Abstract
Among all cancers that affect women, breast cancer has most mortality rate. It is essential to attain more safe and efficient anticancer drugs. Recent advances in medical nanotechnology and biotechnology have caused in novel improvements in breast and other cancer drug delivery. Methotrexate is an anticancer drug that prevents the dihydrofolate reductase enzyme, which inhibits in the formation of DNA, RNA and proteins which have poor water-solubility. For enhancing the solubility and stability of drugs in delivery systems, we used methotrexate-loaded PLGA- beta-cyclodextrin nanoparticles. The PLGA- beta-cyclodextrin nanoparticles were synthesized by a double emulsion method and characterized with FT-IR and SEM. T47D breast cancer cell lines were treated with equal concentrations of methotrexate-loaded PLGA- beta-cyclodextrin nanoparticles and free methotrexate. MTT assay confirmed that methotrexate-loaded PLGA- beta-cyclodextrin nanoparticles enhanced cytotoxicity and drug delivery in T47D breast cancer cells. These results indicate that encapsulated drugs could be effective in controlled drug release for a sustained period would serve the purpose for long-term treatment of many diseases such as breast cancer.
Introduction
Among all cancers that affect women, breast cancer has most mortality rate. In the way of cancer treatments, the delivery of anticancer drugs may contain straight delivery into the tumor sites (Nejati-Koshki et al. Citation2013, Pourhassan-Moghaddam et al. Citation2013). Currently, chemotherapy is the central way for cancer therapy, but it is permanently having many different side effects (Eatemadi et al. Citation2015, Mollazade et al. Citation2013). In treatment of human cancers using chemotherapic drugs, these drugs can cause some toxic side effects in healthy tissues (Davaran et al. Citation2014). Therefore, nanotechnology attempts to resolve these problems by loading drugs or encapsulating to nanoparticles which are resistant to drug efflux (Daraee et al. Citation2016a, Citation2016b, Eatemadi et al. Citation2014, Citation2016, Seidi et al. Citation2014). In medicine and cancer therapy, nanotechnology has become a prospective and influential application for the advance and progress of nanoparticles as drug delivery systems (Mahapatro and Singh Citation2011, Xu et al. Citation2007). By synthesis of a core-shell, delivery of an well-organized agent to where it is desirable can be easier and competence of treatment can improved and it may be obliging for the treatment of breast cancer (Brannon-Peppas and Blanchette Citation2012, Kafshdooz et al. Citation2015a). Drug delivery research using PLGA polymer throughout the 1970s and 1980s was largely confined to contraceptive steroids and small peptides such as luteinizing hormone releasing hormone (LHRH) analogues (Akbarzadeh et al. Citation2012a, Herizchi et al. Citation2016).This polymer developed the widely used system for curing progressive endometriosis, prostate cancer, and precocious adolescence (Abbasi et al. Citation2015, Herizchi et al. Citation2016). Temporarily, research moved to the delivery of evolving large molecular weight drugs that required constant dosage in perfect form (Akbarzadeh et al. Citation2012a, Citation2013). Their PLGA microspheres had range of 55–95 μm, protein encapsulation productivities greater than 90%, and were capable of attain slow release profiles over 100 d in vitro (Chung et al. Citation2015, Freitas et al. Citation2005). The insertion of PEG in the copolymer reduced the effects of ambiguous protein colloidal and adsorption aggregation, more simplifying lengthy transmission (Gholizadeh-Ghaleh Aziz et al. Citation2015, Zhang and van Duijneveldt Citation2006). Advancement in nanotechnology has created many advanced devices based on PLGA (Davaran et al. Citation2013, Lü et al. Citation2009). By this means, the selective delivery of methotrexate to tumor cells would significantly decrease drug toxicity, and increase its therapeutic effects (Fakhravar et al. Citation2015, Kukowska-Latallo et al. Citation2005).
Methotrexate (2S)-2-[[4-[(2,4-diaminopteridin-6-yl) methyl-methylamine] benzoyl] amino] (MTX) is an anticancer drug that prevents the dihydrofolate reductase enzyme, which inhibits in the formation of DNA, RNA, and proteins (Akbarzadeh et al. Citation2012a, Barbosa et al. Citation2014). Among a variety of drug delivery vehicles, polymer nanoparticles have been further broad as of their facility and prospective to besieged and preferential delivery of drugs to cancer tissues (Akbarzadeh et al. Citation2012b, Ghosh et al. Citation2008).
As discussed above, copolymers such as PCL-PEG, PLGA-PEG, and PLA-PEG promise to be a successful system for the targeted and controlled release of cisplatin, doxorubicin, and 5-fluorouracil with decreased systemic toxicity, increased therapeutic efficiency, and patient compliance (Akbarzadeh et al. Citation2012a, Milani et al. Citation2015). This study explored the cell toxicity effect of the three main chemotherapy drugs (cisplatin, doxorubicin, and 5-fluorouracil) on two cell lines attributed to breast cancer (T47D and MCF7) to obtain an appropriate comparing data(Akbarzadeh et al. Citation2012c, Daraee et al. Citation2015).
Materials and methods
Materials
T47D breast cell lines were prepared from the Pasteur Institute of Iran. Fetal bovine serum (FBS), RPMI-1640, and trypsin-EDTA were purchased from Gibco, Invitrogen (UK). One liter of RPMI 1640 were supplemented with 2 mg sodium bicarbonate (Merck, Germany), and 0.08 mg penicillin-G (Serva co, Germany), 0.2 mg amphotericin B, 10% heat-inactivated fetal bovine serum (FBS) (Gibco, Invitrogen, UK), and 50 mg streptomycin (Merck, Germany). MTT (3(4,5-dimethylthiazol-2-yl) 2,5-diphenyl-tetrazolium bromide), and DMSO (dimethyl sulfoxide) were purchased from Gibco, Invitrogen (UK). D,L-lactide and glycolide, beta-Cyclodextrin, polyvinyl alcohol (PVA), stannous octoate [Sn(Oct)2], stannous 2-ethylhexanoate, and dichloromethane (DCM) were prepared from Sigma-Aldrich (St. Louis, MO). Infrared spectra (FT-IR) were done with Fourier transform infrared spectroscopy (FTIR, Perkin Elmer Series) at room temperature. A KYKY model EM3200 were used for scanning electron microscopy (SEM) measurements. The drug-loading (DL) capacity was recorded using a UV–Vis 2550 spectrometer (Shimadzu). The organic phase was vaporized using a rotary (Rotary Evaporator, Heidolph Instruments, Hei-VAP series). The samples were homogenized using a homogenizer (Silent Crusher M, Heidolph Instruments GmbH, Schwa Bach, Germany).
Synthesis and characterization of poly (lactic-co-glycolic acid) (PLGA)- beta-cyclodextrin (βC) copolymer
PLGA was the introduced choices because of their high bio adaptability and biodegradability combination of therapeutic drugs to polymers and has been actively traced to develop the pharmacological and pharmacokinetic possessions of therapeutic particles. Polyesters, alone and also can amalgamation with other polymers, have been broadly modified for the formulation of NPs. These polymers can be shaped by chemically linking preformed polymers (polymer first method) or by growing polymer chains from a multifunctional motivator. D,L-lactide and glycolide and beta-cyclodextrin were heated while stirring to 40 °C, in a nitrogen atmosphere followed by the addition of 0.05% of stannous octoate [Sn(Oct)2] as the catalyst. After, the temperature was increased to 150 °C and 15 min continued to achieved the viscous blend. The solvent was separated using dissolving the mixture in dichloromethane (DCM) by boiling. The solution was washed with water and diluted with DMC and added dropwise within 30 min to methanol at −30 °C. Finally, the product was filtered off and lyophilized chemical structure of PLGA-β-CD copolymer is shown in . The precipitate was stored in a desiccator until it was used. The morphology of the nanoparticles was characterized using SEM. The FT-IR spectrum was gained from a neat film cast of the chloroform copolymer solution between KBr tablets (Asín et al. Citation2012, Hadjipanayis Costas et al. Citation2010, Kimura et al. Citation2009, Kong et al. Citation2012, Mulens et al. Citation2013, Munaweera et al. Citation2015, Sukumar et al. Citation2013).
Methotrexate loading
PLGA-βC nanoparticles loaded with methotrexate were prepared using the double emulsion method. The methotrexate (10 mg/ml) was added and emulsified in buffer phosphate. Thus, 100 mg of the PLGA-βC nanoparticles was emulsified in the DCM, using probe sonication at 10 W for 45 s and the obtained mixture was added to drug solution. The mixture obtained (w/o emulsion) was added to an aqueous solution of polyvinyl alcohol (PVA) 1%, and was then probe sonicated for 1 min, until the evaporation of the organic phase was complete and the mixture obtained (w/o/w emulsion) was gently stirred at room temperature. Nanoparticles gained by three times centrifugation at 12,000 rpm for 15 min and were isolated. Nanoparticles supernatants were detached and stockpiled for subsequent experiments (Asin et al. Citation2012, Cervadoro et al. Citation2013, Ding et al. Citation2013, Landi Citation2013, Mulens et al. Citation2013, Obaidat et al. Citation2015, Sadhukha et al. Citation2013).
Determination of methotrexate entrapment efficiency (EE) and drug loading (DL)
To calculate the encapsulation efficiency (EE) and the drug-loading (DL) rate of the obtained solution, for methotrexate, the supernatant was isolated and used for comparison with the total amount of methotrexate.
The amount of non-encapsulated methotrexate in the supernatant was calculated by using an ultraviolet 2550 spectrophotometer (Shimadzu), with an absorbance peak at 299 nm. The following formula was used to measure the percent of methotrexate encapsulated in the nanoparticles (EquationEquation (1)(1) ), and the rate of DL (EquationEquation (2)
(2) ) (Babu et al. Citation2013, Disch et al. Citation2012, Gudoshnikov et al. Citation2012, Jiang et al. Citation2013, Rivas et al. Citation2012).
(1)
(2)
Cell culture and cell line
T47D breast cell lines were cultured in RPMI1640 medium complemented with and 2 mg/ml sodium bicarbonate, 0.05 mg/ml penicillin-G, 0.08 mg/ml of streptomycin, 10% heat-inactivated FBS, and the cells were grown at 37 °C in an incubator with 55% humidity and 5% CO2.
Cell viability and MTT-based cytotoxicity test (Mellatyar et al. Citation2014)
T47D breast cell lines were exposed to free methotrexate and methotrexate-loaded PLGA-βC in the exponential phase of growth and cytotoxicity assay was calculated at 24 h after treatment using the MTT assay. Ten thousand cells/well in a 96-well plate were seeded and reserved for 24 h in the incubator to promote cell attachment. Then T47D cell lines were treated in the triplicate manner with different concentrations of free methotrexate and methotrexate-loaded PLGA-βC (0–320 μM) for 24 h. Three controls were used such as cells alone, 1% DMSO, and PLGA-βC control for estimation of nanoparticle effect. After 24 h drug exposure durations, the medium was substituted with 200 μL fresh RPMI-1640 medium for 24 h. The cells were incubated with 50 μL of 2 mg/ml MTT which dissolved in PBS, for 4 h. Then, the content of all wells was replaced with 200 μL pure DMSO and 25 μL Sorensen’s glycine buffer. Finally, in a reference wavelength of 630 nm, an ELISA-reader was used to estimate the absorbance measurement at 570 nm (Baviskar et al. Citation2012, Cámara et al. Citation2014, Cervadoro et al. Citation2013, Gudoshnikov et al. Citation2012, Citation2013, Martinez-Boubeta et al. Citation2013, Thakor et al. Citation2013).
Results
NMR and FT-IR spectrum of methotrexate, PLGA-βC, and methotrexate-loaded PLGA-βC
The chemical structures of the copolymers composition of the copolymers were determined with H-NMR (Bruker spectra spin 400 MHz, Leipzig, Germany) (). The first must freeze dried nanoparticles, then dissolved in DMSO showed the configuration for the particles was closely the same as that of the original matrix. Although the H-NMR spectra of polyesters show the resonance signals for typical linear PLGA protons, there are other peaks, due to the amalgamation of β-CD. FT-IR spectroscopy was done by Shimadzu spectrophotometer. FT-IR spectra of methotrexate-loaded methotrexate and PLGA-βC are described. The characteristic peak of methotrexate was showed in at 3464 cm−1 (-NH stretching), 1648.4 cm−1 (COOH), 1653 cm−1 (CONH) and 853.88 cm−1 (aromatic stretch). Successfully, PLGA-βC co-polymer nanoparticles were perpetrated by ring-opening copolymerization of PLGA and βC. The FT-IR spectrum is compatible with the structure of presupposed PLGA-βC copolymer. FT-IR spectroscopy was used to show the original and main structure of PLGA-βC copolymer nanoparticle. FT-IR spectral data were used to confirm the chemical stability of methotrexate in PLGA-βC. These absorption bands indicate that the PLGA-βC block copolymer was successfully synthesized and encapsulated in methotrexate. The FT-IR spectra of methotrexate, PLGA-βC, and methotrexate-loaded PLGA-βC are shown in , , and , respectively.
Determination of morphology of the drug-loaded nanoparticle
The morphology of methotrexate-poly (lactic-co-glycolic acid) (PLGA)-beta-cyclodextrin (βC) copolymer was detected using a scanning electron microscopy (SEM). For the SEM, the lyophilized copolymers were located on a double stick tape over aluminum stubs to become a uniform layer of nanoparticles. Prior to investigation, samples were ready on aluminum stubs and covered with gold under argon atmosphere by means of a sputter coater. displays polymer containing methotrexate. The average particle size of samples was 70 to 200 nm.
Entrapment efficiency (EE) and drug loading (DL)
The methotrexate content in the methotrexate-poly (lactic-co-glycolic acid) (PLGA)-beta-cyclodextrin (βC) copolymer was calculated using the ultraviolet spectrophotometer. The methotrexate-loading content and drug entrapment efficiency were calculated based on the EquationEquations (1)(1) and Equation(2)
(2) , respectively. The drug loading for methotrexate was 18%. The entrapment efficiency for methotrexate was 80%.
Cell cytotoxicity (MTT assay)
In this study cell viability was assessed by MTT assay through exposing T47D breast cancer cell line to different concentrations of pure methotrexate and methotrexate-loaded PLGA-βC for 24 h. The T47D cell toxicity was increased by rising the methotrexate amount, which shows the way that methotrexate is dose-dependent and the cell viability is opposite. The pure methotrexate had cytotoxic effect on T47D breast cancer cell lines.
Inhibitory concentration at 50% (IC50) of free methotrexate on T47D was 0.391, 0.361, and 0.285 mg/ml for 24, 48, and 72 h, respectively. IC50 of methotrexate-poly (lactic-co-glycolic acid) (PLGA)-beta-cyclodextrin (βC) copolymer on T47D was 0.318, 0.294, and 0.241 mg/ml for 24, 48, and 72 h, respectively ( ). DMSO and PCL-PEG have very low cytotoxicity effect on the T47D cells because PLGA-βC and DMSO 1% showed an absorbance value equivalent of 99 and 98% of control for T47D cell lines. The PRISM 4 program was used for plotting the graphs. Cytotoxicity study of free drug and drug loaded nanoparticles on T47D cell line after, 24, 48, and 72 h exposure are shown in .
Figure 7. Cytotoxicity effect of PLGA-βC methotrexate complex and free methotrexate on T47D for 24 h. *: P values < 0.05 was considered statistically significant.
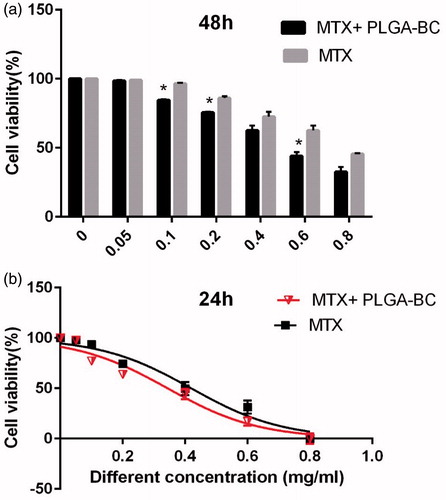
Figure 8. Cytotoxicity effect of PLGA-βC methotrexate complex and free methotrexate on T47D for 48 h. *: P values < 0.05 was considered statistically significant.
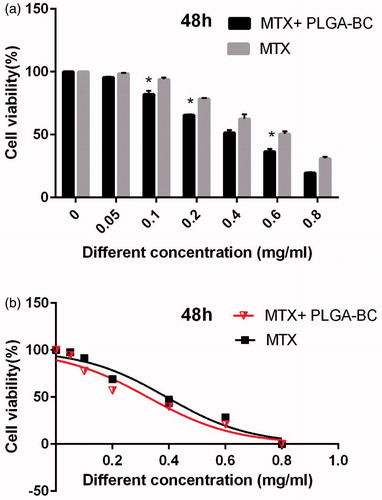
Discussion and conclusion
In treatment of human cancers using chemotherapic drugs, these drugs can cause some toxic side effects in healthy tissues. Therefore, nanotechnology attempts to resolve these problems by loading drugs or encapsulating to nanoparticles which are resistant to drug efflux (Akbarzadeh et al. Citation2012d, Daraee et al. Citation2016a, Eatemadi et al. Citation2014, Citation2016, Tsuda Citation2010). In these studies, we examined the antiproliferative effects of methotrexate-loaded PLGA-βC and methotrexate free on T47D breast cancer cell line. Methotrexate is an anticancer drug that prevents the dihydrofolate reductase enzyme, which inhibits in the formation of DNA, RNA, and proteins (Ahmadi-Aghkand et al. Citation2015, Barbosa et al. Citation2014). Methotrexate-loaded poly (lactic-co-glycolic acid) (PLGA)-beta-cyclodextrin(βC) copolymer is prepared by the double emulsion method (Majidi et al. Citation2015). The FT-IR spectra did not identify any accumulated drug material in the freshly prepared freeze-dried nanoparticles (Kafshdooz et al. Citation2015a, Mellatyar et al. Citation2014). The analysis was shown that polymer was successfully synthesized. MTT assay showed that methotrexate-loaded PLGA-βC has more cell death effect than free methotrexate on T47D breast cancer cell line in same condition. Several studies have been shown that encapsulating drugs to PLGA or PCL nanoparticles can effectively decreases dosage of drug and causes low adverse side effects of the drug (Alimohammadi and Joo Citation2014, Eatemadi et al. Citation2015, Nejati-Koshki et al. Citation2013). The results suggest that methotrexate-loaded poly (lactic-co-glycolic acid) (PLGA)-beta-cyclodextrin (βC) copolymer is appropriate candidate for the chemotherapy. The entrapment of methotrexate within poly (lactic-co-glycolic acid) (PLGA)-beta-cyclodextrin (βC) copolymer remarkably enhanced the IC50 and cell toxicity parameters of methotrexate. Also, either free or encapsulated methotrexate effect at minimum amount on T47D was compared with free and encapsulated methotrexate (Kafshdooz et al. Citation2015b). These results indicate that methotrexate-loaded poly (lactic-co-glycolic acid) (PLGA)-beta-cyclodextrin (βC) copolymer nanoparticles could be effective in controlled drug release for a sustained period would serve the purpose for long term treatment of many disease such as breast cancer.
Funding information
This work is funded by a 2015 grant Drug Applied Research Center, Tabriz University of Medical Sciences.
Disclosure statement
The authors report no declaration of interest. The authors alone are responsible for the content and writing of the paper.
References
- Abbasi E, Akbarzadeh A, Kouhi M, Milani M. 2015. Graphene: synthesis, bio-applications, and properties. Artif Cells Nanomed Biotechnol. 44:150–156.
- Ahmadi-Aghkand F, Gholizadeh-Ghaleh Aziz S, Panahi Y, Daraee H, Gorjikhah F, Gholizadeh-Ghaleh Aziz S, Hsanzadeh A, Akbarzadeh A. 2015. Recent prospective of nanofiber scaffolds fabrication approaches for skin regeneration. Artif Cells Nanomed Biotechnol. [Epub ahead of print]. doi: 10.3109/21691401.2015.1111232.
- Akbarzadeh A, Mikaeili H, Zarghami N, Mohammad R, Barkhordari A, Davaran S. 2012a. Preparation and in vitro evaluation of doxorubicin-loaded Fe3O4 magnetic nanoparticles modified with biocompatible copolymers. Int J Nanomedicine. 7:511–526.
- Akbarzadeh A, Rezaei-Sadabady R, Davaran S, Joo SW, Zarghami N, Hanifehpour Y, et al. 2013. Liposome: classification, preparation, and applications. Nanoscale Res Lett. 8:102.
- Akbarzadeh A, Samiei M, Davaran S. 2012b. Magnetic nanoparticles: preparation, physical properties, and applications in biomedicine. Nanoscale Res Lett. 7:1–13.
- Akbarzadeh A, Samiei M, Joo SW, Anzaby M, Hanifehpour Y, Nasrabadi HT, Davaran S. 2012c. Synthesis, characterization and in vitro studies of doxorubicin-loaded magnetic nanoparticles grafted to smart copolymers on A549 lung cancer cell line. J Nanobiotechnology. 10:46.
- Akbarzadeh A, Zarghami N, Mikaeili H, Asgari D, Goganian AM, Khiabani HK, Samiei M, Davaran S. 2012d. Synthesis, characterization, and in vitro evaluation of novel polymer-coated magnetic nanoparticles for controlled delivery of doxorubicin. Nanotechnol Sci Appl. 5:13–25.
- Alimohammadi YH, Joo SW. 2014. PLGA-based nanoparticles as cancer drug delivery systems. Asian Pac J Cancer Prev. 15:517–535.
- Asin L, Ibarra MR, Tres A, Goya GF. 2012. Controlled cell death by magnetic hyperthermia: effects of exposure time, field amplitude, and nanoparticle concentration. Pharm Res. 29:1319–1327.
- Babu A, Templeton AK, Munshi A, Ramesh R. 2013. Nanoparticle-based drug delivery for therapy of lung cancer: progress and challenges. J Nanomaterials. 2013:863951.
- Barbosa JA, Zoppi A, Quevedo MA, de Melo PN, de Medeiros AS, Streck L, et al. 2014. Triethanolamine stabilization of methotrexate-β-cyclodextrin interactions in ternary complexes. Int J Mol Sci. 15:17077–17099.
- Baviskar DT, Amritkar AS, Chaudhari HS, Jain DK. 2012. Modulation of drug release from nanocarriers loaded with a poorly water soluble drug (flurbiprofen) comprising natural waxes. Pharmazie. 67:701–705.
- Brannon-Peppas L, Blanchette JO. 2012. Nanoparticle and targeted systems for cancer therapy. Adv Drug Deliv Rev. 64:206–212.
- Cámara CI, Monzón LMA, Coey JMD, Yudi LM. 2014. Assembly of magnetic nanoparticles at liquid/liquid interface. Catalytic effect on ion transfer process. J Chem Phys. 143:77–83.
- Cervadoro A, Giverso C, Pande R, Sarangi S, Preziosi L, Wosik J, Brazdeikis A, Decuzzi P. 2013. Design maps for the hyperthermic treatment of tumors with superparamagnetic nanoparticles. PLoS one. 8:e57332.
- Chung JH, Kim YK, Kim KH, Kwon TY, Vaezmomeni SZ, Samiei M, et al. 2015. Synthesis, characterization, biocompatibility of hydroxyapatite–natural polymers nanocomposites for dentistry applications. Artif Cells Nanomed Biotechnol. 44:277–284.
- Daraee H, Eatemadi A, Abbasi E, Fekri Aval S, Kouhi M, Akbarzadeh A. 2016a. Application of gold nanoparticles in biomedical and drug delivery. Artif Cells Nanomed Biotechnol. 44:410–422.
- Daraee H, Etemadi A, Kouhi M, Alimirzalu S, Akbarzadeh A. 2016b. Application of liposomes in medicine and drug delivery. Artif Cells Nanomed Biotechnol. 44:381–391.
- Daraee H, Pourhassanmoghadam M, Akbarzadeh A, Zarghami N, Rahmati-Yamchi M. 2015. Gold nanoparticle–oligonucleotide conjugate to detect the sequence of lung cancer biomarker. Artif Cells Nanomed Biotechnol. [Epub ahead of print]. doi: 10.3109/21691401.2015.1031905.
- Davaran S, Akbarzadeh A, Nejati-Koshki K, Alimohammadi S, Ghamari MF, Soghrati MM, Rezaei A, Khandaghi AA. 2013. In vitro studies of NIPAAM-MAA-VP copolymer-coated magnetic nanoparticles for controlled anticancer drug release*. JEAS. 3:108–115.
- Davaran S, Rezaei A, Alimohammadi S, Khandaghi AA, Nejati-Koshki K, Nasrabadi HT, Akbarzadeh A. 2014. Synthesis and physicochemical characterization of biodegradable star-shaped poly lactide-co-glycolide-β-cyclodextrin copolymer nanoparticles containing albumin. J Adv Nanoparticles. 3:14–22.
- Ding W, Wang F, Zhang J, Guo Y, Ju S, Wang H. 2013. A novel local anti-colorectal cancer drug delivery system: negative lipidoid nanoparticles with a passive target via a size-dependent pattern. Nanotechnology. 24:375101.
- Disch S, Wetterskog E, Hermann RP, Wiedenmann A, Vainio U, Salazar-Alvarez G, Bergström L, Brückel Th. 2012. Quantitative spatial magnetization distribution in iron oxide nanocubes and nanospheres by polarized small-angle neutron scattering. New J Phys. 14:013025.
- Eatemadi A, Darabi M, Afraidooni L, Zarghami N, Daraee H, Eskandari L, Mellatyar H, Akbarzadeh A. 2015. Comparison, synthesis and evaluation of anticancer drug-loaded polymeric nanoparticles on breast cancer cell lines. Artif Cells Nanomed Biotechnol. [Epub ahead of print]. doi: 10.3109/21691401.2015.1008510.
- Eatemadi A, Daraee H, Karimkhanloo H, Kouhi M, Zarghami N, Akbarzadeh A, et al. 2014. Carbon nanotubes: properties, synthesis, purification, and medical applications. Nanoscale Res Lett. 9:1–13.
- Eatemadi A, Daraee H, Zarghami N, Melat Yar H, Akbarzadeh A. 2016. Nanofiber: synthesis and biomedical applications. Artif Cells Nanomed Biotechnol. 44:111–121.
- Fakhravar Z, Ebrahimnejad P, Daraee H, Akbarzadeh A. 2015. Nanoliposomes: synthesis methods and applications in cosmetics. J Cosmet Laser Ther. 12:1–31.
- Freitas S, Merkle HP, Gander B. 2005. Microencapsulation by solvent extraction/evaporation: reviewing the state of the art of microsphere preparation process technology. J Control Release. 102:313–332.
- Gholizadeh-Ghaleh Aziz S, Gholizadeh-Ghaleh aziz S, Akbarzadeh A. 2015. The potential of nanofibers in tissue engineering and stem cell therapy. Artif Cells Nanomed Biotechnol. [Epub ahead of print]. doi: 10.3109/21691401.2015.1029627.
- Ghosh P, Han G, De M, Kim CK, Rotello VM. 2008. Gold nanoparticles in delivery applications. Adv Drug Deliv Rev. 60:1307–1315.
- Gudoshnikov SA, Liubimov B, Sitnov Y, Skomarovsky V, Usov N. 2013. AC magnetic technique to measure specific absorption rate of magnetic nanoparticles. J Supercond Nov Magn. 26:857–860.
- Gudoshnikov SA, Ya Liubimov B, Usov NA. 2012. “Hysteresis losses in a dense superparamagnetic nanoparticle assembly.” AIP Advances. 2:012143.
- Hadjipanayis Costas G, Machaidze R, Kaluzova M, Wang L, Schuette AJ, Chen H, Wu X, Mao H. 2010. EGFRvIII antibody–conjugated iron oxide nanoparticles for magnetic resonance imaging–guided convection-enhanced delivery and targeted therapy of glioblastoma. Cancer Res. 70:6303–6312.
- Herizchi R, Abbasi E, Milani M, Akbarzadeh A. 2016. Current methods for synthesis of gold nanoparticles. Artif Cells Nanomed Biotechnol. 44:596–602.
- Jiang L, Li X, Liu L, Zhang Q. 2013. Thiolated chitosan-modified PLA-PCL-TPGS nanoparticles for oral chemotherapy of lung cancer. Nanoscale Res Lett. 8:66.
- Kafshdooz L, Kafshdooz T, Razban Z, Akbarzadeh A. 2015a. The application of gold nanoparticles as a promising therapeutic approach in breast and ovarian cancer. Artif Cells Nanomed Biotechnol. [Epub ahead of print]. doi: 10.3109/21691401.2015.1029625.
- Kafshdooz T, Kafshdooz L, Akbarzadeh A, Hanifehpour Y, Joo SW. 2015b. Applications of nanoparticle systems in gene delivery and gene therapy. Artif Cells Nanomed Biotechnol. 44:581–587.
- Kimura S, Egashira K, Chen L, Nakano K, Iwata E, Miyagawa M, et al. 2009. Nanoparticle-mediated delivery of nuclear factor κB decoy into lungs ameliorates monocrotaline-induced pulmonary arterial hypertension. Hypertension. 53:877–883.
- Kong M, Li X, Wang C, Ding C, Dong A, Duan Q, Shen Z. 2012. Tissue distribution and cancer growth inhibition of magnetic lipoplex-delivered type 1 insulin-like growth factor receptor shRNA in nude mice. Acta Biochim Biophys Sin (Shanghai). 44:591–596.
- Kukowska-Latallo JF, Candido KA, Cao Z, Nigavekar SS, Majoros IJ, Thomas TP, et al. 2005. Nanoparticle targeting of anticancer drug improves therapeutic response in animal model of human epithelial cancer. Cancer Res. 65:5317–5324.
- Landi GT. 2013. Simple models for the heating curve in magnetic hyperthermia experiments. J Magn Magn Mater. 326:14–21.
- Lü JM, Wang X, Marin-Muller C, Wang H, Lin PH, Yao Q, Chen C. 2009. Current advances in research and clinical applications of PLGA-based nanotechnology. Expert Rev Mol Diagn. 9:325–341.
- Mahapatro A, Singh DK. 2011. Biodegradable nanoparticles are excellent vehicle for site directed in-vivo delivery of drugs and vaccines. J Nanobiotechnology. 9:55.
- Majidi S, Zeinali Sehrig F, Farkhani SM, Soleymani Goloujeh M, Akbarzadeh A. 2015. Current methods for synthesis of magnetic nanoparticles. Artif Cells Nanomed Biotechnol. 44:722–734.
- Martinez-Boubeta C, Simeonidis K, Makridis A, Angelakeris M, Iglesias O, Guardia P, et al. 2013. Learning from nature to improve the heat generation of iron-oxide nanoparticles for magnetic hyperthermia applications. Sci Rep. 3:1652.
- Mellatyar H, Akbarzadeh A, Rahmati M, Ghalhar MG, Etemadi A, Nejati-Koshki K, Zarghami N, Barkhordari A. 2014. Comparison of inhibitory effect of 17-DMAG nanoparticles and free 17-DMAG in HSP90 gene expression in lung cancer. Asian Pac J Cancer Prev. 15:8693–8698.
- Milani M, Sharifi Y, Rahmati-Yamchi M, Somi MH, Akbarzadeh A. 2015. Immunology and vaccines and nanovaccines for Helicobacter pylori infection. Expert Rev Vaccines. 14:833–840.
- Mollazade M, Nejati-Koshki K, Akbarzadeh A, Hanifehpour Y, Zarghami N, Joo S. 2013. PAMAM dendrimers augment inhibitory effects of curcumin on cancer cell proliferation: possible inhibition of telomerase. Asian Pac J Cancer Prev. 14:6925–6928.
- Mulens V, María del Puerto M, Barber DF. 2013. Development of magnetic nanoparticles for cancer gene therapy: a comprehensive review. ISRN Nanomaterials. 2013:646284.
- Munaweera I, Shi Y, Koneru B, Saez R, Aliev A, Di Pasqua AJ, Balkus KJ Jr. 2015. Chemoradiotherapeutic magnetic nanoparticles for targeted treatment of nonsmall cell lung cancer. Mol Pharm. 12:3588–3596.
- Nejati-Koshki K, Akbarzadeh A, Pourhasan-Moghadam M, Joo S. 2013. Inhibition of leptin and leptin receptor gene expression by silibinin-curcumin combination. Asian Pac J Cancer Prev. 14:6595–6599.
- Obaidat IM, Issa B, Haik Y. 2015. Magnetic properties of magnetic nanoparticles for efficient hyperthermia. Nanomaterials. 5:63–89.
- Pourhassan-Moghaddam M, Rahmati-Yamchi M, Akbarzadeh A, Daraee H, Nejati-Koshki K, Hanifehpour Y, Joo SW. 2013. Protein detection through different platforms of immuno-loop-mediated isothermal amplification. Nanoscale Res Lett. 8:1–11.
- Rivas J, Bañobre-López M, Piñeiro-Redondo Y, Rivas B, López-Quintela MA. 2012. Magnetic nanoparticles for application in cancer therapy. J Magn Magn Mater. 324:3499–3502.
- Sadhukha T, Wiedmann TS, Panyam J. 2013. Inhalable magnetic nanoparticles for targeted hyperthermia in lung cancer therapy. Biomaterials. 34:5163–5171.
- Seidi K, Eatemadi A, Mansoori B, Jahanban-Esfahlan R, Farajzadeh D. 2014. Nanomagnet-based detoxifying machine: an alternative/complementary approach in HIV therapy. J AIDS Clin Res. 5:304. doi:10.4172/2155-6113.1000304.
- Sukumar UK, Bhushan B, Dubey P, Matai I, Sachdev A, Packirisamy G. 2013. Emerging applications of nanoparticles for lung cancer diagnosis and therapy. Int Nano Lett. 3:1–17.
- Thakor AS, Gambhir SS. 2013. Nanooncology: the future of cancer diagnosis and therapy. CA Cancer J Clin. 63:395–418.
- Tsuda H. 2010. Risk assessment studies of nanomaterials in Japan and other countries. Asian Pac J Cancer Prev. 11:13–14.
- Xu T, Zhang N, Nichols HL, Shi D, Wen X. 2007. Modification of nanostructured materials for biomedical applications. Mater Sci Eng C. 27:579–594.
- Zhang Z, van Duijneveldt JS. 2006. Experimental phase diagram of a model colloid-polymer mixture in the protein limit. Langmuir. 22:63–66.