Abstract
A biosensor was developed using glutathione peroxidase (GSH-Px) as the enzyme. Firstly, platinum (Pt) nanoparticles were deposited onto a glassy carbon paste electrode (GCPE), and then GSH-Px was immobilized by means of gelatin that was then crosslinked with glutaraldehyde. The measurement was based on the electrochemical oxidation of GSH to its disulfide form in the presence of hydrogen peroxide. The linear range was found to be between 10 and 250 μM, with a correlation coefficient of R2 = 0.9968. The R.S.D value for 25 μM GSH (n = 6) was calculated as 2.92%. Finally, the proposed biosensor was used to analyze GSH in a synthetically prepared plasma sample, and a promising recovery value was obtained.
Introduction
The altered levels of the thiols (reduced forms) and their disulfides (oxidized forms) in physiologic liquids have been linked to specific pathologic conditions and are closely associated with several human diseases, especially premature arteriosclerosis, occlusive vascular and neurodegenerative disorders, leukemia, diabetes, and acquired immunodeficiency syndrome (AIDS) (CitationLiu et al. 2010, CitationÇubukçu et al. 2012).
As a kind of abundant low-molecular mass thiol found in mammals, reduced GSH is the major non-protein thiol in living cells, with cellular concentrations ranging from 0.5 to 10 mmol L− 1, that detoxifies reactive oxygen and drug metabolites (Çubukçu et al., CitationChen et al. 2006, CitationHou et al. 2009). GSH-Px and glutathione reductase (GR) are the main enzymes that participate in the GSH metabolism. They have substantial functions. For example, GSH-Px plays an essential role in the removal of hydrogen peroxide (H2O2) and lipid peroxides from the cells, while the GR regulates the ratio of reduced and oxidized glutathione in erythrocytes (CitationRover et al. 2001). GSH-Px belongs to the family of selenoproteins (CitationLehman et al. 2001, CitationScheller et al. 2002) containing selenocysteine in the catalytic center (CitationRover et al. 2001, CitationScheller et al. 2002), and is the most extensively studied selenoprotein (CitationLehman et al. 2001, 9).
According to Lehmann et al., (CitationLehmann et al. 1998) the catalytic reaction involves the reduced enzyme, which reacts chemically as a selenolate (Ez–Se−) with the H2O2, leading to an activated enzyme (Eq. (1)). Since the oxidized enzyme needs to be reduced to maintain its activity, it may be performed by an electron donor species like GSH, as shown in the scheme:
Where (Ez–Se)I and (Ez–Se)II are the enzyme-oxidized intermediates, GSH is a reducing substrate, and GS• is a free radical (CitationRover et al. 2001). All GSH-Px take part in the hydroperoxide metabolism and oxidative defense system, which reduce hydroperoxides like H2O2, organic hydroperoxides, and fatty acid hydroperoxides with the consumption of GSH, following the equation below (CitationLehman et al. 2001, CitationScheller et al. 2002):
Several methods, including Ellman's method (CitationEllman 1959), high-performance liquid chromatography (CitationShi et al. 1999), spectrofluorimetry (CitationChen et al. 1999), spectrophotometry (CitationRaggi et al. 1991), and potentiometry (CitationCompagnone et al. 1991) have been reported in the literature for GSH analysis.
Enzymatic methods based on GSH-Px (CitationRover et al. 2001, Lehman et al. 1998) immobilized onto an electrode surface (Carsol et al. 1998) or in-column reactors (CitationCompagnone et al. 1994) have also been utilized for the determination of GSH. The enzyme catalyzes the oxidation of GSH to the disulfide form (GSSG), in the presence of hydroperoxides (H2O2 or ROOH), as shown below (CitationRover et al. 2001):
Because of its high sensitivity, voltammetric methods (CitationKinoshita et al. 1999) have been applied extensively for GSH detection in recent years. However, there are some disadvantages that need to be overcome for the direct electrochemical oxidation of GSH at unmodified traditional electrodes. These can be described as the slow electron transfer reaction of GSH, which requires high working potential, and the strong adsorption of GSH at the surface of the metallic electrode, which makes GSH detection harder. These problems have been solved using modified electrodes which were prepared by suitable modifiers, including nanoparticles (NPs) (CitationLiu et al. 2010, CitationÇubukçu et al. 2012).
Xian et al. (CitationXian et al. 2005) proposed a nanocomposite which was prepared by the electrodeposition of Pt microparticles onto the MWCNTs matrix. They modified the surface of the glassy carbon electrode (GCE) with this kind of nanocomposite, and obtained Pt-modified GCE, which was used for measurement of thiols such as L-cysteine and GSH. As a result, they discovered that the nanocomposite-modified electrode exhibited high sensitivity and good stability for the detection of thiols.
In recent years, carbon electrodes have been widely used in electrochemistry, because of low background current, wide potential, chemical inertness, low cost, and suitability for several sensing and biosensing applications, together with the fact that they provide fast response and can easily be fabricated in different configurations and sizes. There have been so many uses of carbon electrode forms in electroanalytical applications, such as GCE, carbon paste electrode (CPE), the rigid carbon-polymer composite-based electrode, graphite epoxy composite electrode, and a composite electrode material based on mixing glassy carbon (GC) micro particles with an oil binder, the glassy carbon paste electrode (GCPE) (CitationAnik Kirgoz et al. 2005, CitationAnik and Cubukcu 2008, CitationAnik and Cevik 2009, CitationAnik et al. 2010a). GCPEs have high electrochemical reactivity, a wide potential window with a low background current, and they are inexpensive, easily prepared, modified, and renewed (CitationAnik et al. 2010a, CitationAnik and Cevik 2009, CitationAnik et al. 2010b). Besides, it was observed that this electrode has better electrochemical reactivity towards the oxidation of hydrogen peroxide (H2O2) compared to conventional CPE (CitationCubukcu et al. 2007).
In the present work, the electrochemical behavior of GSH was investigated using Pt-nanoparticle (Pt-NP)-modified GCPE. Firstly Pt-NP was electrodeposited on the surface of bare GCPE, and then the GSH-Px enzyme was immobilized onto the electrode surface by means of a gelatin membrane, which was then cross-linked by glutaraldehyde, as shown in .
The biosensor response obtained to GSH was monitored by following the oxidation of GSH in the presence of H2O2. After the optimization of experimental parameters, analytical characteristics were investigated, and then the system developed was applied for GSH detection in synthetic plasma solution.
Experimental
Instruments
Differential pulse voltammetric measurements were carried out with the FRA 2 μ-AUTOLAB Type III electrochemical measurement system from ECO CHEMIE Instruments B.V. (the Netherlands, www.ecochemie.nl), driven by GPES software. The experiments were conducted in a 10 mL voltammetric cell, at room temperature (25°C), using a three-electrode configuration. A Pt electrode served as an auxiliary electrode, while an Ag/AgCl electrode served as a reference electrode, and Pt-NP-modified GCPE immobilized with GSH-Px was used as the working electrode. The auxiliary and reference electrodes were inserted into the cell through the Delrin cover from the top, while the working electrode was placed at the bottom of the cell.
Reagents
Carbon glassy spherical powder 2–12 micron, 99.95% metal basis, and mineral oil were purchased form Aldrich. Chloroplatinic acid hexahydrate was obtained from Sigma-Aldrich. KCl solution (max. 0.0001% Al, puriss, from Riedel-de Haën) was used as a background solution for electrochemical deposition of Pt-NPs. GSH-Px (Glutathione-Peroxidase, fetal bovine erythrocyte lyophilized powder, white, ∼100 units.mg− 1) from Sigma was used for biosensor application. Stock solutions of 0.01 M GSH and H2O2 were freshly prepared by dissolving the appropriate amount of GSH (γ-Glutathione reduced, L-γ-Glutamyl-glycine GSH, cell culture-tested, Sigma) and H2O2 (35%, from Merck). Phosphate buffer solution (KH2PO4, Merck/purity 99–99.5%, 0.05 M, pH 7.5) served as a supporting electrolyte.
Synthetic plasma samples were prepared by incorporating the reagents into the TRIS-HCl, including 140 mM NaCl (99.0–100.1%, supplied from Pancreac), 4.5 mM KCl, 2.5 mM CaCl2 (Calcium chloride dehydrate, ACS reagent, ≥ 99%, from Sigma), 0.8 mM MgCl2 (magnesium chloride hexahydrate, GR for analysis 99.0–101.0%, obtained from Merck), 2.5 mM urea (99.5% pure, used from Horasan Chemistry), and 4.7 mM glucose (D(+) glucose monohydrate for microbiology, from Merck). We used 10 mM TRIS-HCl buffer (Tris(hydroxymethyl)-aminomethane, TRIS, 99.0–100.1%, supplied from Merck) as the background for the synthetic plasma solution. For the interference study, ascorbic acid (L-ascorbic acid, reagent grade) and L-cysteine (≥ 98.5% (RT)) were used, supplied from Sigma. All solutions were prepared using double-distilled water, and the other chemicals were of analytical grade.
Electrode preparation
First of all, bare GCPE was prepared with a ratio of 80/20 (w/w %) glassy carbon spherical powder/mineral oil, by hand mixing, using a spatula. A portion of the resulting paste was then packed firmly into the electrode cavity (3.0 mm diameter and 5.0 mm depth) of a Delrin tube, where electrical contact was established via a copper wire. The surface of the resulting paste electrode was smoothed and rinsed carefully with double-distilled water. Pt-NPs were accumulated onto the GCPE by means of an electrochemical process. For this purpose, cyclic voltammetry was applied between − 0.70 V and + 0.80 V at 50 mV·s− 1 for 50 cycles, where 0.1 M KCl containing 1.0 mM H2PtCl6 solution was used as the deposition solution (CitationLiu et al. 2010). Finally, a well-dispersed yellow-colored layer, indicating Pt-NP, was observed on the surface of the electrode ().
The GSH-Px immobilization procedure was then applied according to the following process: 0.2 mg of GSH-Px was diluted in 1 mL of phosphate buffer solution (pH 7.0). Then, 5 U of GSH-Px and gelatin (3 mg) were mixed at 38°C in potassium phosphate buffer (pH 7.0, 50 μL). Next, 50 μL of the mixed solution was spread over the Pt-NP-modified GCPE surface, and allowed to dry at 4°C for 1 h. Finally, it was immersed in 2.5% glutaraldehyde in phosphate buffer (50 mM, pH 7.0), for 5 min, for cross-linkage ().
Procedure
Prior to its use, the modified composite electrode was stirred in a phosphate buffer solution (pH 7.5) for a while, to remove excess glutaraldehyde solution from the electrode surface. Firstly, the right amount of GSH and H2O2 were put into the working cell, and the mixture was deaerated for a further 5 min by passing N2 gas through the solution. The cell was placed in the voltammetric stand, and the reference and counter electrodes were immersed into the solution. Differential pulse voltammograms were recorded in the range between 0 V and + 0.7 V in 50.0 mM phosphate buffer (pH 7.5) medium. The electrode was stirred for 15 min. before the beginning of each measurement.
Sample application
The biosensor obtained was used for the GSH analysis in a synthetically prepared plasma sample, to show the applicability of the method for clinical analysis. These samples were analyzed using the standard addition method. The synthetic plasma sample was prepared according to the procedure reported earlier (CitationColdur et al. 2010). Synthetic plasma solution comprised of 140 mM NaCl, 4.5 mM KCl, 2.5 mM CaCl2, 0.8 mM MgCl2, 2.5 mM urea, and 4.7 mM glucose, and 10 mM TRIS-HCl buffer was used as the background for the synthetic plasma solution. The final pH of the solution was adjusted to 7.3, with the addition of the right amount of 1 M HCl. The standard solutions were prepared by using the synthetic plasma electrolyte as a background solution, and then the phosphate buffer solution was spiked with the proper amount of analytes (pH 7.5).
Results and discussion
Electrodeposition of Pt-NPs by cyclic voltammetry
Pt-NPs were electrodeposited onto the bare GCPE using cyclic voltammetry, between 0.70 V and + 0.80 V at 50 mV·s− 1, in a solution of 0.1 M KCl containing 1.0 mM H2PtCl6. demonstrates the cyclic voltammogram obtained for the deposition of Pt-NPs after 50 successive scans. It was observed that the current increases with an increase in the scanning number, indicating electropolymerization. The two additional redox steps obtained at − 0.356 V and − 0.424 V corresponded to PtCl62−/PtCl42− (I) and PtCl42−/Pt0 (II), respectively. (CitationWand and Lin 2005)
The effect of the presence of Pt-NPs on electrode structure
shows the differential pulse voltammograms for the electrochemical oxidation of GSH, in the presence of H2O2 in phosphate buffer solution (pH 7.0), with GSH-Px(immob)/GCPE () and Pt-NP-modified GSH-Px(immob)/GCPE (). As can clearly be seen from the figure, no anodic peak was observed for GSH at GSH-Px(immob)/GCPE (), while a well-defined anodic peak appeared when the Pt-NP was incorporated onto the electrode surface (). This result demonstrates the electrocatalytic effect of Pt-NP on current response.
The EIS characterization of the GSH biosensor
The Nyquist plot (Zim vs Zre) is one of the typical electrochemical impedance spectra (EIS) which include a semicircular region lying on the Zre axis, observed at higher frequencies related with the electron-transfer-limited process, and then followed by a linear part at lower frequencies, representing the diffusion-limited electron transfer process. The semicircle's diameter equals the electron-transfer resistance, Ret, which is controlled by the surface modification of the electrode (CitationYang et al. 2008). Accordingly, shows the EIS of (a) bare GCPE, (b) GSH-Px-immobilized GCPE, (c) GSH-Px(immob)/Pt-np/GCPE for 1 mM [Fe(CN)6]3−/4− redox couple.
Figure 4. Nyquist plots of the response of the biosensing electrode at different stages in the electrode assembly process: bare GCPE (X); GSH-Px(immob)/GCPE (■) and GSH-Px(immob)/Pt-NP/GCPE (▲). All spectra were recorded in the presence of 1 mM [Fe(CN)6]3−/4− in 0.05 M PBS (pH 7.5), as a redox-active indicator. Frequency range: 104 to 0.01 Hz, 0.05 V amplitude.
![Figure 4. Nyquist plots of the response of the biosensing electrode at different stages in the electrode assembly process: bare GCPE (X); GSH-Px(immob)/GCPE (■) and GSH-Px(immob)/Pt-NP/GCPE (▲). All spectra were recorded in the presence of 1 mM [Fe(CN)6]3−/4− in 0.05 M PBS (pH 7.5), as a redox-active indicator. Frequency range: 104 to 0.01 Hz, 0.05 V amplitude.](/cms/asset/1c983d5e-f168-434e-9617-59ef109ac0ef/ianb_a_1008504_f0004_oc.jpg)
From the spectra obtained, it can be said that bare GCPE shows a response corresponding to the electron-transfer-limited process, while GSH-Px(immob)/GCPE exhibits a tendency towards an increase of the semicircle related with the electron-transfer-limited process. This finding indicates the immobilization of GSH-Px via gelatin onto the electrode surface, the degradation of the interfacial electron transfer between the electrode and redox indicator in solution, and the isolation of conductive support. The electron transfer via redox couple is hindered by the presence of enzymes on the electrode surface. The increased Ret value of GSH-Px(immob)/GCPE is due to the immobilization of enzymes onto the GSH-Px(immob)/GCPE surface. Most biological molecules, together with enzymes, depending on the enhancement of Ret, are poor electrical conductors at low frequencies (at least < 10 kHz), and obstruct the electron transfer (Chaujan et al. 2012).
When the Pt-NP was incorporated onto the GSH-Px(immob)/GCPE, a decrease was observed in Ret. Thus, there was a conducting Pt-NP on the electrode surface, and this shows us that the system tends towards a diffusion-limited electron transfer process from an electron-transfer-limited process, relatively.
Optimization of experimental parameters
Effect of enzyme amount
In order to investigate the optimal amount of enzyme, differential pulse voltammetric responses of GSH were recorded with various amounts of GSH-Px (). The effect of enzyme amount (1, 2, 5, 10 and 15 units) on current values obtained was investigated for 100 μM GSH/50 μM H2O2, and shown in . As can be seen from the figure, current values increased depending on the amount of enzyme, for up to 5 units, and then a sharp decrease was observed for higher amounts of enzyme, which can be attributed to a problem in diffusion (CitationAnik et al. 2010a). Here, the excess amount of protein might limit the efficient substrate-enzyme interaction that is required for this reaction. Since the best results were obtained at 5 units, further experiments were conducted with this amount.
Figure 5. Differential pulse voltammetric responses of 100 μM GSH/50 μM H2O2 on the biosensor response at various amounts of enzyme: (a) 1.0 U, (b) 2.0 U, (c) 5.0 U, (d) 10.0 U, and (e) 15.0 U. Conditions: phosphate buffer solution system (50 mM, pH 7.0), potential range of 0 V and + 0.7 V. Inset: the curve showing the effect of optimum enzyme amount of GSH-Px(immob)/Pt-NP/GCPE.
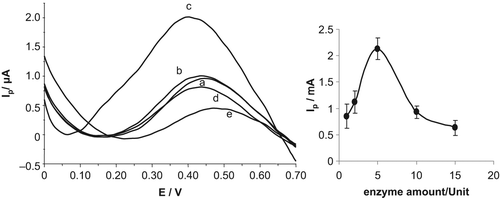
Effect of GSH/H2O2 ratio
According to the enzymatic mechanism proposed for the enzyme GSH-Px, an enzyme pre-activation with peroxide was needed. It is also reported that in the absence of peroxide, no amperometric response was obtained based on the GSH electrooxidation (CitationRover et al. 2001, CitationSatto et al. 1999). On the other hand, the peroxide concentration must be maintained at consistent levels, since at high peroxide concentrations, an enzymatic inactivation can occur during the regeneration of oxidized enzyme with GSH (CitationRover et al. 2001, CitationSatto et al. 1999). As a result, it is important to obtain the optimum peroxide amount for this reaction. For this purpose, the effect of the amount of H2O2 on the assay response was evaluated by differential pulse voltammetry, by varying the proportion between GSH and peroxide, as 0.1/1, 0.5/1, 1/1, 2/1, 5/1 and 10/1. At proportions higher than 2/1, a stabilization of the signal was observed; while for ratios lower than 1/1, the response obtained was considerably lower (). As a result, the proportion of 2/1 was chosen as the optimum value and used for further studies.
Figure 6. Differential pulse voltammetric responses of different GSH/H2O2 ratios on the biosensor response; (a) 0.1/1 GSH/H2O2, (b) 0.5/1 GSH/H2O2, (c) 1/1 GSH/H2O2, (d) 2/1 GSH/H2O2, (e) 5/1 GSH/H2O2, and (f) 10/1 GSH/H2O2. Conditions: phosphate buffer solution system (50 mM, pH 7.0), [GSH] = 50.0 μM; potential range of 0 V and + 0.7 V, T = 25°C. Inset: Effect of the GSH/H2O2 ratio in the performance of the GSH biosensor.
![Figure 6. Differential pulse voltammetric responses of different GSH/H2O2 ratios on the biosensor response; (a) 0.1/1 GSH/H2O2, (b) 0.5/1 GSH/H2O2, (c) 1/1 GSH/H2O2, (d) 2/1 GSH/H2O2, (e) 5/1 GSH/H2O2, and (f) 10/1 GSH/H2O2. Conditions: phosphate buffer solution system (50 mM, pH 7.0), [GSH] = 50.0 μM; potential range of 0 V and + 0.7 V, T = 25°C. Inset: Effect of the GSH/H2O2 ratio in the performance of the GSH biosensor.](/cms/asset/aee449d1-f9ab-4d83-b549-d563b02e0729/ianb_a_1008504_f0006_b.gif)
Effect of pH
The electrochemical behavior of GSH was studied in 50 mM phosphate buffer solution with various values of pH ranging from 5.0–8.0. The results obtained show that for pH values < 7.5, the response decreases. This may be due to the lower activity of the enzyme in this media (CitationRover et al. 2001). However, for pH values higher than 8.0, there is no significant amplification in the signal, presumably due to the reduced GSH being unstable in alkaline solution, as it is easily oxidized. In addition, there is a probability that protein denaturation might occur in alkaline media (CitationRover et al. 2001). Therefore, the pH of 7.5 was selected for further applications (data not shown).
Analytical characteristics
After optimization of the experimental parameters, the analytical characteristics were examined. The linear range between 10–250 μM was obtained for the biosensor developed, with the equation y = 0.0015x + 1.0618 and the correlation coefficient of R2 = 0.9968, respectively (data not shown). The relative standard deviation (R.S.D) value was calculated for 25 μM GSH (n = 6), and found to be 2.92%. On the other hand, the storage stability of the sensor is a very important parameter for long-term applications. When the developed sensor was kept at 4°C in a dry atmosphere for one week, it was observed that 98% of the activity was recovered. Moreover, during this period, 50 measurements were carried out, and no decrease was observed in the signal responses.
On the other hand, the performance of the biosensor developed was compared with that of other electrochemical biosensors devoted for the determination of GSH, in terms of analytical characteristics. The results have been demonstrated in . As clearly seen from the table, the overall performance of the GSH-Px(immob)/Pt-NP/GCPE biosensor is within acceptable limits. Some parameters of the system developed are even better than those of the other biosensors that have been developed.
Table I. A comparison of the analytical characteristics of differently modified electrochemical biosensors for the detection of GSH.
Interference study
In this work, the interferences were considered to be the compounds that are structurally related to GSH and present in biological samples, since this electrode was developed to analyze GSH in synthetically prepared plasma samples. The two potential interfering substances with similar structure, namely ascorbic acid (AA) and cysteine, were used to evaluate the selectivity of GSH-Px(immob)/Pt-NP/GCPE. For this, 25 μmolL− 1 GSH solution, and interfering substances in the amount of 1, 5, 10, 50 and 100-fold more, were added to the working medium. With addition of upto 5-fold and 10-fold, it was found that the developed system was affected to the extent of 7.9% and 5.3% for cysteine and AA, respectively. These values are within acceptable limits, and it can be concluded that this system works in the presence of a ten-fold volume of AA and cysteine. The interference of cysteine arises due to the structural similarity between this compound and the GSH molecule (CitationRover et al. 2001). The feature of the high reactivity of AA may cause interference in determination of GSH. This interference from AA can be minimized by using ascorbate oxidase enzyme, which exhibits high selectivity for the AA oxidation (CitationRover et al. 2001, CitationRaoof et al. 2009).
Sample application
The synthetic plasma solution was prepared as described in the section on sample preparation. Then, the standard addition method was applied. Each analysis was performed thrice, and the recovery of the analytical signal for the diluted samples was calculated as 104.6% ± 0.125. Thus, it can be concluded that the biosensor developed is reliable and sensitive enough for the usage of GSH in matrices like biological fluids.
Conclusions
The GSH-Px(immob)/Pt-NP/GCPE was used as an electrochemical biosensor for the determination of GSH. According to our data, it can be concluded that a sensitive, reproducible, stable, and robust biosensor was obtained. The developed system was also applied for GSH detection in synthetically prepared plasma solution, without applying any sample preparation procedure. The promising recovery value obtained demonstrates that the GSH-Px(immob)/Pt-NP/GCPE biosensor can easily be adapted for GSH detection in real samples. Further studies for developing effective GSH electrochemical.
Acknowledgements
This work is supported by The Scientific and Technical Research Council of Turkiye (TUBITAK) (project no. 109T885). M.C. and F.N.E are gratefully acknowledged the grant from Ege University, Project number 2011 FEN 088.
Declaration of interest
The authors report no declarations of interest. The authors alone are responsible for the content and writing of the paper.
References
- Anık Kırgöz Ü, Marín S, Pumera M, Merkoçi A, Alegret S. 2005. Stripping voltammetry with bismuth modified graphite-epoxy composite electrodes. Electroanalysis. 17:881–886.
- Anık Ü, Çubukçu M. 2008. Examination of the electroanalytic performance of carbon nanotube (CNT) modified carbon paste electrodes as xanthine biosensor transducers. Turk J Chem. 32:711–719.
- Anık Ü, Çevik S. 2009. Double-walled carbon nanotube based carbon paste electrode as xanthine biosensor. Microchim Acta. 166:209–213.
- Anik Ü, Çubukçu M, Çevik S, Timur S. 2010a. Usage of bismuth film electrode as biosensor transducer for alkaline phosphatase assay. Electroanalysis. 22:1519–1523.
- Anik Ü, Çevik S, Pumera M. 2010b. Effect of nitric acid ‘‘washing’’ procedure on electrochemical behavior of carbon nanotubes and glassy carbon μ-particles. Nanoscale Res Lett. 5:846–852.
- Carsol MA, Pouliquen I, Lesgards G, Macini M. 1996. Enzymatic determination of glutathione using electrochemical sensor based on cobalt phthalocyanine screen-printed electrode. Food Technol Biotechnol. 34:147–52.
- Chauhan N, Narang Meena J, Pundir CS. 2012. An amperometric glutathione biosensor based on chitosan–iron coated gold nanoparticles modified Pt electrode. Int J Biol Macromol. 51:879–86.
- Chen XP, Cross RF, Clark AG, Baker WL. 1999. Analysis of reduced glutathione using a reaction with 2,4′-dichloro-1-(naphthyl-4-ethoxy)-S-triazine (EDTN). Microchim Acta. 130:225–231.
- Chen J, He Z, Liu H, Cha C. 2006. Electrochemical determination of reduced glutathione (GSH) by applying the powder microelectrode technique. J Electroanal Chem. 588:324–330.
- Coldur F, Andaç M, Işıldak I. 2010. Flow-injection potentiometric applications of solid state Li+ selective electrode in biological and pharmaceutical samples. J Solid State Electrochem. 14:2241–2249.
- Compagnone D, Massoud R, Di Ilio C, Federici G. 1991. Potentiometric determination of glutathione and glutathione transferase activity. Anal Lett. 24:993–1004.
- Compagnone D, Federici G, Scarciglia L, Palleschi G. 1994. Flow through analysis of glutathione in human erythrocytes with an amperometric biosensor. Anal Lett. 27:15–27.
- Cubukçu M, Ertaş FN, Anık Ü. 2012. Metal/metal oxide micro/nanostructured modified GCPE for GSH detection. Current Analytical Methods. 8:351–357.
- Cubukçu M, Timur S, Anik Ü. 2007. Examination of performance of glassy carbon paste electrode modified with gold nanoparticle and xanthine oxidase for xanthine and hypoxanthine detection. Talanta. 74:434–439.
- Ellman GL. 1959. Tissue sulfhydryl groups Arch. Biochem Biophys. 82:70–77.
- Hou Y, Chrysostome Ndamanisha J, Guo P-L, Peng X-J, Bai J. 2009. Synthesis of ordered mesoporous carbon/cobalt oxide nanocomposite for determination of glutathione. Electrochimica Acta. 54:6166–6171.
- Kinoshita H, Miya T, Kamihira S. 1999. Amperometric determination of glutathione in yeast extract using a membrane-covered phthalocyanine embedded carbon-paste electrode. Bunseki Kagaku. 48:117–120.
- Lehmann, C, Wollenberger U, Brigelius-Flohe R, Scheller FW. 1998. Bioelectrocatalysis by a selenoenzyme. J Electroanalytical Chemistry. 455:259–263.
- Lehmann C, Wollenberger U, Brigelius-Flohe R, Scheller FW. 2001. Modified gold electrodes for electrochemical studies of the reaction of phospholipid hydroperoxide glutathione peroxidase with glutathione and glutathione disulfide. Electroanalysis. 13: 364–369.
- Liu L-P, Yin Z-J, Yang Z-S. 2010. l-cysteine sensor based on pt nanoparticles/poly(o-aminophenol) film on glassy carbon electrode. Bioelectrochemistry. 79:84–89.
- Mao Ü, Ç K. 2000. Amperometric Biosensor for Glutathione Based on Osmium-Polyvinylpyridine Gel Polymer and Glutathione Sulfhydryl Oxidase. Electroanalysis. 12:577–582.
- Raggi MA, Nobile L, Giovannini AG. 1991. Spectrophotometric determination of glutathione and of its oxidation-product in pharmaceutical dosages forms. J Pharm Biomed Anal. 9:1037–1040.
- Raoof J-B, Ojani R, Baghayeri M. 2009. Simultaneous electrochemical determination of glutathione and tryptophan on a nano-TiO2/ ferrocene carboxylic acid modified carbon paste electrode. Sen Actuators B. 143:261–269.
- Rover L, Tatsuo Kubota L, Fenalti Höehr N. 2001. Development of an amperometric biosensor based on glutathione peroxidase immobilized in a carbodiimide matrix for the analysis of reduced glutathione from serum. Clin Chim Acta. 308:55–67.
- Satto SS, Kubota LT, Neto GO. 1999. Biosensor for phenol based on the direct electron transfer blocking of peroxidase immobilising on silica–titanium. Chim Acta. 390:65–72.
- Scheller FW, Wollenberger U, Lei C, Jin W, Ge B, Lehmann C, et al. 2002. Bioelectrocatalysis by redox enzymes at modified electrodes. Rev Mol Biotechnol. 82:411–424.
- Shi G, Lu J, Xu F, Sun W, Jin L, Yamamoto K, et al. 1999. Determination of glutathione in vivo by microdialysis using liquid chromatography with a cobalt hexacyanoferrate chemically modified electrode. Anal Chim Acta. 391:307–313.
- Timur S, Odacı YY, Dincer A, Zihnioglu F, Telefoncu A. 2008. Biosensing Approach for Glutathione Detection Using Glutathione Reductase and Sulfhydryl Oxidase Bienzymatic System. Talanta 817: 239–246.
- Xian YZ, Zhou YY, Wang HT, Zhou LH, Liu F, Jin LT. 2005. Nanostructured electrode based on multi-wall carbon nanotubes/Pt microparticles nanocomposite for electrochemical determination of thiols in rat striatum by high performance liquid chromatography separation. J Chromatogr B. 817:239–246.
- Wang S, Lin X. 2005. Electrodeposition of Pt–Fe(III) nanoparticle on glassy carbon electrode for electrochemical nitric oxide sensor. Electrochim Acta. 50:2887–2891.
- Yang G, Yuan R, Chai Y-Q. 2008. A high-sensitive amperometric hydrogen peroxide biosensor based on the immobilization of hemoglobin on gold colloid/l-cysteine/gold colloid/nanoparticles Pt–chitosan composite film-modified platinum disk electrode. Colloids Surf B Biointerfaces. 61:93–100.