney 293) cells. The cells were cultivated in a DMEM nutrient mixture (Difco, USA) containing 10% (v/v) fetal calf serum (FCS) (Biochrom, UK), and supplemented with 100 U/ml penicillin and 100 μg/ml streptomycin (Sigma, Germany). The cultures were incubated at 37 ˚C under 5% CO2 for 24 h in a humid atmosphere. For pDNA-archaeosome transfection, the cells were seeded into 24-well culture plates (5 × 104 cells per well), and incubated under standard culture conditions for 20–24 h. The culture medium was renewed 30 min before transfection. pDNA (1 μg for each well) diluted in DMEM (-) at a concentration of 20 ng/μl, and 5–20 μl of archaeosome hydrated with HEPES buffer, were mixed and incubated for 20 min at room temperature. The encapsulated pDNA in archaeosomes was directly diluted in DMEM (-) at a concentration of 10 μl/100 μl. The DNA samples were added to the cultures and incubated for 48 h. The medium was replaced 6 h after the transfection, to remove plasmids not internalized by cells. Twenty-four hours post-transfection, the medium was removed, the cells were washed using PBS and lysed in a buffer containing 100 mM KH2PO4/K2HPO4 (pH 7.5), 0.2% Triton X-100, and 1 mM DTT. All transfection experiments were performed in triplicate.
β-gal activity assay
β-galactosidase activity was determined according to CitationTuran and Nagata (2006). In vitro transfection efficiency and β-galactosidase expression were analyzed enzymatically by using ortho-nitrophenyl-β-galactoside (ONPG) (AMRESCO, USA). Briefly, 25 μl of cell lysate was mixed with 295 μl of reaction buffer containing 100 mM of KH2PO4/K2HPO4 (pH 7.5), 10 mM of KCl, 1 mM of MgSO4, and 50 mM of 2-β-mercaptoethanol, and incubated at 37°C for 5 min. Then, 100 μl of ONPG substrate solution (4 mg/ml ONPG in 100 mM phosphate buffer, pH 7.5) was added, followed by a 4-h and 12-h incubation period at 37°C, to allow the enzymatic hydrolysis of ONPG. At the end of the incubation, 180 μl of 1 M Na2CO3 was added to the samples to stop the reaction. The concentration of β-galactosidase was determined by measuring the absorbance at 420 nm with a Shimadzu PharmaSpec UV-1700 spectrophotometer. Two standard graphs were constituted for 4-h and 12-h incubations. β-galactosidase activity was expressed as mU/μg of protein. The total protein was measured with the Bradford protein assay, using bovine serum albumin (BSA) as the standard.
GFP detection by fluorescence microscopy
The expression of the green fluorescence protein in transiently transfected cells was monitored with a fluorescence microscope (Olympus BX50).
Results and discussion
Characterization of archaeosomes
The physical characteristics of the H. hispanica-based archaeosomes were investigated with AFM, TEM, and dynamic light scattering, before sizing with a Lipofast Basic injection system. The AFM and TEM results showed that archaeosomes have small unilamellar spherical shapes ( and ). The AFM images in show that archaeosomes were dispersed and spherical. The sizes of the vesicles were under 400 nm and the mean diameter was approximately 310 nm, which was in close agreement with data obtained by TEM (). Size distribution analysis showed that archaeosomes have a diameter of 350 nm, while archaeosome-pDNA complexes have a diameter of 417 nm and pDNA encapsulated archaeosomes have a diameter of approximately 490 nm. The average particle diameters are shown in , together with the surface potential measurements. The sizes of the vesicles were under 400 nm, and the mean diameter was approximately 350 nm, which was in close agreement with data obtained by AFM and TEM ( and ). These results are in close agreement with those found in the study by CitationGonzález-Paredes et al. (2011), which showed that H. salinarum archaeosomes did not exceed 400 nm in mean diameter and archaeosomes had more irregular membranes than liposomes. Moreover, TEM shows all of the molar ratios at which these vesicles are formed, although their sizes are not very uniform, and they do not aggregate, even after storage for 31 days at 4°C (data not shown). That is in agreement with the findings of CitationGmajner et al. (2011). The reason for the non-aggregation was explained by CitationKates (1993) and CitationTenchov et al. (2006), in extreme halophile archaea.
Table I. Physicochemical characterization of archaeosomes (mean ± SD, n = 3).
The particle size of archaeosomes is 417.8 nm when mixed with pDNA at a pH of 7.74 in PBS at 25˚C, and 490.6 nm when encapsulated with pDNA. Zeta potential analysis showed that all archaeosome formulations are electronegative, as CitationGmajner et al. (2011) observed in the hyperthermophilic archaeon Aeropyrum pernix K1 polar lipids. The average particle size of uncomplexed anionic archaeosomes was 346.1 ± 11.81 nm, whereas those obtained with the addition of higher levels of lipids (20 μg/μl) had a larger average particle size of 353.7 ± 6.75 nm (). The addition of pDNA to the archaeosome suspension produced a change in the particle size, and the average particle size of the archaeosome-pDNA complexes was 417.8 ± 3.22 nm. In formulations containing pDNA-encapsulated archaeosomes, the average particle size was 490.6 ± 7.48 nm. Even though the zeta potential was negative in both formulations tested, it was proved by CitationManosroi et al. (2002) that negatively charged liposomes have higher physical stability and a relatively higher percentage of drug entrapment than positively charged ones.
In vitro transfection of archaeosomes
In order to examine the effect of archaeosome formulations on in vitro transfection, HEK293 cells were transfected with archaeosomes formulated with pSV-β-Gal or pEGFP-N1 plasmid DNA. The transfection efficiencies of total lipids (TL) and polar lipids (PL) of H. hispanica 2TK2 were analyzed at the first step. shows the in vitro transfection results of archaeosomes made of TL or PL of H. hispanica 2TK2. The archaeosomes were mixed with pSV-β-Gal pDNA and transfected into HEK293 cells. β-Gal activities were measured after 48 h. As shown in , β-Galactosidase activity of the PL fraction is about 3 times more than the activity of the TL fraction, which is similar to the results of previous studies. There are multiple studies on the total PL of several bacteria, such as those by CitationChoquet et al. (1992), CitationPatel et al. (1999), CitationBrard et al. (2007), CitationJacquemet et al. (2009), CitationLi et al. (2010), CitationFaisal et al. (2011), and CitationCarrer et al. (2014). Therefore, PL were preferred in relation to the constitution of archaeosomes. However, the transfection efficiency was lower than expected. Accordingly, several helper molecules were utilized, on the basis that they would have a positive effect on the transfection efficiency. The pEGFP-N1 plasmid, which codes the GFP marker protein, was used in some of the transfection assays. The green fluorescent protein synthesized by mammalian cells was viewed under fluorescence microscopy, in order to examine the transfection efficiency in a population of cells after the transfection experiments with the pEGFP-N1 plasmid vector. The HEK293 cells were transfected with pEGFP-N1 (20 ng/μl) at 10 μl/1 μg of archaeosome/pDNA ratio, in the presence or absence of helper molecules. The complete DMEM medium was replaced 6 h after the transfection period, and the cells were incubated in normal growing conditions. GFP expression was visualized at 48 h post-transfection (). Since the archaeosome-pDNA formulations were prepared at a ratio of 10 μl/μg, only a small population of cells was expected to show expression of the GFP transgene. shows a group of cells within the monolayer, which stained positive for archaeosome-pDNA (green). The efficiency of GFP delivery in these experiments was very low, which is in line with the experiments conducted by Zavec et al. (2014) with K14 protein aggregates encapsulated by K1 archaeosomes of Aeropyrum pernix K1.
Figure 3. The transfection of archaeosomes made of total lipids (TL) or polar lipids (PL) of H. hispanica 2TK2 into HEK293 cells grown in 24-well plate. 1 μg pSV-β-Gal pDNA were diluted in DMEM (-) in a concentration of 25 ng/μl per well. pDNA was mixed with related amount of archaeosome given in figure and used in transfection assay. Experiments were performed in triplicate.
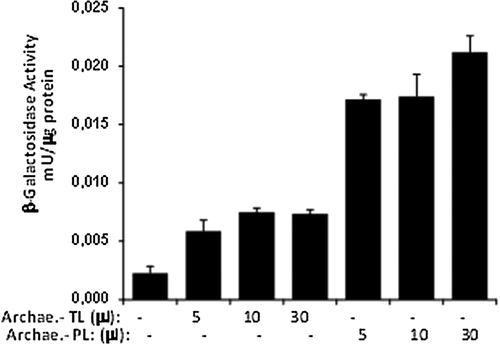
Figure 4. The effects of helper molecules on the transfection of pDNA with archaeosomes. HEK293 cells grown in 24-well plate were used in the experiments. Each well contains 1 μg pEGFP-N1 plasmid DNA diluted in the concentration of 25 ng/μl in DMEM (-), archaeosomes made of polar lipids of H. hispanica 2TK2 (10 μl) and helper molecules. (A) Archaeosome:pDNA (10 μl: 1 μg). (B) 20 mM CaCl2 (C) 50 mM CaCl2 (D) 100 mM CaCl2 (E) pDNA:DOTAP (1 μg: 10 μl) F) Archaeosome:pDNA:DOTAP (10 μl: 1 μg: 10 μl).
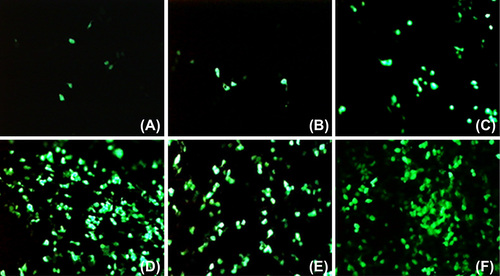
When transfected with complexes incubated in the presence of Ca2+, a much higher proportion of cells expressing GFP could be detected (). As the delivered plasmid resulted in transient expression, the number of cells expressing GFP increased as cells transfected with an increased amount of archaeosomes and CaCl2, like CitationPatil et al. (2004) previously reported. These data demonstrate that the increase in transfection efficiency is due, at least in part, to a higher proportion of cells being transfected when Ca2+ is present in the transfection complex mixtures (CitationLam and Cullis 2000). Also DOTAP, which is a cationic transfection reagent, enhanced in vitro transfection efficiency in the presence of archaeosomes ( and ).
In order to examine the effect of archaeosome formulations on in vitro transfection, HEK293 cells were transfected with pSV-β-Gal DNA and archaeosomes prepared in the presence or absence of helper molecules. The pDNA was complexed with archaeosomes at a lipid to pDNA charge ratio of 10/1 (μl/μg). Increasing concentrations of Ca2+ (10–100 mM) were incubated for 20 min before diluting to the final volume with DMEM culture media, and then applied to cells. Forty-eight hours post-transfection, the cells were harvested in 100 μl of lysis buffer and protein concentrations were detected using the Bradford method. β-galactosidase activities were determined as mU/μg protein, as outlined in ‘methods’. As shown in , a very low level of β-galactosidase activity was detected when cells were transfected with archaeosomes on their own. However, β-galactosidase activities were clearly enhanced in cells that were transfected with complexes incubated in the presence of Ca2+. Similar data was observed with different lipid sources in studies by CitationLam and Cullis (2000) and CitationMozafari et al. (2002). In particular, up to three-fold increases in transgene expression were detected at Ca2+ concentrations between 10 and 100 mM. The β-galactosidase activity was determined by the β-gal activity assay. 1 unit of enzyme is defined as the amount of enzyme hydrolyzing 1 μmol of ONPG into o-nitrophenol and galactose in 1 min at 37°C, at a pH of 7.5.
Figure 5. The effects of CaCl2 (A) and LiCl (B) as helper molecules with archaeosomes on the transfection of pDNA. HEK293 cells grown on 24-well plate were used. 1 μg pSV-β-Gal plasmid DNA was diluted in the concentration of 25 ng/μl per well in DMEM (-) and helper molecules were used in certain amounts indicated on the figure. Experiments were performed in triplicate.
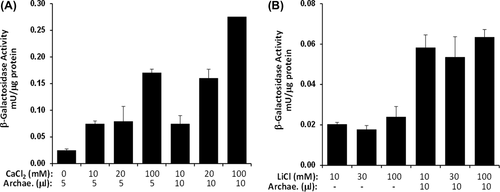
The increased transfection, shown in , in the presence of Ca2 +, could be due to cells expressing a higher level of the delivered gene, or a greater proportion of cells being transfected, or a combination of both. In order to examine the transfection efficiency, the amount of archaeosome was increased, and this enhanced transfection by nearly double. Also, the efficiency of transfection increased as the Ca2 + concentration increased. Enhanced β-gal expression was observed for the archaeosome formulations examined in the presence of helper molecules, while DNA-lipid complexes containing Ca2 + showed the highest transfection efficiency, followed by those with LiCl. The stimulatory effect of LiCl on the transfection properties of plasmid DNA-anionic archaeosomes was also examined (). When the archaeosomes were added to the transfection environment, it was observed that the transfection efficiency increased nearly three-fold in the presence of LiCl.
Conclusions
Easy and economic synthesis, as well as high delivery efficiencies, make the liposomes attractive among non-viral delivery vectors. However, using the archaeosomes alone for nucleic acid delivery was not successfully demonstrated. In this study, we have investigated the potential of archaeal lipids to deliver pDNA into mammalian cells. Here, we formed liposomes from Haloarcula hispanica 2TK2 polar lipids (archaeosomes), and evaluated their potential application as a non-viral gene delivery vectors. The archaeosomes are easy to prepare and highly stable, although anionic liposomes do not show a tendency to create complexes with DNA because of their negative charges. In the scope of the results, the idea of using helper molecules to make cationic archaeosomes arose. Efforts were made to increase the DNA transfection of anionic liposomes into mammalian cells, and the polar haloarchaeal lipids have been used in liposome or archaeosome production in various other studies. In this study, positively charged ions or molecules were used to improve the capacity of archaeosomes to transfer plasmid DNA. We found that the anionic archaeosomes were not effective for gene delivery as stand-alone reagents, while formulating the archaeosomes with DOTAP or Ca2 + showed significant enhancement in DNA delivery in vitro. The effectiveness of this method is not as high as that of commercial products, but it is relatively inexpensive when compared with methods using commercial transfection reagents. Therefore, archaeosomes have the potential to reduce costs if they are used with commercial reagents. Even though we only demonstrated the archaeosome formulation for DNA delivery in this paper, we consider that these formulations would also be useful for the delivery of other nucleic acid-based cargos or therapeutic agents. The design rationales demonstrated in this paper could provide the principles for developing highly efficient new materials for gene delivery and therapy.
Acknowledgements
We thank Prof. Dr. Meral Birbir from the Department of Biology, Marmara University, for providing us H. hispanica 2TK2 strain. The authors wish to commemorate this study to the beloved Prof. Dr. Huriye Kuzu.
Declaration of interest: The authors report no declarations of interest. The authors alone are responsible for the content and writing of the paper.
References
- Benvegnu T, Réthoré G, Brard M, Richter W, Plusquellec D. 2005. Archaeosomes based on novel synthetic tetraether-type lipids for the development of oral delivery systems. Chem Commun. 44:5536–5538.
- Bligh EG, Dyer WJ. 1959. A rapid method of total lipid extraction and purification. Can J Biochem Physiol. 37:911–917.
- Brard M, Laine C, Réthoré G, Laurent I, Lemiègre L, Benvegnu T. 2007. Synthesis of archaeal bipolar lipid analogues: a way to versatile drug/gene delivery systems. J Org Chem. 72:8267–8279.
- Carrer DC, Higa LH, Tesoriero MVD, Morilla MJ, Roncaglia DI, Romero EL. 2014. Structural features of ultradeformable archaeosomes for topical delivery of ovalbumin. Colloids Surf B. 121:281–289.
- Choquet CG, Patel GB, Beveridge TJ, Sprott GD. 1992. Formation of unilamellar liposomes from total polar lipid extracts of methanojens. Appl Environ Microbiol 58:2894–2900.
- Choquet CG, Patel GB, Sprott GD, Beveridge TJ. 1994. Stability of pressure-extruded liposomes made from archaeobacterial ether lipids. Appl Microbiol Biotechnol. 42:375–384.
- Chrystie IL. 1996. Electron microscopy. In: Mahy BWJ, Kangro HO, Eds. Virology Methods Manual London: Academic Press, pp. 91–106.
- De Rosa M, Gambacorta A, Gliozzi A. 1986. Structure, biosynthesis, and physicochemical properties of archaebacterial lipids. Microbiol Rev. 50:70–80.
- Faisal SM, Chen J, McDonough SP, Chang CF, Teng CH, Chang YF. 2011. Immunostimulatory and antigen delivery properties of liposomes made up of total polar lipids from non-pathogenic bacteria leads to efficient induction of both innate and adaptive immune responses. Vaccine. 29:2381–2391.
- Gmajner D, Grabnar PA, Žnidarič MT, Štrus J, Šentjurc M, Ulrih NP. 2011. Structural characterization of liposomes made of diether archaeal lipids and dipalmitoyl-L-α-phosphatidylcholine. Biophys Chem. 158:150–56.
- González-Paredes A, Clarés-Naveros B, Ruiz-Martínez MA, Durbán-Fornieles JJ, Ramos-Cormenzana A, Monteoliva-Sánchez M. 2011. Delivery systems for natural antioxidant compounds: Archaeosomes and archaeosomal hydrogels characterization and release study. Int J Pharm. 421:321–331.
- Grant WD, Kamekura M, McGenity TJ, Ventosa A. 2001. Order I. Halobacteriales In: Garrity GM, Ed. Bergey's Manual of Systematic Bacteriology V.I, The Archaea and Deeply Branching and Phototrophic Bacteria, 2nd ed. New York: Springer.
- Hanford MJ, Peeples TL. 2002. Archaeal tetraether lipids. Appl Biochem Biotech. 97:45–62.
- Higa LH, Schilrreff P, Perez AP, Iriarte MA, Roncaglia DI, Morilla MJ, Romero EL. 2012. Ultradeformable archaeosomes as new topical adjuvants. Nanomedicine. 8:1319–1328.
- Hutcheon GW, Vasisht N, Bolhuis A. 2005. Characterisation of a highly stable α-amylase from the halophilic archaeon Haloarcula hispanica. Extremophiles. 9:487–495.
- Jacquemet A, Barbeau J, Lemiègre L, Benvegnu T. 2009. Archaeal tetraether bipolar lipids: Structures, functions and applications. Biochimie. 91:711–717.
- Kates M. 1993. Membrane lipids of extreme halophiles: biosynthesis, function and evolutionary significance. Experientia. 49:1027–1036.
- Krishnan L, Dicaire CJ, Patel GB, Sprott GD. 2000. Archaeosome vaccine adjuvants induce strong humoral, cell-mediated, and memory responses: comparison to conventional liposomes and alum. Infect Immun. 68:54–63.
- Lam AMI, Cullis PR. 2000. Calcium enhances the transfection potency of plasmid DNA-cationic liposome complexes. Biochim Biophys Acta. 1463:279–290.
- Li Z, Chen J, Sun W, Xu Y. 2010. Investigation of archaeosomes as carriers for oral delivery of peptides. Biochem Biophys Res Commun. 394:412–417.
- Li Z, Zhang L, Sun W, Ding Q, Hou Y, Xu Y. 2011. Archaeosomes with encapsulated antigens for oral vaccine delivery. Vaccine. 29: 5260–5266.
- Liang X, Mao G, Ng KYS. 2005. Effect of chain lengths of PEO–PPO–PEO on small unilamellar liposome morphology and stability: an AFM investigation. J Colloid Interface Sci. 285:360–372.
- Lichtenberg D, Barenholz Y. 1988. Liposomes: preparation, characterization, and preservation. In: Glick D, Ed. Methods of Biochemical Analysis, Volume 33. New York: Wiley, pp. 337–462.
- Luo Y, Wasserfallen A. 2001. Gene transfer systems and their applications in Archaea. Syst Appl Microbiol. 24:15–25.
- Manosroi A, Podjanasoonthon K, Manosroi J. 2002. Development of novel topical tranexamic acid liposome formulations. Int J Pharm. 235:61–70.
- Mayer LD, Hope MJ, Cullis PR. 1986. Vesicles of variable sizes produced by a rapid extrusion procedure. Biochim Biophys Acta. 858:161–168.
- Mozafari MR, Reed CJ, Rostron C, Kocum C, Piskin E. 2002. Formation and characterisation of non-toxic anionic liposomes for delivery of therapeutic agents to the pulmonary airways. Cell Mol Biol Lett. 7:243–244.
- Omri A, Agnew BJ, Patel GB. 2003. Short-term repeated-dose toxicity profile of archaeosomes administered to mice via intravenous and oral routes. Int J Toxicol. 22:9–23.
- Patel GB, Sprott GD. 1999. Archaeobacterial ether lipid liposomes (archaeosomes) as novel vaccine and drug delivery systems. Crit Rev Biotechnol. 19:317–357.
- Patel GB, Agnew BJ, Jarrell HC, Sprott GD. 1999. Stability of liposomes prepared from the total polar lipids of Methanosarcina mazei is affected by the specific salt form of the lipids. J Liposome Res. 9:229–245.
- Patel GB, Agnew BJ, Deschatelets L, Fleming LP, Sprott GD. 2000. In vitro assessment of archaeosome stability for developing oral delivery systems. Int J Pharm. 194:39–49.
- Patil SD, Rhodes DG, Burgess DJ. 2004. Anionic liposomal delivery system for DNA transfection. AAPS J. 6:1–10.
- Sambrook J, Fritsch EF, Maniatis T. 1989. Molecular Cloning: A Laboratory Manual. New York: Cold Spring Harbor Laboratory Press.
- Schöll I, Boltz-Nitulescu G, Jensen-Jarolim E. 2005. Review of novel particulate antigen delivery systems with special focus on treatment of type I allergy. J Control Release. 104:1–27.
- Sprott GD. 1992. Structures of archaebacterial membrane lipids. J Bioenerg Biomembr. 24:555–566.
- Sprott GD, Sad S, Fleming LP, Dicaire CJ, Patel GB, Krishnan L. 2003. Archaeosomes varying in lipid composition differ in receptor- mediated endocytosis and differentially adjuvant immune responses to entrapped protein. Archaea. 1:151–164.
- Szoka F, Papahadjopoulos D. 1980. Comparative properties and methods of preparation of lipid vesicles (liposomes). Annu Rev Biophys Bioeng. 9:467–508.
- Tenchov BG, MacDonald RC, Siegel DP. 2006. Cubic phases in phosphatidylcholine–cholesterol mixtures: cholesterol as membrane fusogen. Biophys J. 91:2508–2516.
- Turan K, Nagata K. 2006. Chitosan-DNA nanoparticles: The effect of cell type and hydrolysis of chitosan on in vitro DNA transfection. Pharm Dev Technol. 11:503–512.
- Zavec AB, Ota A, Zupancic T, Komel R, Ulrih NP, Liovic M. 2006. Archaeosomes can efficiently deliver different types of cargo intoepithelial cells grown in vitro. J Biotechnol. 192: 130–135.