Abstract
In this study presented, p(HEMA) nanoparticles were synthesized by the emulsion polymerization technique and then activated by a silanization agent, 3-aminopropyltriethoxysilane (APTES). The APTES-functionalized p(HEMA) nanoparticles that were synthesized were characterized by studies using the Zetasizer, FTIR and SEM. The p(HEMA)-APTES nanoparticles were further modified with phenyl boronic acid (PBA), and these boronate affinity nanoparticles were used for the recognition of some sugars such as galactose, fructose and raffinose. The system parameters (temperature and initial sugar concentration) were optimized for maximum sugar adsorption. The maximum amount of galactose, fructose, and raffinose adsorbed were found to be 4334.5 mg/g; 4334.9 and 810.0 mg/g, respectively (at 25°C, in a phosphate buffer of pH 7.0). Considering the results of this study, it can be concluded that these nanoparticles may be used as a new alternative for the specific recognition of sugar.
Introduction
It is well known that when the particle size is decreased, the surface area of the adsorbents and their adsorption capacities are increased (CitationÖztürk et al. 2007, CitationSarı et al. 2006, CitationAkgöl et al. 2010). Nanoparticles have been extensively used and developed as new support materials, in order to enhance the adsorption capacity of the adsorbent. Owing to their extremely small size, nanoparticles show unusual physical and chemical properties (CitationEdelstein and Cammarata 1996, CitationLiao and Chen 2002). There are many advantageous behaviors of nanoparticles, vis-à-vis micro or macro meter sized materials, such as minimal diffusional limitations, extremely high surface area per unit of mass of adsorbent, and high adsorption capacity (CitationHegedüs and Nagy 2009, CitationUygun et al. 2010).
Various investigators have extensively studied the interaction of the phenylboronic acid moiety and the molecules carrying the diol group (CitationVan Duin et al. 1984, CitationYamamoto et al. 1998, CitationTong et al. 2001, CitationSpringsteen and Wang 2002, CitationCabell et al. 1999, CitationSpringsteen and Wang 2001). Based on this interaction, polymeric materials have been modified with phenylboronic acid, and they have been used as chromatographic support materials for the separation or purification of the diol group-carrying biomolecules such as sugars, nucleotides, nucleic acids, enzymes, and glycoproteins (CitationMaestas et al. 1980, CitationBouriotis et al. 1981, CitationKoyama and Terauchi 1996, CitationÖzdemir and Tuncel 2000, CitationTuncel and Ozdemir 2000, CitationCamli et al. 2002, CitationElmas et al. 2004, CitationShiino et al. 1995). Phenylboronic acid-functionalized polymeric materials have also been used in studies for the detection of specific biomolecules (CitationGao et al. 2001, CitationAppleton and Gibson 2000, CitationElmas et al. 2007). Boronate affinity systems are one of the affinity techniques which depend on the reversible interaction between boronate and the diol-carrying sugar, and have been used intensively in various biotechnological applications such as saccharide detection, nucleotide adsorption, and glycoprotein and nucleic acid separation. Boronic acid-functionalized polymeric materials also have different kinds of biotechnological applications, for example, as self-healing materials, therapeutic agents, and self-regulated drug delivery systems (CitationCambre and Sumerlin 2011). Boronate affinity chromatography systems have been intensively used for the isolation and/or purification of a large number of biomolecules carrying 1,2- or 1,3- diol groups (CitationPace and Pace 1980, CitationHo et al. 1981, CitationSugumaran and Lipke 1982, CitationGascon et al. 1981, CitationDuncan and Gilham 1975, CitationSinghal 1983, CitationWilliams et al. 1982, CitationMiddle et al. 1983). Specific interaction occurs between the boronic acid group of the polymeric matrix and cis-diol of the target biomolecule, yielding an anionic boronate complex (CitationŞenel 2003). This interaction between the boronic acid-modified polymers and the diol-carrying biomolecules have been investigated, and these boronate affinity systems have been used for the isolation and separation of macromolecules (CitationKataoka et al. 1994, CitationAoki et al. 1996, CitationHisamitsu et al. 1997, CitationAoki et al. 1995, CitationElmas et al. 2004, CitationSteinberg-Tatman et al. 2006).
In the present work, p(HEMA) nanoparticles were synthesized using the emulsion polymerization technique. The p(HEMA) nanoparticles synthesized were activated with 3-aminopropyltriethoxysilane (APTES), and then further functionalized with phenylboronic acid. These boronate affinity polymeric systems were used for the specific adsorption of sugars. For this purpose, the experimental conditions were optimized in order to achieve maximum adsorbed amount of sugars.
Experimental
Materials
2-hydroxyethyl methacrylate (HEMA), ethylene glycol dimethacrylate (EGDMA), poly vinyl alcohol (PVA), phenyl boronic acid (PBA), sugars (galactose, fructose, and raffinose) and 3,5-dinitro salicylic acid (DNS reagent) were purchased from Merck (Darmstadt, Germany). All other chemicals were of analytical grade and were supplied by Sigma Chemical Co. (St. Louis, USA). Water used in the experiment was deionized ultrapure water from the Millipore Simplicity® system (18.2 mΩ cm).
Methods
Synthesis and modification of p(HEMA) nanoparticles
p(HEMA) nanoparticles were synthesized by the surfactant-free emulsion polymerization technique, according to the procedure outlined in the literature, with minor modifications (CitationUygun et al. 2009). Briefly, 0.5 g of PVA was dissolved in 25.0 mL of distilled water, and then mixed with 0.6 mL of HEMA and 0.3 mL of EGDMA. A solution of KPS was prepared by dissolving of 0.0198 g of KPS in 45.0 mL of water, and mixed with the initial polymerization solution. Polymerization was carried out in a water bath at a constant temperature of 70°C for 5 h, with orbital shaking at 100 rpm. At the end of the polymerization process, the synthesized nanoparticles were cleaned by washing with ethanol and water several times, in order to remove the unreacted monomers. A schematic representation of the polymerization process is demonstrated in .
In order to activate the p(HEMA) nanoparticles, APTES was used as a silanization agent. For this, the p(HEMA) nanoparticles synthesized were mixed with 200.0 μL of silane reagent (0.9%), and shaken for 2 h at room temperature. After the silanization process, the nanoparticles were washed five times with 10.0 mL of distilled water and stored at room temperature (CitationSteinberg-Tatman et al. 2006).
Functionalization of the p(HEMA)-APTES nanoparticles with phenyl boronic acid
The boronate affinity agent – phenyl boronic acid – was introduced to the p(HEMA)-APTES nanoparticles by simple coordinate covalent bonding (with the Lewis acid-base reaction) between the nitrogen of APTES and the boron of PBA. Briefly, 200.0 μL of p(HEMA)-APTES nanoparticles were mixed with 5.0 mL of PBA (0.25 mg/mL; in MES buffer of 25 mM and pH 6.0). The solution was stirred for 2 h by an orbital shaker (200 rpm) and centrifuged at 10,000 rpm for 20 min. The supernatant was analyzed spectrophotometrically (268 nm), in order to evaluate the amount of unbound PBA. The schematic representation of the preparation of p(HEMA)-APTES-PBA nanoparticles is shown in .
Characterization studies
The surface morphology and internal structure of the silanized- p(HEMA) nanoparticles were investigated using a scanning electron microscope (SEM) (Philips, XL-30S FEG, the Netherlands). For this purpose, dried samples were covered with a thin layer of gold, and SEM photographs of the nanoparticles were then taken. The FTIR spectra of p(HEMA)-APTES and p(HEMA)-APTES-PBA nanoparticles were obtained with a FTIR spectrophotometer (Shimadzu, FTIR 8000 Series, Japan). For this, dry nanoparticles (about 0.1 g) were thoroughly mixed with KBr (0.1g, IR Grade, Merck, Germany), and pressed into a pellet form, and the FTIR spectrum was then recorded. In order to evaluate the size distribution of the nanoparticles, a Zetasizer was used (Malvern Instruments, 3000 HSA, Malvern Instruments, England).
Sugar recognition studies
The binding of sugars onto the boronic-acid functionalized nanoparticles was carried out via the cyclic borate-ester formation, by the reaction between the boronic acid groups in the tetrahedral anionic form, and the diol groups of sugars (CitationElmas et al. 2004). It is also known that more stable cyclic esters can be produced using boronates that have a tetrahedral structure, and in the present work, the attached PBA was already in the tetrahedral form, in the medium at a pH of 7.0. The adsorption of sugar onto the p(HEMA)-APTES-PBA nanoparticles was studied in a batch system. For this, 100.0 μL of boronate affinity nanoparticles were mixed with sugar solutions and stirred at the rate of 100 rpm at room temperature for 2 h. At the end of this adsorption period, the nanoparticles were separated from the adsorption medium using a centrifuge at 14,000 rpm for 15 min. The supernatant was stored, for the determination of the final sugar concentration. Sugar concentrations in both the initial and final adsorption solutions were determined by the DNS method (CitationAmaya-Delgado et al. 2006) for galactose and fructose, and the phenol sulfuric acid method for raffinose. The amounts of sugars adsorbed were determined according the following equation:
Where Q is the amount of sugar adsorbed on unit mass of the nanoparticle (mg/g); Ci and Ct are the concentrations of the sugar in the initial solution and in the supernatant after adsorption, respectively (mg/ml); V is the volume of the aqueous phase (mL); and m is the mass of the nanoparticles (g).
In order to reach the maximum sugar adsorption, some system parameters were optimized. First of all, the concentration of p(HEMA) in the adsorption medium was optimized. The effect of p(HEMA) concentration on the sugar adsorption was investigated with the concentration range of 0.1–1.0 mg/mL. The amount of boronate affinity agent, PBA, was also varied, between 0.1 and 0.75 mg/mL, and the effect of the PBA concentration on the sugar adsorption was examined. Sugar adsorption efficiency is highly affected by the sugar concentration, and thus adsorption experiments were repeated for various sugar concentrations. For this, the concentrations of galactose and fructose in the medium were varied, between 0.25 and 1.25 mM, while the raffinose concentration was studied with the range of 0.05–0.35 mM. The temperature of the medium is another important factor affecting the adsorption efficiency, and thus sugar adsorption studies were carried out at different temperatures (+ 4–60°C).
Separation of sugars from soy milk medium
Studies on the separation of sugar from soy milk were carried out as an indicator for the usage of boronate affinity nanoparticles in industrial applications. For this, the sugar separation studies were performed with the soy milk concentration ranging from 0.1 to 1.0 mM.
Results and discussion
Synthesis and characterization of p(HEMA) nanoparticles
P(HEMA) nanoparticles were synthesized using the surfactant-free emulsion polymerization technique, activated with a silanization agent– APTES, and then functionalized with PBA. The surface morphology and internal structure of the p(HEMA) nanoparticles were investigated using an electron microscope, and it was easily seen from the SEM pictures that the synthesized particles were spherical in shape and had a rough surface ().
shows the size and size distribution of the particles. As seen in , the average particle size of the synthesized nanoparticles was 163.2 nm, with a polydispersity index of 1.276.
The FTIR spectra of the p(HEMA)-APTES and the p(HEMA)-APTES-PBA nanoparticles are shown in . As seen in the figure, characteristic bands of –OH, C = C and –CH2 of p(HEMA)-APTES were shown at 3500, 1475 and 1465 cm− 1, respectively. Likewise, –OH bands of the p(HEMA)-APTES-PBA were located at 3500 cm− 1, while the bands at 1475 and 1465 cm− 1 belong to the C = C and CH2 bonds, respectively. The peak, at 1725 cm− 1, was relevant to C = C aromatic bonding, and B–O bonds were located at 1347 cm− 1. These findings clearly demonstrate that functional PBA groups were successfully introduced to the nanoparticle structure.
Optimization of sugar recognition conditions
In order to reach the maximum sugar recognition value, the system parameters were changed, and optimum conditions were determined. For this purpose, the effect of the amount of p(HEMA) on the sugar adsorption was investigated first, and the findings have been demonstrated in . As seen in the figure, the amount of galactose and raffinose adsorbed were relatively stable with the increase in the p(HEMA) concentration from 0.1 mg/mL to 1.0 mg/mL. On the contrary, the amount of fructose adsorbed increased first, and then reached a plateau value at higher amounts of p(HEMA). It is also concluded from this figure that the adsorbed amount of galactose and fructose were found to be higher than that of raffinose. This decrease in the adsorption of raffinose can be explained by the steric hindrance of the trisaccharide-structured raffinose molecule.
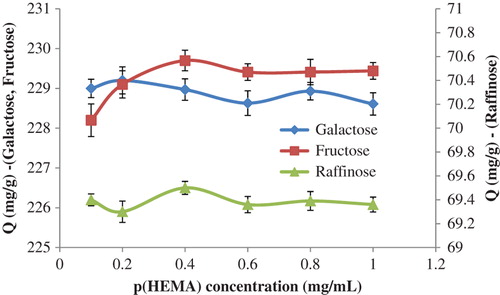
The effects of the amount of PBA on the sugar recognition capacity of the synthesized nanoparticles were also investigated, and the findings have been summarized in . As demonstrated in this figure, the raffinose adsorption capacity of the p(HEMA)-APTES-PBA nanoparticles decreased slightly with the increasing amount of PBA, while the adsorbed amount of galactose increased with the increase in the PBA concentration. Surprisingly, it was found that the fructose adsorption profile had an optimum PBA concentration, and because of this, a PBA concentration of 250.0 μg/mL was used for the further sugar adsorption studies.
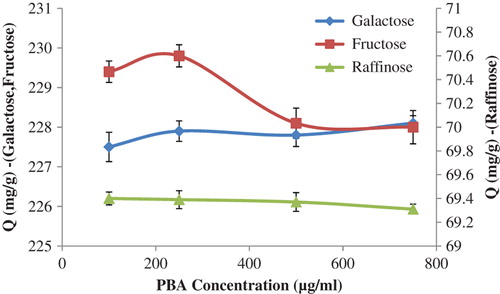
The effects of sugar concentrations on the adsorption of sugar onto boronate affinity nanoparticles were investigated with the concentration of galactose and fructose ranging from 0.25 to 1.25 mg/mL, and the concentration of raffinose ranging from 0.05 to 0.35 mg/mL. shows the effects of sugar concentrations on the sugar adsorption efficiency, and it can be clearly understood from this figure that the amount of sugar adsorbed increased with increasing sugar concentrations.
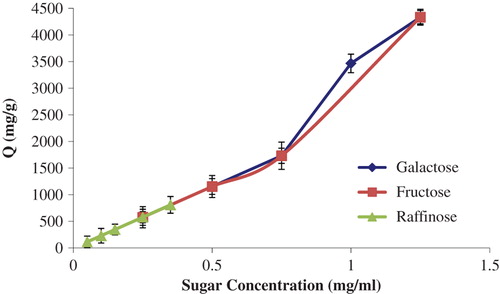
The effect of the temperature of the medium on sugar adsorption was investigated with a temperature range of 4.0°C–55.0°C, and the effect of temperature has been demonstrated in . As seen in the figure, the adsorbed amount of the sugars studied seems to have stayed stable between the temperatures of 4°C and 40°C. However, it was observed that the amount of sugar adsorbed decreased sharply at temperatures higher than 40°C. At low temperatures, boronic acid molecules of the p(HEMA)-APTES-PBA nanoparticles were in a relaxed mode. However, at high temperatures, the boronic acid molecules tended to be together, and were tightened and puckered. The structure of the carbohydrate molecules were also corrupted at high temperatures. These steric hindrances may possibly affect the amount of sugars adsorbed. It is also well known that adsorption is an exothermic process, and therefore the amount of sugars adsorbed decreased with increasing temperature.
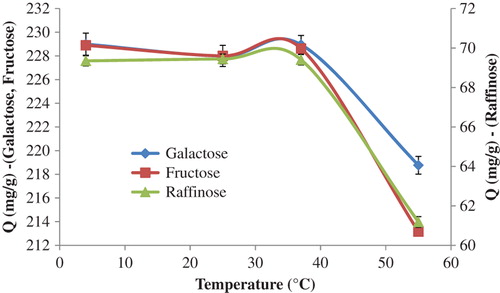
Separation of sugars from the soy milk medium
The profile of sugar separation by the p(HEMA)-APTES-PBA nanoparticles, from the soy milk, is demonstrated in . As shown in the figure, the amount of sugar adsorbed increased with the increase in the concentration of the soy milk. This result led to the conclusion that these boronate affinity nanoparticles may be used successfully for the separation of sugar from the raw soy milk samples.
Conclusion
Boronate affinity systems have been recently used for the recognition and separation of cis-diol-bearing molecules. The purification and recognition of some biological molecules have importance in medical, biotechnological and preparative applications. For these purposes, boronic acid group-bearing affinity supports have been developed and applied. One of the most extensively used support materials are the nanoparticles. These nano-sized particles not only serve to provide a great surface area for the adsorption of biomolecules, but also present unique physical and chemical properties. With these features, nanoparticles can adsorb and recognize a high amount of biomolecules per unit mass. In this study, silanized p(HEMA) nanoparticles were synthesized, functionalized with the boronate affinity ligand – phenylboronic acid, and were used for the recognition of some sugars such as galactose, fructose, and raffinose. It was found from the results of this study that these newly synthesized boronate affinity nanoparticles have good capability for the specific recognition of the sugars analyzed. Based on the advantages of the good sugar-recognition properties, these boronate affinity nanoparticles can potentially be used in biosensor applications for the specific recognition of the sugars. Finally, it can be concluded that these boronate affinity nanoparticles may be used for the recognition of the sugars and sugar-bearing biomolecules, such as nucleic acids and glycoproteins.
Declaration of interest
The authors report no declarations of interest. The authors alone are responsible for the content and writing of the paper.
References
- Akgöl S, Öztürk N, Denizli A. 2010. New generation polymeric nanospheres for lysozyme adsorption. J Appl Polym Sci. 115:1608–1615.
- Amaya-Delgado L, Hidalgo-Lara ME, Montes-Horcasitas MC. 2006. Hydrolysis of sucrose by invertase immobilized on nylon-6 microbeads. Food Chem. 99:299–304.
- Aoki T, Nagao Y, Sanui K, Ogata N, Kikuchi A, Sakurai Y, et al. 1996. Glucose-sensitive lower critical solution temperature changes of copolymers composed of N-isopropylacrylamide and phenylboronic acid moieties. Polym J. 28:371–374.
- Aoki T, Nagao Y, Terada E, Sanui K, Ogata N, Yamada N, et al. 1995. Endothelial cell differentiation into capillary structures by copolymer surfaces with phenylboronic acid groups. J Biomater Sci Polym Ed. 7:539–550.
- Appleton B, Gibson TD. 2000. Detection of total sugar concentration using photoinduced electron transfer materials: development of operationally stable, reusable optical sensors. Sens. Actuators B 65:302–304.
- Bouriotis V, Galpin IJ, Dean PDG. 1981. Applications of immobilised phenylboronic acids as supports for group-specific ligands in the affinity chromatography of enzymes. J Chromatogr. 210:267–278.
- Cabell LA, Monahan MK, Anslyn EV. 1999.A competition assay for determining glucose-6-phosphate concentration with a tris-boronic acid receptor Tetrahedron Lett. 40:7753–7756.
- Cambre JN, Sumerlin BS. 2011. Biomedical applications of boronic acid polymers. Polymer 52:4631–4643.
- Camli ST, Tuncel M, Senel S, Tuncel A. 2002. Nucleotide adsorption–desorption behaviour of boronic acid functionalized uniform- porous particles. J. Chromatogr B. 769:283–295.
- Duncan RE, Gilham PT. 1975. Isolation of transfer RNA isoacceptors by chromatography on dihydroxyboryl-substituted cellulose, polyacrylamide, and glass. Anal Biochem. 66:532–539.
- Edelstein AS, Cammarata RC. 1996. Nanomaterials: Synthesis, Properties and Applications. Institute of Physics Publishing: Bristol, UK.
- Elmas B, Onur MA, Senel S, Tuncel A. 2004. Thermosensitive N- isopropylacrylamide-vinylphenyl boronic acid copolymer latex particles for nucleotide isolation. Colloids Surf A. 232:253–259.
- Elmas B, Senel S, Tuncel A. 2007. A new thermosensitive fluorescent probe for diol sensing: Poly(N-isopropylacrylamide-co-vinylphenylboronic acid)- alizarin red S complex. React Funct Polym. 67:87–96.
- Gao S, Wang W, Wang B. 2001. Building fluorescent sensors for carbohydrates using template-directed polymerizations. Bioorg Chem. 29:308–320.
- Gascon A, Wood T, Chitemerere L. 1981. The separation of isomeric pentose phosphates from each other and the preparation of D-xylulose 5-phosphate and D-ribulose 5-phosphate by column chromatography. Anal Biochem. 118:4–9.
- Hegedüs I, Nagy E. 2009. Improvement of chymotrypsin enzyme stability as single enzyme nanoparticles. Chem Eng Sci. 64:1053–1060.
- Hisamitsu I, Kataoka K, Okano T, Sakurai Y. 1997. Glucose-responsive gel from phenylborate polymer and poly (Vinyl Alcohol): prompt response at physiological pH through the interaction of borate with amino group in the gel. Pharm Res. 14:289–293.
- Ho NWY, Duncan RE, Gilham PT. 1981. Esterification of terminal phosphate groups in nucleic acids with sorbitol and its application to the isolation of terminal polynucleotide fragments. Biochemistry 20:64–67.
- Kataoka K, Miyazaki H, Okano T, Sakurai Y. 1994. Sensitive glucose-induced change of the lower critical solution temperature of poly[N,N-(dimethylacrylamide)-co-3-(acrylamido)-phenylboronic acid] in physiological saline. Macromolecules 27:1061–1062.
- Koyama T, Terauchi K. 1996. Synthesis and application of boronic acid-immobilized porous polymer particles: a novel packing for high-performance liquid affinity chromatography. J Chromatogr B. 679:31–40.
- Liao M-H, Chen D-H. 2002. Fast and efficient adsorption/desorption of protein by a novel magnetic nano-adsorbent. Biotechnol Lett. 24:1913–1917.
- Maestas RR, Prieto JR, Kuehn GD, Hageman JH. 1980. Polyacrylamide-boronate beads saturated with biomolecules: a new general support for affinity chromatography of enzymes. J Chromatogr. 189:225–231.
- Middle FA, Bannister A, Bellingham AJ, Dean PDG. 1983. Separation of glycosylated haemoglobins using immobilized phenylboronic acid. Effect of ligand concentration, column operating conditions, and comparison with ion-exchange and isoelectric-focusing. Biochem J. 209:771–779.
- Özdemir A, Tuncel A. 2000. Boronic acid-functionalized HEMA-based gels for nucleotide adsorption. J Appl Polym Sci. 78:268–277.
- Öztürk N, Tabak A, Akgöl S, Denizli A. 2007. Newly synthesized bentonite–histidine (Bent–His) micro-composite affinity sorbents for IgG adsorption. Colloid Surf A. 301:490–497.
- Pace B, Pace NR. 1980. The chromatography of RNA and oligoribonucleotides on boronate-substituted agarose and polyacrylamide. Anal Biochem. 107:128–135.
- Sarı M, Akgöl S, Karataş M, Denzli A. 2006. Reversible immobilization of catalase by metal chelate affinity interaction on magnetic beads. Ind Eng Chem Res. 45:3036–3043.
- Senel S. 2003. Boronic acid carrying (2-hydroxyethylmethacrylate)-based membranes for isolation of RNA. Colloids Surf A. 219:17–23.
- Shiino D, Murata Y, Kubo A, Kim YJ, Kataoka K, Koyama Y, et al. 1995. Amine containing phenylboronic acid gel for glucose-responsive insulin release under physiological pH. J Control Release. 37:269–276.
- Singhal RP. 1983. High-performance liquid chromatography of transfer RNAs: separation of transfer RNAs from mammalian sources. J Chromatogr. 266:359–383.
- Springsteen G, Wang B. 2002. A detailed examination of boronic acid-diol complexation. Tetrahedron. 58:5291–5300.
- Springsteen G, Wang BH. 2001. Alizarin Red S. As a general optical reporter for studying the binding of boronic acids with carbohydrates. Chem Commun. 17:1608–1609.
- Steinberg-Tatman G, Huynh M, Barker D, Zhao C. 2006. Synthetic modification of silica beads that allows for sequential attachment of two different oligonucleotides. Bioconjug Chem. 17:841–848.
- Sugumaran M, Lipke H. 1982. A procedure for isolation and concentration of catechols by chromatography on dihydroxyboryl cellulose. Anal Biochem. 121:251–256.
- Tong AJ, Yamauchi A, Hayashita T, Zhang ZY, Smith BD, Teramae N. 2001. Boronic acid fluorophore/β-cyclodextrin complex sensors for selective sugar recognition in water. Anal Chem. 73:1530–1536.
- Tuncel A, Ozdemir A. 2000. Thermally reversible VPBANIPAM copolymer gels for nucleotide adsorption. J Biomater Sci-Polym E. 11:817–831.
- Uygun DA, Çorman ME, Öztürk N, Akgöl S, Denizli A. 2010. Poly (hydroxyethyl methacrylate-co-methacryloylamidotryptophane) nanospheres and their utilization as affinity adsorbents for porcine pancreas lipase. Mat Sci Eng C. 30:1285–1290.
- Uygun DA, Karagözler AA, Akgöl S, Denizli A. 2009. Magnetic hydrophobic affinity nanobeads for lysozyme separation. Mat Sci Eng C. 29:2165–2173.
- Van Duin M, Peters JA, Kieboom APG, Van Bekkum H. 1984. Studies on borate esters 1: The ph dependence of the stability of esters of boric acid and borate in aqueous medium as studied by 11B NMR. Tetrahedron. 40:2901–2911.
- Williams GT, Johnstone AP, Dean PDG. 1982. Fractionation of membrane proteins on phenylboronic acid-agarose. Biochem J. 205:167–171.
- Yamamoto M, Takeuchi M, Shinkai S. 1998. Molecular Design of a PET-based chemosensor for uronic acids and sialic acids utilizing a cooperative action of boronic acid and metal chelate. Tetrahedron 54:3125–3140.