Abstract
This work describes the synthesis and macromolecular reactions of maleic anhydride (MA)-acrylamide (AAm) binary and MA-vinyl acetate (VA)- AAm ternary reactive copolymers with γ-aminopropyltriethoxysilane (APTS) as a polyfunctional crosslinker. Swelling parameters such as the start-time of the hydrogel-formation, initial rate of swelling, swelling rate constant, equilibrium swelling, and equilibrium water content (EWC) are determined for polymers/APTS/water systems with certain copolymer/crosslinker ratios (1.4/1 and 9/1). The formation of a hyperbranched network structure by the fragmentation of the side-chain reactive groups in the systems studied has also been confirmed by the Fourier Transform Infrared (FTIR) method.
Introduction
The design and synthesis of novel macromolecular architectures based on hyperbranched polymers and new types of composites including crosslinked networks, hydrogels, and solvent-gel systems are important fields of polymer science and macromolecular engineering (CitationTsukruk 1997, CitationVogl and Jaycox 1999, CitationGao and Yan 2004, CitationNuttelman et al. 2008). There are many different macromolecular structures that are possible for physical and chemical hydrogels. They include the following crosslinked or entangled networks of linear homopolymers, linear copolymers and block or graft copolymers: polyion–multivalent ion, polyion–polyion, or H-bonded complexes; hydrophilic networks stabilized by hydrophobic domains; IPNs or physical blends; and composite hydrogels (CitationHoffman 2002, CitationKim et al. 2007, CitationTibbitt and Anseth 2009, CitationZhang et al. 2011). From this position, highly reactive anhydride-containing macromolecules, including alternating and random copolymers, cyclic copolymers, and block and graft copolymers of maleic anhydride (MA) and its isostructural analogs, can serve as starting materials in achieving the above-mentioned synthesis. The synthesis and macromolecular reactions of anhydride-containing polymers and copolymers with various amines, epoxides, alcohols, polyols, etc. have been described and discussed (CitationCowie 1985, CitationWebster 2003). The copolymerization of AAm with various water-soluble monomers in water or DMSO has been studied (CitationBune et al. 1997, CitationWever et al. 2012, CitationKakuta et al. 2013). Terpolymers of water-soluble acrylamide, acrylic acid, and N-(1,1-dimethyl-3-oxobutyl) acrylamide, were synthesized and characterized (CitationCamail et al. 1998, CitationVermolen et al. 2011). Hyperbranched polymers have a large number of terminal groups that may have some desirable chemical properties – either enhanced solubility in some solvents, or some catalytic property, etc. (CitationKim et al. 2006). Hyperbranched polyesters (HPE) are highly efficient in encapsulating bioactive agents, including drugs, genes, and proteins, due to their globe-like nanostructure (CitationZhang et al. 2011, CitationPillai et al. 2014). The presence of ester groups in the backbone can be used to tune the biological properties and degradability of the scaffolds (CitationZhang et al. 2013). In recent years, hydrogels have attracted a great deal of attention because of their biocompatibility and high water retention. Among different biomaterials, hydrogels are one of the potential candidates, as they can imitate the physical, chemical, electrical, and biological properties of most biological tissues. Owing to these properties, significant progress has been made in designing, synthesizing, and using these materials for tissue engineering, drug delivery, and many biological and biomedical applications (CitationPeppas et al. 2006, CitationHamidi et al. 2008, CitationFisher et al. 2010, CitationKloxin et al. 2010). Improvements in micro- and nanofabrication technologies, and in biomolecular engineering, have been directed at designing composite hydrogel networks with customized functionality (CitationCaldorera-Moore and Peppas 2009, CitationAnnabi et al. 2014).
Silane-based coupling agents, most frequently γ-aminopropyltriethoxysilane (APTS), were used to improve surface adhesion in various polymer composites (CitationMittal 1992, CitationAiroldi and Arakaki 2001) to surface-modify polyethylene films (CitationAkovalı et al. 1996), and for the preparation of silica hybrid materials by an in situ solvent (THF)-gel process using a MA (12%)-styrene random copolymer/tetraethoxysilane/APTS system. Polyimide–silica hybrids were obtained using the non-aqueous solvent-gel process, by polycondensation of phenyltriethoxysilane in a solution of polyamic acid (CitationZhou et al. 1998). Self-catalyzed hydrolysis of phenyl-substituted alkoxysilane and modification of the polyimide structure were applied, and resulted in highly compatible polyimide–silica hybrids. The prepared hybrid films, with a silica content of (45%), had high thermostability. In the APTS-deuterated ethanol-H2O system, the rate of release of ethanol from the ethoxy-groups, a reaction which occurs during the condensation process, was followed by using 1H-NMR spectroscopy at different temperatures (CitationBayer et al. 1999). Changing the hydrophilic-hydrophobic character of the precipitated silica, highly dispersed silica fibers were studied (CitationHsiue et al. 2000, CitationOgasawara et al. 2001, CitationJesionowski and Krysztafkiewicz 2001, CitationXu et al. 2012, CitationFan et al. 2014). Biocomposite hydrogel was treated with silane-based coupling agents, to evaluate the performance properties of treated biocomposite hydrogel. The outcomes of these studies reveal that the treated biocomposite hydrogel was found to possess superior properties compared to the untreated biocomposite hydrogel, with respect to the overall properties of biocomposite hydrogels. We have recently reported that some anhydride-containing copolymers easily undergo crosslinking with APTS in non-aqueous solutions (CitationKaplan Can et al. 2003, CitationRzaev et al. 2001). The synthesis and macromolecular reactions of MA-Methyl methacrylate (MMA) and MA- trans-stilbene (Stb)-n-Butyl methacrylate (BMA) reactive copolymer, and terpolymers with APTS as a polyfunctional crosslinker, were studied (CitationRzaev et al. 2001). One of the macromolecular reactions occurs between the MA and acrylic acid copolymer with APTS (CitationKaplan Can et al. 2003).
In this work, binary and ternary polymerization of MA, AAm, and vinyl acetate (VA) monomer will used with 2,2’-azobisisobutyronitrile (ABIN) as an initiator in benzene, at 70°C, in a nitrogen atmosphere and with an initial monomer feed ratio of 1/1 and 1/2/1. Synthesized polymers will be characterized by FTIR, X-ray diffraction (XRD), elemental analysis, viscosity, acid number, and differential scanning calorimetry (DSC). The results of experiments on the macromolecular reactions of poly(MA-alt-AAm) and poly(VA-co-MA-co-AAm) terpolymer with APTS as a polyfunctional crosslinker are investigated. The characteristics of synthesized hydrogel systems and the swelling parameters in the poly(MA-alt-AAm)/APTS and poly(VA-co-MA-co-AAm)/APTS hydrogels are described and discussed. Swelling parameters, such as the start-time of the hydrogel formation, initial rate of the swelling, swelling rate constant, equilibrium swelling, and equilibrium water content (EWC), are determined for the polymers/APTS/water systems, with certain copolymer/crosslinker ratios.
Experimental
Materials
Initial monomers such as MA, AAm, and VA, were supplied by Fluka, and were distilled before use. They had the following characteristics: MA C4H2O3, b.p. 197–9°C, m.p. 60 (56)oC, MW. 98.06 g/mol.; AAm, C3H5NO, b.p. 87°C (2.7 hPa), m.p. 84°C, d420 = 1.127 g/cm3, MW = 71.08 g/mol. The initiator was 2,2’-azobisisobutyronitrile (AIBN), C8H6N4, b.p.103°C, MW.164.2 g/mol, supplied by Fluka, and was purified by recrystallizing twice from chloroform solution. It was dried under vacuum, and has an m.p. of 106°C. APTS, supplied by Fluka, was used as crosslinker. NH2-CH2CH2CH2-Si (OC2H5)3 was purified before use by distillation under vacuum, and had the following average characteristics: b.p. 217°C (110°C/25.5 mm), d420 = 0.9420 and nD20 = 1.4210. Solvents (Merck) such as p-dioxane, acetone, methyl ethyl ketone, chloroform, benzene, and toluene, as well as methanol, ethanol, and n-hexane were used as precipitating agents (Merck) and were purified before use by a well-known distillation method.
Copolymerization
The syntheses of MA, AAm and VA binary and ternary polymers were carried out in a degassed Pyrex glass, in benzene, with ABIN as an initiator, at 70°C under nitrogen atmosphere. After the given amount of the monomers, initiator, and solvent mixture had been placed into the glass tube and then degassed by three-fold freezing with subsequent melting under vacuum, the reaction system was blown off by purified nitrogen and the tube was sealed and put into a thermostat in a glycerin bath. In order to determine the copolymer yield in the reaction mixture, hydroquinone was added as an inhibitor, and then this mixture was poured into a large amount of methanol to precipitate the copolymers (solution/precipitator = 1/5). The powder-like product obtained was separated by filtration, and then purified by multiple washing in methanol and diethyl ether, and filtered again. The polymers were dried under vacuum at 40°C to a constant weight. All polymers synthesized were powdered in liquid nitrogen, and the powdered product obtained was dried under vacuum at 40°C to a constant weight.
Synthesis of amino-silanized polymer networks
The crosslinking reactions of poly(MA-alt-AAm) and poly(VA-co-MA-co-AAm) synthesized polymers were carried out with APTS as a crosslinker in water at 40°C, 30–40 s before the gel formation of hydrogel. Then, the procedure was carried out in poly(MA-alt-AAm)/APTS and poly(VA-co-MA-co-AAm)/APTS solutions, with various polymer/crosslinker ratios. The process of the formation of network structures was carried out under various thermo treatment conditions at 140°C (15 min, 30 min, and 45 min), and confirmed by FTIR structural analyses of the network polymers formed. For a sample of the polymer hydrogel, the swelling characteristics were also determined. Crosslinking reactions were carried out using the polymer/crosslinker molar ratios of polymer/APTS of : (1.4/1 and 9/1 ().
Table I. Preparation conditions of polymer/crosslinker system (poly(MA-alt-AAm)/APTS) and poly(VA-co-MA-co-AAm)/APTS in water at 40 ± 0.1°C.
Swelling studies
Poly(MA-alt-AAm) and poly(VA-co-MA-co-AAm) gels were obtained in long cylindrical shapes cut into pieces that were 0.5 cm in length. The polymer gels prepared in water or benzene solvent were firstly immersed in water for a week to remove uncrosslinked polymers, and dried to constant weight in vacuum at 40°C, before being used for the swelling experiment. The hydrogels were left to swell in water at 25°C to determine the degree of swelling, and they were then removed from the silicon bath, dried, weighed, and placed in the same bath. The degree of swelling of the crosslinked polymer/APTS crosslinker systems was determined gravimetrically by the following equation:
Where Mo is the dry/initial weight of the polymer gel, and Mt is the weight of the swollen gel at a given time (t) in water.
Measurements
FTIR studies
To analyze the synthesized sample, the FTIR spectra of copolymer films with KBr pellets were recorded with a FTIR Mattson 1000 spectrometer in the 4000–400 cm− 1 range, where 40 scans were taken at a resolution of 16 cm− 1.
XRD studies
The powder diffraction patterns of synthesized samples were recorded using a Philips powder diffractometer. The XRD diffractograms were measured at 2θ, in the range of 2° ≤ 2θ ≤ 50°, using a Cu-Kα incident beam λ = 1.54059 A0), monochromated by a Ni-filter. The scanning speed was 1°/min, and the voltage and current of the X-ray tubes were 40 kV and 30 mA, respectively.
The Bragg equation was used to calculate the interlayer spacing (d) nλ = (2d sinθ), where n is the order of reflection, and θ is the angle of reflection. The crystallinity of the copolymer was calculated using the equation
where s is the magnitude of the reciprocal-lattice vector, which is given by s = (2sinθ)/λ (θ is one-half the angle of deviation of the diffracted rays from the incident X-rays, and λ is the wavelength); I(s) and Ic(s) are the intensities of coherent X-ray scattering from both crystalline and amorphous regions and from only the crystalline region of the polymer sample, respectively,
Where Wc and Wa are the areas of the crystalline and amorphous portions in the X-ray patterns, respectively.
Elemental analysis
The Perkin-Elmer Model 240C Elemental Analyzer was used to determine the C, H, O, and N contents in the polymers synthesized.
DSC Studies
DSC analysis of copolymers was carried out under a nitrogen atmosphere at a heating rate of 10°C/min using a DuPont 910 calorimeter (DuPont, Boston, MA, USA).
The Acid Number
The acid numbers (AN) of the anhydride-containing co- and terpolymers were determined by the non-aqueous titration method, using the following equation:
where V1 is the total content of KOH before addition (ml); N1 is normality of KOH (0.0909 N KOH); V2 is content of HCl required to titrate the KOH abundance (ml); N2 is the normality of HCl (0.096 N HCI); and m is the content of the polymer sample (g). Thus, the AN is the number of milligrams of KOH required to neutralize 1 g of polymer sample.
Viscosity studies
Intrinsic viscosities of the synthesized polymers were also determined in water at 25 ± 0.1°C and at concentrations ranging from 0.1–1.0 g/dL using an Ubbelohde viscometer. The values of [η] were determined from the plot of ηsp/c vs c concentration of polymers, using the following equation:
where ηsp is known as specific viscosity. Non-interaction of the polymer coils requires infinite dilution and this is achieved mathematically by defining a quantity called the intrinsic viscosity, [η], according to the equation
Results and discussion
Macromolecular Crosslinking Reactions of Poly (MA-alt-AAm) and Poly (VA-co-MA-co-AAm) with APTS
Anhydride-containing reactive functional groups are vitally important in polymer science. Preparation of gels and thermosetting systems of polymers containing epoxy, isocyanate, and amine and carboxylic acid groups are widely used commercially in applications such as protective coatings, adhesives, and composites (CitationWebster 2003). Two of the important intra- and intermolecular structural characteristics of polyfunctional polymers are the structural regularity and the bond flexibly of their macromolecules. When the polar polymers possess H-bonding capability, the most energetically favored crystal structures will tend to capitalize on these features. The new functional copolymers synthesized can be used as reactive materials for the synthesis of new materials and network structures, with a given composition and specific properties (CitationKaplan Can et al. 2003). APTS attaches proteins, DNA, and drug molecules to glass surfaces, which can be achieved by derivatizing and coating the surface with a silane containing an amino group. Once the amine is available, numerous crosslinking agents can be used to immobilize proteins, DNA, or other molecules to the surface of microplates, glass cover slips, silica supports, pipettes, and other surfaces. Surface modification by a silanization reaction is used in numerous fields of bio nanotechnology, especially in the field of biomaterials, where cellular adhesion and proliferation often need to be promoted for better biocompatibility (CitationSong et al. 2012, CitationZhang et al. 2012, CitationMoreno et al. 2014).
In this part of the study, the results of experiments on the macromolecular reaction of poly(MA-alt-AAm) and poly(VA-co-MA-co-AAm) polymers with APTS as a polyfunctional crosslinker were given. The identifying characterization of the crosslinking mechanism and the swelling process of the hydrogels in water also were exhibited.
The copolymers and terpolymers synthesized using the given concentration and molar ratios of the initial monomers were found to have the following characteristics:
Poly(MA - AAm) Poly(MA-alt-AAm)
[Benzene]/[M]total = 2.5, [AIBN] = 2.1.10 − 3 mol/L, reaction temperature 70 ± 0.1°C, reaction time 4 h, Yield 85%, intrinsic viscosity [η] = 0.21 dL/g in water at 25°C, percent crystallinity 9.8% (by XRD analysis), AN = 296 mg KOH/g, Tg = 137.8°C and Tm = 403.0°C (by DSC analysis), monomer unit ratio in copolymer by elemental analysis (m1/m2:) = 58.3/41.7
FTIR spectra (KBr pellet), cm− 1: 3500–3200 (–NH2 in the AAM unit and –OH in the MA unit), 3610, 3200 and 2178 (–OH stretching in –COOH), 2980 (vas –CH in –CH3), 2942 (vas CH in CH2), 2920 (vs –CH in –CH2), 2875 (vas CH in CH3), 2860 (vs –CH in –CH2), 1863 (vas C = O) and 1783 (vs C = O) for anhydride unit 2950–2800 (stretch of CH in the AAm unit), 1845 –C = O of the anhydride group), 1715–1610 (–C = O of the acid and amide groups), 1500 (bending bands of –CH in the MA group), 1350 (C–O of the acid group), 1250–1200 (–CN in the AAm unit), 1100 (deformation bands of –CH in the AAm unit), 940 (acid –OH group in MA).
Poly(VA - MA - AAm), Poly(VA-co-MA-co-AAm)
[Benzene]/[M]total = 2.5, [AIBN] = 2.1 × 10− 3 mol/L, reaction temperature 70 ± 0.1°C, reaction time 4 h, Yield 21%, intrinsic viscosity [η] = 0.26 dL/g in water at 25°C, crystallinity 8.9% (by XRD analysis), AN = 340 mg KOH/g, Tg = 141.0°C and Tm = 404.0°C (by DSC analysis), monomer unit ratio in terpolymer by elemental analysis (m1/m2/m3) = 24.8/43.8/31.2
FTIR spectra (KBr pellet), cm− 1: 3610, 3200 and 2178 (–OH stretching in –COOH), 2980 (vas –CH in –CH3), 2942 (vas –CH in–CH2), 2920 (vs –CH in –CH2), 2875 (vas –CH in –CH3), 2860 (vs –CH in –CH2), 1863 (vas –C = O) and 1783 (vs –C = O) for the anhydride unit, 1743 (–C = O), 1634 (–C = O) for –COOH group, 1470 (scissor vibrations of –CH2), 1430 (δasCH3 antisymmetric deformation), 1220–1180 (stretching of anhydride C‐O‐C), 1210–1200 (δCH3), 1100 (broad stretching of C‐O‐C in VA), 620–595 (δCH in CH‐CH anhydride unit), 3500–3200 (–NH2 in the AAm unit and –OH in the MA unit), 2950–2800 (stretch of CH in the AAm unit), 1845(–C = O of the anhydride group), 1715–1610 (–C = O of the acid and amide groups), 1500 (bending bands of CH in the MA group), 1350 (C–O of the acid group), 1250–1200 (–CN in the AAm unit), 1100 (deformation bands of –CH in AAm unit), 1030 (deformation bands of –CH in the VA unit), 940 (acid –OH group in MA).
For the studies of the crosslinking and swelling processes, poly(MA-alt-AAm) copolymer (50/50) with monomer unit composition m1/m2 = 58.3/41.7, and poly(VA-co-MA-co-AAm), are used as cross-linkable polymers. APTS contains amine and triethoxysilyl reactive groups which are used for their polyfunctional crosslinker property. From the structural peculiarities of these polymer/crosslinker systems and the classic principles of macromolecular reactions, it can be assumed that the hyperbranched network structure in these systems will be formed by the intermolecular reactions between the anhydride unit and the amine group, as well as between the ethoxysilyl and free carboxyl fragments.
The general scheme of crosslinking reactions of anhydride-containing copolymers can be represented in the following form (). It is shown that the gel formation process in poly(MA-alt-AAm)/APTS systems starts after heating at 40°C with intensive mixing of these polymer/crosslinker solutions in water for a certain period of time. represents the preparation conditions of polymer/crosslinker systems in water.
The start-time of gel-formation (Gt), in the system studied depends on the type of polymer, polymer/crosslinker ratio, temperature, and other factors. After the start of gel formation, a dramatic increase of viscosity in the polymer/crosslinker solution and in the formation of hydrogel is observed.
To elucidate some peculiarities of the gel formation process in poly(MA-alt-AAm)/APTS and poly(VA-co-MA-co-AAm)/APTS crosslinker systems, swelling parameters such as the start-time of hydrogel formation (Gt), equilibrium swelling, initial swelling rate (ri), rate constant (ks), maximum swelling equilibrium Smax, and EWC are determined by using known methods which are described in the experimental section (CitationKaplan Can et al. 2000a,Citationb). A fundamental relationship exists between the swelling of a crosslinked polymer in a solvent, and the nature of the polymer and the solvent. Swelling of the three-dimensional network structure in a suitable solvent is the most important parameter for swelling measurements (CitationKaplan Can et al. 2003).
The kinetic curves of swelling in the poly(MA-alt-AAm)APTS and poly(VA-co-MA-co-AAm)/APTS systems studied are illustrated and . The swelling capacities of poly(MA-alt-AAm)/APTS gels increase with time, but after a certain period, they show constant swelling, and the swelling process changes to an equilibrium state (CitationHorkay and Zirinyi 1988).

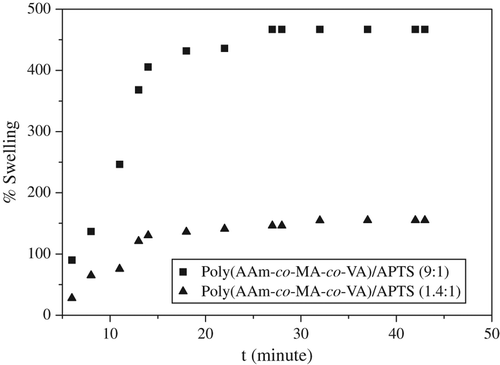
The increase of APTS concentration in the gel systems can cause a decrease in the swelling behavior for copolymers and terpolymers. For the terpolymer/APTS system, the percentage of swelling is less than that of the copolymer/APTS system. This behavior can be explained by the VA structure in the terpolymer system.
For extensive swelling of poly(MA-alt-AAm)/APTS and poly(VA-co-MA-co-AAm)/APTS hydrogels, the following equation (CitationMencer and Gomzi 1994, CitationKaplan and Güner 2000a) can be written:
where B = 1/(Seq), the reciprocal of the maximum swelling Seq (g water/g gel) is A = 1/(kASeq2), ri is the initial swelling rate (g water/g gel)/min of the gel, and ks is the swelling rate constant (g gel/g water)/min. This relation represents second order kinetics ( and ). The results obtained are given in .
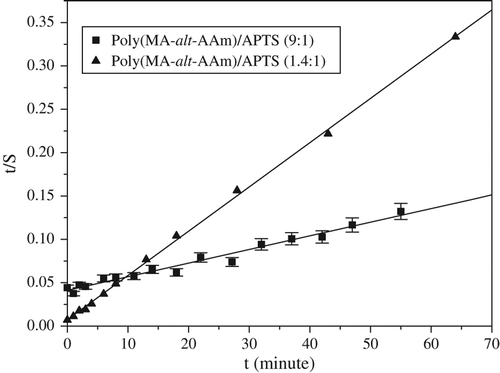
Figure 4. t/S vs. t curves of poly(VA-co-MA-alt-co-AA)/APTS systems using various ratios of terpolymer/crosslinker.
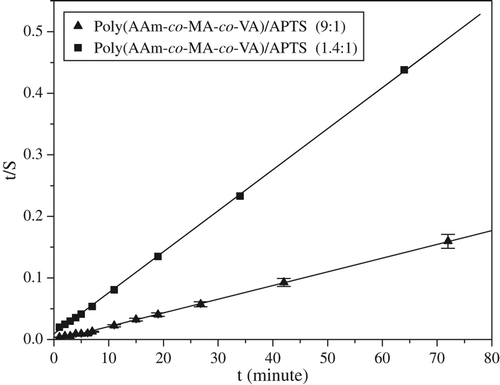
Table II. Swelling parameters of the various polymer/APTS gel systems.
The values of theoretical equilibrium swelling of the hydrogels are in good agreement with the results of equilibrium swelling of poly(MA-alt-AAm)/APTS and poly(VA-co-MA-co-AAm)/APTS hydrogels ( and ). It is well known that the swelling phenomena are directly related to the structure of the crosslinked polymer and/or the density of gel.
The value for EWC is calculated from the following equation (CitationFrisch 1980, CitationKaplan and Güner 2000b)
Where Weq is the water content diffused into the gel at equilibrium state and Wdry is the weight of initially dried gel; the determined percent EWC (% EWC) value is presented in . EWC, as well as the swelling behavior of the hydrogel, mainly depend on the nature of the network in the network structure, that is, hydrophilicity, crosslink density, and the average molecular weight between two consecutive crosslinks. The results obtained allow to simply reflect on the possibility of the network structure formation in poly(MA-alt-AAm)/APTS and poly(VA-co-MA-co-AAm)/APTS hydrogel systems (CitationKaplan and Güner 2000a).
According to all the swelling parameters of the two hydrogel systems, we find that poly(MA-alt-AAm)/APTS depicts higher swelling behavior than the poly(VA-co-MA-co-AAm)/APTS hydrogel system. This can be explained by the free hydrolyzed anhydride-COOH group of the copolymer, which can cause high swelling and EWC.
FTIR studies of crosslinking reactions
The formation of network structures and the structural changes are also confirmed by FTIR studies for poly(MA-alt-AAm)/APTS and poly(VA-co-MA-co-AAm)/APTS systems. The thin polymers and KBr pellet are prepared for the polymer/APTS mixture (9/1 and 1.4/1) and undergo thermo-treatment at 140°C for 15, 30, 45, and 60 min. Then, FTIR spectra of the copolymer/APTS and terpolymer/APTS are recorded ( and ). The analysis of FTIR spectra reveals the following structural changes of macromolecules in consequence to the intermolecular reactions between the functional groups of the copolymer and terpolymer and APTS (CitationKaplan Can et al. 2003).
Figure 5. FTIR spectra of poly(MA-alt-AAm)/APTS gel systems with different molar ratios of copolymer (a), and copolymer/APTS = 9/1 (b), 1.4/1 (c).
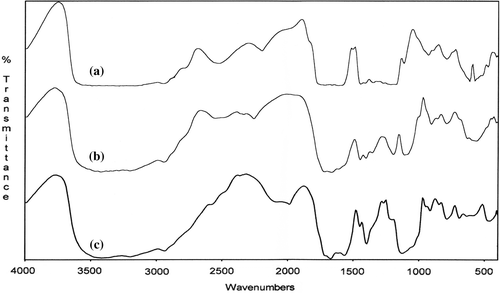
Figure 6. FTIR spectra of poly(VA-co-MA-alt-co-AA)/APTS gel systems with different molar ratios of terpolymer (a) and terpolymer/APTS = 9/1 (b), 1.4/1 (c).
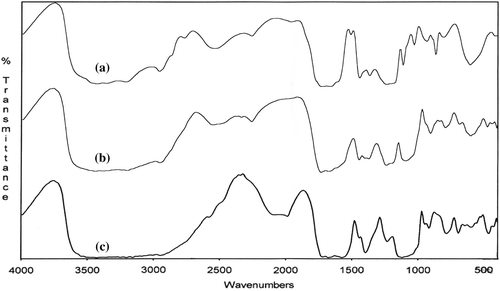
(1) | Appearance of the anhydride unit bands at 1850 and 1750 cm–1 (ν C = O) (Spectra b and c, and ). | ||||
(2) | Disappearance of the peak of –COOH at 3500 cm–1 of because of intra –H bonding between MA –C = O groups (Spectra b and c, and ). | ||||
(3) | Appearance of a new broad peak at 3500–2500 cm–1 because of interaction between the (NH–) amine groups of APTS and the –COOH of MA groups (Spectra b and c, and ). | ||||
(4) | Appearance of a new –NH deformation band at 1570 cm–1 (for amide group) which is shifted, and the deformation in the shape of the peak. Another observation can be explained by the initial amidization reaction between the anhydride unit (–C = O) and –NH group of the APTS, as shown in the scheme ( and ). | ||||
(5) | The intensity of bands appreciably increases by thermo treatment, this effect is more appreciable (Spectra b, c, d and e, and ). With respect to the full transformation of the anhydride unit to the amide form in the case of a gel coating system containing a relatively high concentration of APTS these changes observed can be explained by the initial amidization reaction of the anhydride unit as shown in the scheme. | ||||
(6) | The appearance of new N–H bands at 1580–1640 cm–1 (for amide group) in the form of a doublet, due to the known interaction between symmetric stretching and bending vibrations in the –O = C–NH– group; the intensity of these bands increases with thermo treatment ( and ). A new band at 1640 cm–1 is related to the antisymmetric stretching vibration of the amide band; it is a known fact that even in the case of interaction between MA copolymers and the same drugs with a free amine group, the reaction proceeds via amidization of the anhydride group, and the FTIR analysis indicates the formation of an amide band. | ||||
(7) | The presence of the bands at 1610 cm–1 and 1485 cm–1, arises from the stretching vibrations of the –C = O groups and the carboxylate ion groups (–C = O in –COOH, –COO −, NH3+– and –COOSi(OEt)n fragments); the reaction of the anhydride cycle with the primary and carbonyl group in the salt form (–COOH….NH2–) is a known fact; furthermore, the unchanged deformation in the bands between 1400–1635 cm–1 represent impacts of the silicon carboxylate fragments formed in the network structure; the Si–O–Si stretching bands appear in the field of 1029–1120 cm–1 and 850 cm–1,which also confirms the formation of a crosslinked network in the systems studied, by the fragmentation side-chain ethoxysilyl groups initiating with free carboxyl groups, as shown in the scheme. | ||||
(8) | and also show the crosslinking reaction completed. There is no spectral change that can be seen from the spectra e and f. This means that the macromolecular reaction between the water-soluble anhydride-containing copolymer and the crosslinking agent (APTS) cannot change after 45 min (CitationKaplan Can et al. 2003). |
Conclusions
Given the composition, poly(VA-co-MA-co-AAm) and poly(MA-alt-AAm) were synthesized by radical binary copolymerization with 2,2’-ABIN as an initiator in benzene, at 70°C, in a nitrogen atmosphere and with an initial monomer feed ratio of 1/1 and 1/2/1. The synthesis and macromolecular reactions of MA– AAm binary and MA– VA- AAm ternary reactive copolymers with APTS were achieved. The synthesized polymers were characterized by FTIR, XRD, elemental analysis, viscosity, acid number, and DSC. The swelling parameters, such as the start-time of the hydrogel formation, the initial rate of swelling, the swelling rate constant, equilibrium swelling, and the EWC were determined for the polymer/APTS/water systems with certain copolymer/crosslinker ratios. The formation of a hyperbranched network structure through the fragmentation of side–chain reactive groups and crosslinking mechanisms in the systems studied was also highlighted by the FTIR method. Anhydride-based hydrogel systems are promising as potential biotechnological materials for many applications.
Declaration of interest
The authors report no declarations of interest. The authors alone are responsible for the content and writing of the paper.
References
- Airoldi C. Arakaki LNH. 2001. Immobilization of ethylenesulfide on silica surface through sol–gel process and some thermodynamic data of divalent cation interactions. Polyhedron. 20:929–936.
- Akovalı G, Rzaev ZMO, Mamedov DG. 1996. Plasma surface modification of polyethylene with organosilicon and organotin monomers. Eur Polym J. 32:373–383.
- Annabi N, Tamayol A, Uquillas JA, Akbari M, Bertassoni LE, Cha C, et al. 2014. 25th anniversary article: Rational design and applications of hydrogels in regenerative medicine. Adv Mater. 26:85–124.
- Bayer T, Eichhorn KJ, Grundke K, Jacobasch HJ. 1999. FTIR spectroscopic studies of interfacial reactions between amino functionalized silicon surfaces and molten maleic anhydride copolymers. Macromol Chem Phys. 200:852–857.
- Bune YV, Barabanavo AJ, Bogachev YS, Gromov VF. 1997. Copolymerization of Acrylamide with various water-soluble monomers. Eur Polym J. 33:1313–1323.
- Caldorera-Moore M, Peppas NA. 2009. Micro- and nanotechnologies for intelligent and responsive biomaterial-based medical systems. Adv Drug Deliv Rev. 61:1391–1401.
- Camail M, Essaoudi H, Gedoux B, Margaillan A, Vernet, JL. 1998. Terpolymeâ rısatıon radıcalaıre de L’acrylamıde, de l’acıde acrylıque et de L’acrylamide de n-(1,1-dımeâ thyl-3-oxobutyle). Eur Polym J. 34:1007–1012.
- Cowie JMG. 1985. Alternating Copolymers. New York: Plenum Press.
- Fan Y, Labreche Y, Lively RP, Jones CW, Koros WJ. 2014. Dynamic CO2 adsorption performance of internally cooled silica-supported poly(ethylenimine) hollow fiber sorbents. AIChE J. 60:3878–3887.
- Fisher OZ, Khademhosseini A, Langer R, Peppas NA. 2010. Bioinspired materials for controlling stem cell fate. Acc Chem Res. 43:419–428.
- Frisch HL. 1980. Sorption and transport in glassy polymers-A review. Polym Eng Sci. 20:2–13.
- Gao C, Yan D. 2004. Hyperbranched polymers: from synthesis to applications. Prog Polym Sci. 29:183–275.
- Hamidi M, Azadi A, Rafiei P. 2008. Hydrogel nanoparticles in drug delivery. Adv Drug Deliv Rev. 60:1638–1649.
- Hoffman AS. 2002. Hydrogels for biomedical applications. Adv Drug Deliv Rev. 54(1):3–12.
- Horkay F, Zirinyi M. 1988. Studies on mechanical and swelling behavior of polymer networks on the basis of the scaling concept. 7. Effect of deformation on the swelling equilibrium concentration of gels. Macromolecules. 21:3260–3266.
- Hsiue GH, Chen JK, Liu YL. 2000. Synthesis and characterization of nanocomposite of polyimide–silica hybrid from nonaqueous sol–gel process. J Appl Polym Sci. 76:1609–1618.
- Jesionowski T, Krysztafkiewicz A. 2001. Influence of silane coupling agents on surface properties of precipitated silicas. Appl Surf Sci. 172:18–32.
- Kakuta T, Takashima Y, Nakahata M, Otsubo M, Yamaguchi H, Harada A. 2013. Preorganized hydrogel: Self-healing properties of supramolecular hydrogels formed by polymerization of host–guest-monomers that contain cyclodextrins and hydrophobic guest groups. Adv Mater. 25:2849–2853.
- Kaplan H, Güner A. 2000a. Characterization and determination of swelling and diffusion characteristics of poly(N-vinyl-2-pyrrolidone) hydrogels in water. J Appl Polym Sci. 78:994–1000.
- Kaplan H, Güner A. 2000b. Swelling behavior of poly(N-vinyl-2-pyrrolidone) and poly(N-vinyl-2-pyrrolidone)/K2S2O8 hydrogels in urea solutions. Adv Polym Tech. 3:210–217.
- Kaplan Can H, Rzaev ZMO, Güner A. 2003. Synthesis and characterization of new hydrogels on the basis of water-soluble maleic anhydride copolymers with γ-aminopropyltriethoxysilane. J Appl Polym Sci. 90:4009–4015.
- Kim BS, Im JS, Baek ST, Lee JO, Sigeta M, Yoshinaga K. 2006. Synthesis of polyglycidol hydrogel films crosslinked with carboxyl-terminated poly(ethylene glycol). Polym J. 38:335–342.
- Kim BS, Im JS, Baek ST, Lee JO, Azuma Y, Yoshinaga K. 2007. Synthesis and characterization of crosslinked hyperbranched polyglycidol hydrogel films. J Macromol Sci Pure Appl Chem. 43:829–839.
- Kloxin AM, Kloxin CJ, Bowman CN, Anseth KS. 2010. Mechanical properties of cellularly responsive hydrogels and their experimental determination. Adv Mater. 22:3484–3494.
- Mencer HJ, Gomzi Z. 1994. Swelling kinetics of polymer-solvent systems. Eur Polym J. 30:33–36.
- Mittal KL. 1992. Silanes and Other Coupling Agents. The Netherlands: VSB BV.
- Nuttelman CR, Rice MA, Rydholm AE, Salinas CN, Shah DN, Anseth KS. 2008. Macromolecular monomers for the synthesis of hydrogel niches and their application in cell encapsulation and tissue engineering. Prog Polym Sci. 33:167–179.
- Moreno E, Schwartz J, Larraneta E, Nguewa PA, Sanmartín C, Agüeros M, et al. 2014. Thermosensitive hydrogels of poly(methyl vinyl ether-co-maleic anhydride) – Pluronic® F127 copolymers for controlled protein release. Int J Pharm. 459:1–9.
- Ogasawara T, Yoshino A, Okabayashi H, O’Connor C. 2001. Polymerization process of the silane coupling agent 3-aminopropyltriethoxy silane–1H NMR spectra and kinetics of ethanol release. J Colloid Surf A. 180:317–322.
- Peppas NA, Hilt JZ, Khademhosseini AT, Langer R. 2006. Hydrogels in biology and medicine: From molecular principles to bionanotechnology. Adv Mater. 18:1345–1360.
- Pillai JJ, Thulasidasan AKT, Anto RJ, Chithralekha DN, Narayanan A, Kumar GSV. 2014. Folic acid conjugated cross-linked acrylic polymer (FA-CLAP) hydrogel for site specific delivery of hydrophobic drugs to cancer cells. J Nanobiotechnology. 12:2–9.
- Rzaev ZMO, Güner A, Kaplan Can H, Asıcı A. 2001. Reactions of some anhydride-containing copolymers with γ-aminopropyltriethoxysilane. Polymer. 42:5599–5606.
- Song A, Rane AA, Christman KL. 2012. Antibacterial and cell-adhesive polypeptide and poly(ethylene glycol) hydrogel as a potential scaffold for wound healing. Acta Biomater. 8:41–50
- Tibbitt MW, Anseth KS. 2009. Hydrogels as extracellular matrix mimics for 3D cell culture. Biotechnol Bioeng. 103:655–663.
- Tsukruk VV. 1997. Assembly of supramolecular polymers in ultrathin films. Prog Polym Sci. 22:247–311.
- Vermolen ECM, Van Haasterecht MJT, Masalmeh SK, Faber MJ, Boersma DM, Gruenenfelder MA. 2011. Pushing the envelope for polymer flooding towards high-temperature and high salinity reservoirs with polyacrylamide based terpolymers. SPE Middle East oil and Gas Show and Conference, 25–26 september Manama-Bahrain.
- Vogl O, Jaycox GD. 1999. ‘Trends in Polymer Science’ Polymer Science in the 21st century. Prog Polym Sci. 24:3–6 .
- Webster DC. 2003. Cyclic carbonate functional polymers and their applications. Prog Org Coating. 47:77–86.
- Wever DAZ, Raffa P, Picchioni F, Broekhuis AA. 2012. Acrylamide homopolymers and acrylamide− N-isopropylacrylamide block copolymers by atomic transfer radical polymerization in water. Macromolecules. 45:4040–4045.
- Xu L, Feng J, Li J, Liu X, Jiang S. 2012. Graphene oxide bonded fused-silica fiber for solid-phase microextraction-gas chromatography of polycyclic aromatic hydrocarbons in water. J Sep Sci. 35:93–100.
- Zhang H, Patel A, Gaharwar AK, Mihaila SM, Iviglia G, Mukundan S, et al. 2013. Hyperbranched polyester hydrogels with controlled drug release and cell adhesion properties. Biomacromolecules. 14:1299–1310.
- Zhang J, Liang Y, Li N, Li X, Hu R, Xing J, et al. 2012. Thermosensitive hydrogel based on poly(ether–ester anhydride) nanoparticle as drug delivery system: Preparation, characterization and biocompatibility. Colloids Surf B. 96:56–61.
- Zhang N, Li Ruqiang R, Zhang L, Chen H, Wang W, Liu Y, et al. 2011. Actuator materials based on graphene oxide/polyacrylamide composite hydrogels prepared by in situ polymerization. Soft Matter. 7:7231–7239.
- Zhou WJ, Dong HK, Qui Y, Wei YJ. 1998. Preparation and properties of poly(styrene-co-maleic anhydride)/silica hybrid materials by the in situ sol–gel process. Polym Sci Chem A. 36:1607–1613.