Abstract
Double-stranded RNAs are an important class of functional macromolecules in living systems. They are usually found as part of highly specialized intracellular machines that control diverse cellular events, ranging from virus replication, antiviral defense, RNA interference, to regulation of gene activities and genomic integrity. Within different intracellular machines, the RNA duplex is often found in association with specific RNA-dependent ATPases, including Dicer, RIG-I and DRH-3 proteins. These duplex RNA-activated ATPases represent an emerging group of motor proteins within the large and diverse super family 2 nucleic acid-dependent ATPases (which are historically defined as SF2 helicases). The duplex RNA-activated ATPases share characteristic molecular features for duplex RNA recognition, including motifs (e.g., motifs IIa and Vc) and an insertion domain (HEL2i), and they require double-strand RNA binding for their enzymatic activities. Proteins in this family undergo large conformational changes concomitant with RNA binding, ATP binding and ATP hydrolysis in order to achieve their functions, which include the release of signaling domains and the recruitment of partner proteins. The duplex RNA-activated ATPases represent a distinct and fascinating group of nanomechanical molecular motors that are essential for duplex RNA sensing and processing in diverse cellular pathways.
Background
Many cellular processes result in the production of double-stranded RNA molecules, including transcription of convergent cellular genes or mobile genetic elements, self-annealing of cellular transcripts and the replication of common RNA viruses. Duplex RNAs are important for numerous cellular functions, including gene regulation, chromatin remodeling, antiviral defense and maintenance of genomic integrity.Citation1,Citation2 Most of these processes involve the interaction of double-stranded RNAs with conserved and highly specialized intracellular machines. Well-characterized examples include Dicer and the RIG-I like receptors, as well as Dicer-like RNA helicases 1 and 3 (DRH-1 and -3), which are two mechanical proteins involved in the RNA interference (RNAi) pathway in worms.Citation1,Citation3 Although there are fundamental differences between these proteins, they share a similar, highly conserved motor domain that is essential for duplex RNA sensing, signaling and processing. This domain is similar in sequence and form, if not function, to the helicase domain that is found in many DNA and RNA remodeling proteins.Citation4,Citation5
Helicases have been classically defined as enzymes that couple ATP hydrolysis to the unwinding of nucleic acid duplexes, and they were originally phylogenetically grouped into families based on sequence conservation rather than function.Citation6 However, these family members were subsequently shown to have diverse mechanical functions, of which duplex unwinding is only one type of activity. Therefore, these enzymes are now commonly referred to as nucleic acid remodeling proteins or, perhaps more correctly, as nucleic acid-dependent ATPases.Citation5,Citation7 Other classifications have grouped these proteins by their nucleic acid target (RNA or DNA), the nucleic acid strandness (α for single stranded NA or β for double stranded NA) and the translocation polarity on the nucleic acid (A for 3′ to 5′ or B for 5′ to 3′) as defined by Wigley et al.Citation8 Sequence and structure analysis have revealed a common arrangement of conserved motifs for the Superfamily 1 and 2 (SF1 and 2) nucleic acid dependent ATPases.Citation5 In these proteins, two conserved RecA-like domains lie against each other, forming a cleft that binds and hydrolyzes ATP, thereby serving as the catalytic core. This ATPase core includes conserved motifs Q, I, II and VI (), which are aligned and rigidified through binding of RNA along the surface of the RecA folds. Conserved motifs Ia, Ib, Ic, IV, IVa, V and Vb mediate RNA binding, while motifs III and Va help to couple nucleic acid binding with ATP hydrolysis. Despite the high degree of conservation in both RNA binding and ATPase motifs, SF1 and SF2 proteins have unique functions and are usually not interchangeable. Specialization in mechanical function and the presence of accessory domains makes each nucleic acid-dependent ATPase unique.Citation4 These enzymes are involved in every aspect of nucleic acid metabolism in all living organisms and viruses.Citation5,Citation9-Citation11 Because of their conserved molecular functions, they are also heavily involved in genetic, autoimmune and infectious diseases, and they are potential targets for drug discovery.
Figure 1. DRAs and related nucleic acid-dependent ATPases. (A) The conserved SF2 ATPase/helicase core. The figure is prepared from eIF4aIII, which is a component of the exon junction complex (PDB: 2J0S). Positions of the characteristic motifs are highlighted. (B) Domain organization of DRAs. Domains are not to the scale. C-terminal regions that resemble the ATPase domains of Dicer and FANCM-like proteins are simplified and are not labeled. (C) Schematic cladogram showing DRAs within the SF2 family of proteins, specifically the DEAD box family and double stranded nucleic acid binding ATPases. The alignment and family trees were determined with the UGENE software package.Citation118 The multiple sequence alignment was run with T-CoffeeCitation119 on the core ATPase/helicase domains listed in and the family tree was determined using the PHYLIP Neighbor Joining method with the Jones-Taylor-Thornton distance matrix. Pair-wise sequence identity for the ATPase core regions of DRAs range from the highest 42% (hsMDA5: hsLGP2) and 36% (hsRIG-I: hsMDA5), to 26% (hsRIG-I: ceDRH-1) and 21% (ceDRH-1: ceDRH-3), with the lowest 14% between hsDicer1 and ceDRH-3.
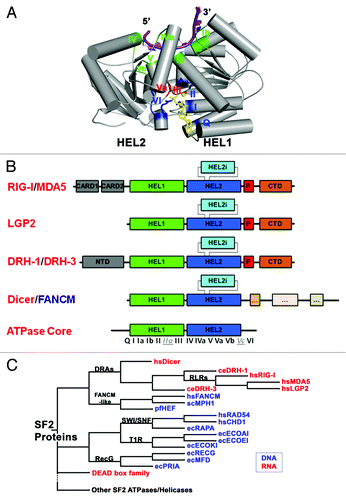
Recently, significant progress has been made in our understanding of RNA-dependent ATPases, including the identification and characterization of new examples like DRH-1 and DRH-3Citation12,Citation13 from nematodes and new structural and functional insights of known cases like DicerCitation14 and RIG-I.Citation15 In this review, we focus on this emerging group of specialized RNA-dependent ATPases that include Dicer, a ribonuclease that plays an essential role in miRNA and siRNA biogenesisCitation14; RIG-I, an intracellular surveillance protein for detecting pathogenic RNACitation15; and DRH-3, Dicer-related-helicase-3, a component of the siRNA pathway from Caenorhabditis elegans.Citation12,Citation13 The mechanistic feature shared by all these proteins [hereafter named Duplex RNA-activated ATPases (DRAs)] is that dsRNA is required to stimulate their ATPase activity and thereby activate all subsequent functions, which is in sharp contrast to other SF2 proteins that are specifically activated by single-stranded RNA. Further, unlike the bona fide RNA helicases, DRAs are unlikely to display RNA unwinding activity.Citation16,Citation17 Rather, the conformational changes that occur upon binding to RNA and ATP are coupled to other processes, such as the release of signaling domains and binding to partner proteins. Here we review the discovery of the DRAs, highlight recent advances in understanding of their function and discuss how this is related to their structural features.
Comparison of DRAs with Related Mechanical Proteins
DRA proteins are phylogenetically classified as a subgroup within Helicase Superfamily 2 (known as SF2 proteins, ),Citation4 and present a core ATPase domain that is very similar in both sequence and structure to the DEAD box family ATPases/Helicases. Unlike DEAD box proteins, DRAs contain a unique α-helical insertion domain (HEL2i) within the second RecA fold of the core ATPase/Helicase domain. Structural studies have shown that this adaptation is important for duplex RNA binding ( and C).Citation17-Citation19 As the closest phylogenetic relatives of of RIG-I, innate immune sensors MDA5 and LGP2 are more similar to each other than to RIG-I, although there are conflicting reports on which of these proteins should be considered the evolutionary precedent of the others.Citation20,Citation21 A constructed family tree of SF2 proteins from several subgroups suggests that DRH-1 is more closely related to RIG-I than to Dicer (), underscoring the difficulties in naming these proteins based on functional associations.
Perhaps most significant given their function, DRAs are most closely related to proteins that function not on RNA, but on double-stranded DNA (). The DRAs are relatives of the FANCM family of proteins that function during DNA repair, and these include Hef, FANCM, Mph1 and Fml1 (http://www.rnahelicase.org/rig.htm database) ( and ). Members of the FANCM family bind dsDNA and contain a similar α-helical HEL2i insertion as the DRAs.Citation22 Other closely related dsDNA binding proteins include members of the Swi/Snf, RecG and the T1R families ( and ).Citation4 Swi/Snf proteins have a six α helix motif that is inserted within two parts of the HEL2 domain. This motif interacts with the 5′ strand of dsDNA and occupies the same position relative to HEL1 and HEL2 as the Hef and the RIG-I HEL2i domain.Citation23-Citation25 Interestingly, RecG proteins have a TRG motif (translocation in RecG) located immediately after motif VI that forms a helical hairpin proposed to be a transmission system for driving double-stranded translocation.Citation26 This hairpin appears to be analogous to the pincer domain of RIG-I and other DRAs, although the pincer domain is significantly longer and more complex.Citation27 Similarly, members of the prokaryotic T1R family have an α-helical domain at their C terminus that plays a role in recognizing foreign dsDNA, either from transmissible plasmids or from phages.Citation28
Table 1. SF2 nucleic acid-dependent ATPases listed in this study
In addition to the conserved ATPase/Helicase core and the specialized RNA recognition and transduction domains, DRAs contain two semi-conserved motifs that contribute to binding of double-stranded nucleic acid (). In these proteins, motifs IIa and Vc form contacts with the 5′-3′ “second strand,” which contrasts with the other nucleic acid binding motifs that contact only the 3′-5′ “tracking strand” that is bound by all translocative helicases. Motif IIa in particular was initially noted in the Sulfolobus solfataricus Rad54 structure and later within the motor subunit of the T1R restriction modification enzyme, EcoR124, from Escherichia coli.Citation23,Citation29 Not surprisingly, motif IIa also appears to be present in members of the DEAD box family, and its functional role in duplex RNA binding is supported by recent structural studies of DEAD-box protein Mss116 bound to duplex RNA.Citation30 Motifs IIa and Vc are primarily conserved structurally rather than in primary sequence ().Citation17,Citation23,Citation29 Although there are semi-conserved lysines and asparagines in motif IIa and a semi-conserved asparagine in motif Vc, the majority of the contacts made with nucleic acids involve the peptide backbone.
Figure 2. Sequence and structural features of RIG-I that contribute to duplex RNA recognition. (A) Sequence alignment of DRAs and other SF2 proteins. Notice motifs IIa and Vc (boxed in dotted lines) are not very conserved in amino acid sequence. (B) HEL2i domain juxtaposes with the duplex RNA backbone (PDB codes: 3TMI in green; 2YKG in yellow; 4A36, in magenta). (C) Specialized motifs IIa and Vc recognize the top strand of the duplex RNA (Botton strand or tracking strand is the strand nucleic acid that binds to the SF2α proteins; Top strand is the complementary strand). (D) Possible structural conservation of motif IIa and motif Vc found in DEAD-box RNA family members. Figure shows the aligned structures of DEAD-box protein:ssRNA complexes with duplex RNA (PDB codes: 2J0S, 3I5X, 3G0H and 2DB3). The possible presence of motif IIa and Vc in DEAD-box proteins are labeled in parenthesis.
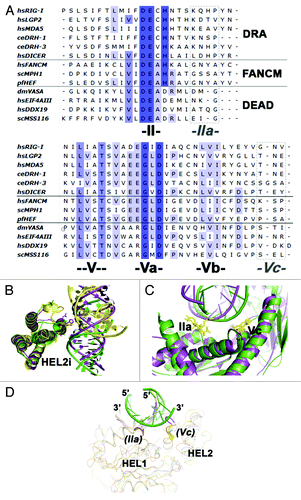
By contrast, RNA-dependent ATPases that function as helicase enzymes preferentially bind single stranded nucleic acid before unwinding adjacent duplex regions. Two distinct mechanisms of unwinding have been proposed, and these include melting of the RNA backbone through local distortions of the A-form duplex RNA, as proposed for the DEAD box family of helicases including Ded1p and Mss116p,Citation31 and displacement of adjacent duplex strands during translocation, as shown for the viral SF2 DExH helicases including NS3Citation32 and NPH-II.Citation32,Citation33 In contrast to these bona fide helicases, DRAs preferentially bind RNA duplex instead of single stranded regions,Citation16,Citation34,Citation35 and in all existing structures of RNA-RIG-I complexes, the duplex RNA maintains an undistorted A-form conformation.Citation17-Citation19,Citation36 Furthermore, the crucial β-hairpin motif that participates in strand separation by DExH helicases is missing in RIG-I and other DRAs.Citation17,Citation37,Citation38 Therefore, because DRAs have no structural features designed to disrupt duplex RNA, and no structural motifs for coupling translocation with strand separation, it is not surprising that DRAs have not yet been shown to function as unwindases.
The Molecular and Structural Biology of DRAs
Structural studies on nucleic acid-dependent ATPases are hampered by the intrinsic flexibility that arises from their function as molecular motors. In these proteins, the two conserved Rec-A like domains are loosely connected in the absence of nucleic acid and ATP. This is particularly true for DEAD box proteins, which tend to be captured crystallographically only in the presence of both ssRNA and ATP analogs,Citation39-Citation43 thereby limiting our understanding of their functional cycles. Structural studies of DRAs face the same challenges as those focused on DEAD box proteins. Adding to this difficulty, DRAs are large multi-domain proteins with several moving parts that usually function within even larger protein complexes. Nevertheless, recent cryo-electron microscopy studies of DicerCitation44-Citation46 and crystallographic studies on RIG-I have advanced our understanding of the biological function and mechanical properties of DRA proteins.Citation15,Citation17-Citation19
Dicer: the small RNA processing machine
Ever since Fire et al., published the groundbreaking paper on RNA interference (RNAi),Citation47 great strides have been made in understanding the biogenesis and functional mechanisms of the small RNAs that facilitate dsRNA-mediated gene regulation.Citation1,Citation48-Citation53 There are two major types of small RNAs: microRNA (miRNA) and small interfering RNA (siRNA). While these two RNAs differ in their pathway of biogenesis, they share similarities in function. Of central importance to the RNAi pathway is the formation of an RNA-induced silencing complex (RISC),Citation54,Citation55 which binds to the target mRNA and results in downregulation of gene expression by either RNA degradation or translational arrest.Citation1,Citation52 RISC is assembled from the RISC-loading complex (RLC), which includes dsRNA, Ago, Dicer and other additional dsRNA binding and accessory proteins.Citation56,Citation57
Dicer plays a major role in gene regulation by processing dsRNA precursors into short fragments that are used to target the silencing of specific genes.Citation58-Citation61 Dicer cleaves long duplex precursor RNAs (pre-miRNA or pre-siRNA) into short miRNA and siRNA fragments and then loads the correct “guide” strand into RISC. Dicer received its name because of its dsRNA cleavage, or “dicing” activity.Citation58 Phylogenetically, Dicer is a class III RNase, members of which are conserved among eukaryotic species.Citation62 In humans, there is only one Dicer protein, hsDicer1, however Drosophila and plants contain two and four Dicer proteins respectively. Human Dicer1 mutations have been found in various cancer syndromes,Citation63-Citation65 emphasizing its fundamental roles in gene regulation. In general, all Dicer and Dicer like proteins (DCL) from eukaryotic species share a similar domain architecture, containing a SF2 RNA-dependent ATPase domain at the N terminus, a DUF283 domain (Domain of Unknown Function), a PAZ domain, two tandem RNase III domains and a dsRNA-binding domain (dsRBD) at the C terminusCitation14,Citation44(). The first structural insights into Dicer components came from a crystal structure of a Dicer homolog obtained from the unicellular eukaryote Giardia intestinalis. This structure revealed a specific spatial arrangement of the PAZ domain relative to the two RNaseIII domains,Citation66 suggesting that Dicer contains a molecular ruler that enables it to generate dsRNA fragments of specific length. Unfortunately, unlike Dicer genes from other organisms, Giardia intestinalis Dicer does not contain an ATPase motor domain.
The ATPase motor domain of Dicer is highly conserved across species and it is phylogenetically distinguishable as a DRA protein (). The precise biochemical function of the motor domain is still unclear, and it is not yet known whether the RNA-dependent ATPase activity is actually linked to duplex unwinding, and whether the motor domain behaves like a helicase. Recent studies have indicated that it plays a role in helping to select the “guide strand” from the two duplex strands that are initially bound within the RISC-loading complex. This is accomplished by sensing thermodynamic features of the RNA duplex, and determining which terminus is more easily opened.Citation67 The selected siRNA guide strand will then be loaded into the Ago protein, resulting in formation of a functional RISC complex.Citation67
The overall three-dimensional architecture and domain organization of Dicer is well conserved among orthologs.Citation14,Citation44 Dicer adopts an L-shape as determined by negative-stain electron microscopy (EM).Citation44 Using a streptavidin tagging method and domain deletion constructs, Lau et al. accurately located the position of the motor domain at the base of the L shaped structure (). Furthermore, when the motor domain of the RIG-I was docked into the EM structure of Dicer, the RNA binding interfaces of the motor domain and the RNase III domain creates an adjacent central RNA binding groove.Citation44 A complex between Dicer and its TRBP (TAR RNA Binding Protein, an accessory protein of Dicer and a dsRBD) forms a similar L shape with a long edge of 150 Ǻ and a 100 Ǻ extension at the bottom end.Citation45 Because of the small size and intrinsic flexibility of TRBP, it is difficult to accurately assign its location, particularly in the absence of a siRNA or miRNA substrate. A low resolution EM structure of the human RISC-loading complex (containing Dicer, AGO2 and TRBP in a 1:1:1 stoichiometric ratio) was obtained by crossing-linking the complex. In the resulting model, AGO2 was proposed to interact with the C-terminal region of Dicer.Citation68 The RNA binding site of AGO2 is located in close proximity to the C-terminal region of Dicer, pointing away from the N-terminal motor domain. This model is consistent with biochemical data suggesting that the motor domain of Dicer may not be required for loading mature siRNA into the AGO2.Citation69 Two discrete conformations of the Dicer motor domain have been identified, suggesting that it may adopt multiple conformations on dsRNACitation44. This structural flexibility contributes to specific dsRNA recognition and may support a processive dicing mechanism ().Citation35,Citation70,Citation71 Not surprisingly, it is similar to structural rearrangements observed when the RIG-I motor domain binds to RNA duplex.Citation18,Citation19
Figure 3. (A) Segmented map of human Dicer with crystal structures of homologous domains docked. (B) Model for pre-miRNA recognition. A pre-miRNA hairpin is modeled into the proposed binding channel of Dicer, with the stem-loop fit in the RNA-binding cleft of the protein. (C) Schematic for processive dicing in which dsRNA is translocated into the nuclease core (1). The PAZ domain (purple) recognizes the dsRNA end, positioning RNase III (orange) for cleavage (2). The siRNA product is released while the dsRNA substrate remains bound to the protein (3). Reprinted with permission from Lau et al.Citation44
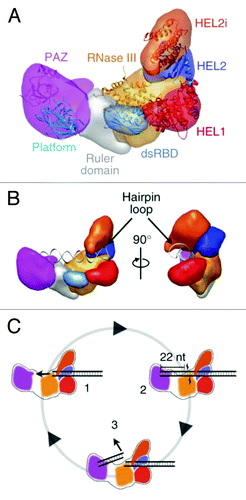
RIG-I: The innate immune sensor for viral RNA detection and defense
A diverse group of cytoplasmic surveillance proteins sensitively detect the presence of viral genomes and gene products and then initiate inflammatory responses that enable vertebrates to fight viral infections.Citation72,Citation73 These proteins form the foundation of our innate immune response. The RIG-I-like receptors (RLRs) are a specialized subclass of DRA proteins that detect double stranded viral RNAs in the cytoplasm and initiate a series of signaling events to elicit an antiviral response. The RLR motor proteins include RIG-I,Citation74 MDA5 (Melanoma Differentiation Associated gene 5)Citation34 and LGP2 (Laboratory of Genetics and Physiology 2).Citation75 They were initially identified in different biological contexts and were later re-discovered to be key members in antiviral innate immunity.Citation72,Citation73,Citation76 RIG-I is the most extensively studied member of the RLRs and has been demonstrated to be the major antiviral RLR. RIG-I recognizes a broad range of viruses, including negative stranded viruses, e.g., vesicular stomatitis virus, Sendai virus, influenza virus and rabies virus; positive stranded viruses such as dengue virus, Japanese encephalitis virus, West Nile virus and Hepatitis C virus; dsRNA virus (reovirus) and DNA virus (Epstein-Barr virus).Citation3,Citation77 MDA5 is both structurally and functionally similar to RIG-I and complements RIG-I by recognizing a distinct set of virus RNAs although there might be some overlap.Citation3 LGP2 is thought to serve as a feedback regulator but its exact function is still not clearly defined.Citation78,Citation79
RIG-I contains two tandem caspase activation and recruitment domains (CARDs; CARD1 and CARD2) at its N-terminus, which mediate a downstream signaling relay; a central DRA motor domain, and a C-terminal domain (CTD) that facilitates viral RNA recognition ().Citation15,Citation17-Citation19,Citation80 It is commonly believed that RIG-I is inactive in resting cells and it is activated upon detection and binding of viral RNA. The activated RIG-I is believed to hydrolyze ATP and initiate a signaling cascade and type I interferon (IFN) response via the adaptor protein MAVS, also known as IPS-1, VISA or CARDIF.Citation3 MAVS in turn activates several transcription factors including IRF3, IRF7 and NF-κB, and leads to the production of IFN and inflammatory cytokines.Citation77,Citation80,Citation81 Moreover, RIG-I displays apoptosis-inducing properties in tumor cells.Citation82,Citation83 Effective therapeutic RIG-I antagonists and agonists may provide new tools for the treatment of viral infections and cancer.Citation84
Recent research has focused on characterizing the molecular determinants for RNA-RIG-I recognition, the mechanisms of activation and signaling, and regulatory pathways that help control RIG-I signaling. Structural and biochemical studies on RIG-I have revealed that 5′ tri-phosphorylated blunt-ended duplex RNAs are the optimal substrate for RIG-I binding and activation.Citation17-Citation19,Citation73,Citation85,Citation86 The exact length of the duplex is unclear although it is generally accepted that RIG-I recognizes RNA that is ten to hundreds of base pairs in length, while MDA5 forms filaments on longer RNA in the thousands of base pairs.Citation85,Citation87-Citation90 The CTD is primarily responsible for 5′tri-phosphate recognition and both the CTD and helicase domain form critical contacts with the RNA duplex.Citation15,Citation18,Citation19,Citation85,Citation91-Citation93 The latest structural studies indicate RNA binding induces a dramatic conformational change in RIG-I ( and B). The role of ATP binding and hydrolysis has not been determined, although mutations in the ATPase domain are clearly deleterious to function.Citation15,Citation18,Citation19 Post translational modifications of RIG-I, including ubiquitination, phosphorylation and SUMOylation, have been reported to be important for its function.Citation94-Citation96 Non-covalent polyubiquitin binding to the CARDs is likely to be essential for full activation of RIG-I and possibly oligomerization.Citation97,Citation98
Figure 4. Structural basis for dsRNA recognition and activation of RLRs. Models were created by aligning and merging known duck and human RIG-I structures and by taking consideration of our recent solution hydrodynamic studies on RIG-I conformational dynamics upon RNA and ATP binding.Citation36 (A) Model of full length RIG-I apoenzyme based on structures of duck RIG-I (PDB: 4A2W) and the CTD (PDB: 4A2V). In the autoinhibited conformation, the N-terminal CARDs are sequestered from signaling and maintain RIG-I in an autoinhibited state. (B) RIG-I switches into a semi-closed conformation upon RNA binding. Binding of dsRNA to the CTD brings the HEL domains in contact with dsRNA (PDB: 4A2Wand 2YKG). (C) ATP binding (yellow) closes the HEL domains that would cause a clash between the CARDs and CTD (PDB: 4A2W, 4A36 and 2YKG). (D) The change in conformation upon dsRNA and ATP binding releases the CARD domains for signaling.
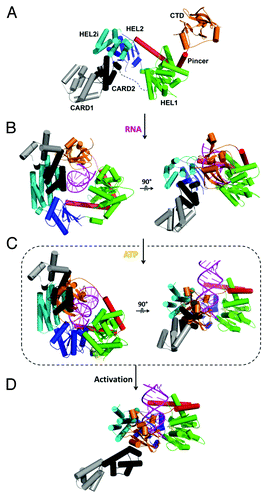
In the resting state, RIG-I adopts an autoinhibited conformation in which the motor domain is sterically blocked.Citation15,Citation18 The CARDs are trapped in a fixed conformation relative to the HEL domains (synonymous with Rec-A folds 1 and 2) through an interaction between the second CARD and the insertion domain (HEL2i) ().Citation18 This conformation was speculated to inhibit both CARD1 ubiquitination by the ubiquitin E3 ligase TRIM25 and non-covalent binding of polyubiquitin to the CARDs, both of which are required for RIG-I activation. In the apoenzyme state the RNA binding surfaces of RIG-I (and particularly the CTD) are largely exposed, allowing RIG-I to search for viral RNAs. The CTD, which is connected to the HEL domain through a long and flexible pincer domain, enhances the specificity of RIG-I for tri-phosphorylated RNA.Citation18
It is believed that viral activation of RIG-I signaling occurs in a carefully choreographed sequence of events. Binding of viral RNA is the initial trigger for RIG-I activation, whereupon the motor domain (comprised of HEL1, HEL2and HEL2i) of RIG-I forms a ring-shaped clamp around the sugar-phosphate backbone of the duplex and the CTD caps the helical terminus, even in the absence of a 5′ triphosphate. The tight and specific interaction of the CTD with the duplex terminus may prevent RIG-I from binding with high affinity to internal sites on the duplexCitation17-Citation19(). Structural analysis suggests that binding of RIG-I to RNA alone may not be sufficient to disrupt the autoinhibitory interaction between the CARD2 and HEL2i domains,Citation19 hinting that an additional trigger might be needed to activate signaling. In the crystal structures of RIG-I:dsRNA with AlFx and BeFx, ATP binding appears to bring the RIG-I helicase into a more closed and compact conformation relative to RIG-I structures that contain only dsRNA ().Citation17,Citation18,Citation36 This ATP-induced conformational change shifts the CTD and HEL2i toward each other, resulting in a clash between the CARDs and CTD (). Consequently the structure is likely to reorganize, reorienting the relative positions of the CARDs and HEL2i, and potentially releasing the CARDs which makes them available for interaction with MAVS and activates the innate immune response ().Citation97,Citation98
In agreement with this idea, ATP is required for in vitro reconstitution of the RIG-I signaling pathway,Citation97,Citation99 although ATP hydrolysis and turnover is not essential.Citation100 Activation of RIG-I is therefore a tightly-regulated, multi-checkpoint process, starting with recognition of the correct RNA substrate, followed by ATP binding, and then subsequent coupled structural rearrangements that release auto-inhibition and switch RIG-I into a signaling-competent state ().Citation15,Citation101
DRH-3: Attenuating the siRNA pathway in Caenorhabditis elegans
A group of endogenous siRNAs, named 22G endo-siRNA, from Caenorhabditis elegans are linked to a variety of biological processes that are vital to maintaining genetic stability, including transposon silencing and chromosome segregation in germline cells.Citation102-Citation104 Defects in the endo-RNAi pathway can result in many forms of genetic instability such as loss of chromosomes during mitosis, abnormal gene expression and increased sensitivity to X-ray irradiation.Citation102-Citation105 These siRNAs are classified as secondary siRNA molecules because they are produced directly by RNA-dependent RNA polymerase (RdRP) transcription, without a double-stranded RNA intermediate or cleavage.Citation106,Citation107
Dicer-related helicase 3 (DRH-3) is a large multi-domain, multi-functional protein that is essential for the biogenesis of these endogenous secondary siRNAs.Citation12,Citation13,Citation103,Citation104 DRH-3 interacts with members of the C. elegans RNAi machinery, including Dicer (DCR-1) and the RdRP, RRF-1.Citation12,Citation13 A large protein (1119 amino acids, ca. 130 kDa), DRH-3 contains 3 sub-domains (). These include an N-terminal domain of novel sequence that lacks a known homolog, and the central motor domain that is common to DRA proteins.Citation5,Citation10,Citation108 The C-terminal domain of DRH-3 shares sequence similarity with the CTD that plays an important role in RNA and triphosphate recognition by RIG-I. Although there are no structural studies of DRH-3, a preliminary biochemical characterization has been reported. Several key features of DRH-3 are now apparent: the protein binds more strongly to dsRNA than to ssRNA; potent ATP hydrolysis by DRH-3 is only stimulated by dsRNA; DRH-3 does not have unwinding activity.Citation16 DRH-1, a homolog of DRH-3, is implicated in both germline and somatic RNA interference (RNAi) pathways as well as virus sensing and viral siRNA formationCitation109,Citation110 and may be an equally important target for future biochemical and structural studies.
DRH-3 has a domain organization that is very similar to RIG-I. It is tempting to speculate that DRH-3 might bind to the RNA duplexes generated by the endogenous siRNA pathway and recruit signaling partners through its NTD. This speculation is further supported by the absence of helicase activity and the preference for canonical RNA duplex binding.Citation16
Concluding Remarks and Future Directions for Research on DRA Proteins
DRAs share several characteristic features that distinguish them from other groups of RNA-dependent ATPases. First, in addition to the conserved motifs that classify DRAs as SF2 RNA-dependent ATPases, DRAs contain unique motifs (e.g., motifs IIa and Vc) and domains (HEL2i) that specialize in duplex RNA recognition. Second, although the literature on DRAs is somewhat unclear on this point, DRAs do not appear to possess RNA unwinding activity and they may accomplish their biological function by simply binding duplex RNA or by translocating along the duplex without unwinding. Lastly, the DRAs discussed in this review are all part of larger protein complexes that function in duplex RNA sensing and processing.
One of the most intriguing questions about DRAs is whether they, in fact, require ATP hydrolysis for function. At the present time, it is not established that DRAs require ATP binding and/or hydrolysis and, like DEAD-box proteins, they may only utilize ATP for recycling. ATPase activity is unnecessary for pre-miRNA processing by human Dicer,Citation35,Citation69,Citation111 but in contrast, Drosophila Dicer-2 appears to require ATP for siRNA production.Citation55,Citation112 The ATPase motor domain from C. elegans DCR is required for the biogenesis of some but not all siRNAs.Citation113 Evidently, there is no consensus for the function of ATP hydrolysis by Dicers from different species. RIG-I has shown a clear dependence for ATP in in vitro reconstitution systems,Citation97,Citation98 but mutagenesis studies by Bamming and Horvath suggest that signaling by RIG-I and MDA5 can occur independent of ATPase enzymatic activity.Citation100 To reconcile this, recent structural data suggests that ATP binding but not necessarily hydrolysis induces a conformational change on the RIG-I helicase domain that may eventually lead to RIG-I activation (). Further experiments are needed to verify this structure driven hypothesis.
Although the recent crystal structures of RIG-I advance our understanding of DRAs, there are several questions that remain unanswered. One future question is whether DRAs recognize specific RNA sequences or structures. Several studies suggest that Dicer recognizes specific terminal structures of RNA as evidenced by the fact that human dicer is more efficient in processing pre-miRNA than siRNA.Citation71 Furthermore, Drosophila Dicer-1 recognizes the terminal loop structure of pre-miRNAs through its motor domain,Citation70 and both Drosophila Dicer-2 and C. elegans DCR-1 differentiate the end structures of long duplex RNAs for endo-siRNA processing.Citation114 This suggests there might be a special structural feature in the Dicer RNA-dependent ATPase domain that is responsible for recognizing RNA ends. RIG-I specifically recognizes tri-phosphorylated RNA through its accessory CTD domain, and given that RIG-I recognizes a broad but distinct set of RNA viruses, it will be interesting to determine if RIG-I can recognize unique viral RNA sequences or structures, in addition to 5′ triphosphate and duplex RNA.
Oligomerization is a variable characteristic that can be important for the function of SF2 proteins, including DRAs. To generalize, the minimum functional unit of SF2 proteins is monomeric, but there is biochemical evidence suggesting that oligomerization can enhance biological activity of certain SF2 proteins.Citation115,Citation116 As for DRAs, there is no indication that Dicer forms oligomers, however RIG-I has been proposed to dimerizeCitation93 or tetramerizeCitation97 upon activation. The exact molecular basis for RIG-I oligomerization is still unknown; however the downstream target of RIG-I, MAVS, forms filaments upon activation. Distinct from RIG-I, MDA5 cooperatively binds to long duplex RNAs and may form a filament-like structure itself,Citation115,Citation117 which would be unique not only among DRAs but also SF2 proteins in general. It is therefore important to establish whether oligomerization obligatory for RIG-I or MDA5 function.
Given the central role of DRA proteins in diverse cellular pathways that range from epigenetic regulation to the innate immune response, it will be interesting to characterize the regulatory cofactors that help to specify and control the activity of DRAs. It will also be exciting to identify new DRA proteins that have distinct molecular functions. The DRA proteins seem to be markers of interesting biology, and investigation of this protein family will continue to yield major insights into the nanomechanical features of living systems.
Acknowledgments
We apologize to all colleagues whose contributions could not be highlighted or discussed within this manuscript. We thank David Rawling for reading the manuscript. This research was funded by Howard Hughes Medical Institute. D.L. is a postdoctoral associate and A.M.P. is an investigator with the Howard Hughes Medical Institute.
Disclosure of Potential Conflicts of Interest
No potential conflicts of interest were disclosed.
References
- Ketting RF. The many faces of RNAi. Dev Cell 2011; 20:148 - 61; http://dx.doi.org/10.1016/j.devcel.2011.01.012; PMID: 21316584
- Ghildiyal M, Zamore PD. Small silencing RNAs: an expanding universe. Nat Rev Genet 2009; 10:94 - 108; http://dx.doi.org/10.1038/nrg2504; PMID: 19148191
- Ramos HJ, Gale M Jr.. RIG-I like receptors and their signaling crosstalk in the regulation of antiviral immunity. Curr Opin Virol 2011; 1:167 - 76; http://dx.doi.org/10.1016/j.coviro.2011.04.004; PMID: 21949557
- Fairman-Williams ME, Guenther UP, Jankowsky E. SF1 and SF2 helicases: family matters. Curr Opin Struct Biol 2010; 20:313 - 24; http://dx.doi.org/10.1016/j.sbi.2010.03.011; PMID: 20456941
- Pyle AM. Translocation and unwinding mechanisms of RNA and DNA helicases. Annu Rev Biophys 2008; 37:317 - 36; http://dx.doi.org/10.1146/annurev.biophys.37.032807.125908; PMID: 18573084
- Gorbalenya AE, Koonin EV. Helicases: amino acid sequence comparisons and structure-function relationships. Curr Opin Struct Biol 1993; 3:419 - 29; http://dx.doi.org/10.1016/S0959-440X(05)80116-2
- Tanner NK, Linder P. DExD/H box RNA helicases: from generic motors to specific dissociation functions. Mol Cell 2001; 8:251 - 62; http://dx.doi.org/10.1016/S1097-2765(01)00329-X; PMID: 11545728
- Singleton MR, Dillingham MS, Wigley DB. Structure and mechanism of helicases and nucleic acid translocases. Annu Rev Biochem 2007; 76:23 - 50; http://dx.doi.org/10.1146/annurev.biochem.76.052305.115300; PMID: 17506634
- Ranji A, Boris-Lawrie K. RNA helicases: emerging roles in viral replication and the host innate response. RNA Biol 2010; 7:775 - 87; http://dx.doi.org/10.4161/rna.7.6.14249; PMID: 21173576
- Jankowsky E, Fairman ME. RNA helicases--one fold for many functions. Curr Opin Struct Biol 2007; 17:316 - 24; http://dx.doi.org/10.1016/j.sbi.2007.05.007; PMID: 17574830
- Steimer L, Klostermeier D. RNA helicases in infection and disease. RNA Biol 2012; 9:751 - 71; http://dx.doi.org/10.4161/rna.20090; PMID: 22699555
- Aoki K, Moriguchi H, Yoshioka T, Okawa K, Tabara H. In vitro analyses of the production and activity of secondary small interfering RNAs in C. elegans. EMBO J 2007; 26:5007 - 19; http://dx.doi.org/10.1038/sj.emboj.7601910; PMID: 18007599
- Duchaine TF, Wohlschlegel JA, Kennedy S, Bei Y, Conte D Jr., Pang K, et al. Functional proteomics reveals the biochemical niche of C. elegans DCR-1 in multiple small-RNA-mediated pathways. Cell 2006; 124:343 - 54; http://dx.doi.org/10.1016/j.cell.2005.11.036; PMID: 16439208
- Jinek M, Doudna JA. A three-dimensional view of the molecular machinery of RNA interference. Nature 2009; 457:405 - 12; http://dx.doi.org/10.1038/nature07755; PMID: 19158786
- Jiang QX, Chen ZJ. Structural insights into the activation of RIG-I, a nanosensor for viral RNAs. EMBO Rep 2012; 13:7 - 8; http://dx.doi.org/10.1038/embor.2011.239; PMID: 22157887
- Matranga C, Pyle AM. Double-stranded RNA-dependent ATPase DRH-3: insight into its role in RNAsilencing in Caenorhabditis elegans. J Biol Chem 2010; 285:25363 - 71; http://dx.doi.org/10.1074/jbc.M110.117010; PMID: 20529861
- Jiang F, Ramanathan A, Miller MT, Tang GQ, Gale M Jr., Patel SS, et al. Structural basis of RNA recognition and activation by innate immune receptor RIG-I. Nature 2011; 479:423 - 7; http://dx.doi.org/10.1038/nature10537; PMID: 21947008
- Kowalinski E, Lunardi T, McCarthy AA, Louber J, Brunel J, Grigorov B, et al. Structural basis for the activation of innate immune pattern-recognition receptor RIG-I by viral RNA. Cell 2011; 147:423 - 35; http://dx.doi.org/10.1016/j.cell.2011.09.039; PMID: 22000019
- Luo D, Ding SC, Vela A, Kohlway A, Lindenbach BD, Pyle AM. Structural insights into RNA recognition by RIG-I. Cell 2011; 147:409 - 22; http://dx.doi.org/10.1016/j.cell.2011.09.023; PMID: 22000018
- Sarkar D, Desalle R, Fisher PB. Evolution of MDA-5/RIG-I-dependent innate immunity: independent evolution by domain grafting. Proc Natl Acad Sci U S A 2008; 105:17040 - 5; http://dx.doi.org/10.1073/pnas.0804956105; PMID: 18971330
- Zou J, Chang M, Nie P, Secombes CJ. Origin and evolution of the RIG-I like RNA helicase gene family. BMC Evol Biol 2009; 9:85; http://dx.doi.org/10.1186/1471-2148-9-85; PMID: 19400936
- Whitby MC. The FANCM family of DNA helicases/translocases. DNA Repair (Amst) 2010; 9:224 - 36; http://dx.doi.org/10.1016/j.dnarep.2009.12.012; PMID: 20117061
- Dürr H, Körner C, Müller M, Hickmann V, Hopfner KP. X-ray structures of the Sulfolobus solfataricus SWI2/SNF2 ATPase core and its complex with DNA. Cell 2005; 121:363 - 73; http://dx.doi.org/10.1016/j.cell.2005.03.026; PMID: 15882619
- Thomä NH, Czyzewski BK, Alexeev AA, Mazin AV, Kowalczykowski SC, Pavletich NP. Structure of the SWI2/SNF2 chromatin-remodeling domain of eukaryotic Rad54. Nat Struct Mol Biol 2005; 12:350 - 6; http://dx.doi.org/10.1038/nsmb919; PMID: 15806108
- Hauk G, Bowman GD. Structural insights into regulation and action of SWI2/SNF2 ATPases. Curr Opin Struct Biol 2011; 21:719 - 27; http://dx.doi.org/10.1016/j.sbi.2011.09.003; PMID: 21996440
- Mahdi AA, Briggs GS, Sharples GJ, Wen Q, Lloyd RG. A model for dsDNA translocation revealed by a structural motif common to RecG and Mfd proteins. EMBO J 2003; 22:724 - 34; http://dx.doi.org/10.1093/emboj/cdg043; PMID: 12554672
- Luo D, Wei N, Doan DN, Paradkar PN, Chong Y, Davidson AD, et al. Flexibility between the protease and helicase domains of the dengue virus NS3 protein conferred by the linker region and its functional implications. J Biol Chem 2010; 285:18817 - 27; http://dx.doi.org/10.1074/jbc.M109.090936; PMID: 20375022
- Murray NE. Type I restriction systems: sophisticated molecular machines (a legacy of Bertani and Weigle). Microbiol Mol Biol Rev 2000; 64:412 - 34; http://dx.doi.org/10.1128/MMBR.64.2.412-434.2000; PMID: 10839821
- Lapkouski M, Panjikar S, Janscak P, Smatanova IK, Carey J, Ettrich R, et al. Structure of the motor subunit of type I restriction-modification complex EcoR124I. Nat Struct Mol Biol 2009; 16:94 - 5; http://dx.doi.org/10.1038/nsmb.1523; PMID: 19079266
- Mallam AL, Del Campo M, Gilman B, Sidote DJ, Lambowitz AM. Structural basis for RNA-duplex recognition and unwinding by the DEAD-box helicase Mss116p. Nature 2012; 490:121 - 5; http://dx.doi.org/10.1038/nature11402; PMID: 22940866
- Yang Q, Del Campo M, Lambowitz AM, Jankowsky E. DEAD-box proteins unwind duplexes by local strand separation. Mol Cell 2007; 28:253 - 63; http://dx.doi.org/10.1016/j.molcel.2007.08.016; PMID: 17964264
- Pang PS, Jankowsky E, Planet PJ, Pyle AM. The hepatitis C viral NS3 protein is a processive DNA helicase with cofactor enhanced RNA unwinding. EMBO J 2002; 21:1168 - 76; http://dx.doi.org/10.1093/emboj/21.5.1168; PMID: 11867545
- Jankowsky E, Gross CH, Shuman S, Pyle AM. The DExH protein NPH-II is a processive and directional motor for unwinding RNA. Nature 2000; 403:447 - 51; http://dx.doi.org/10.1038/35000239; PMID: 10667799
- Kang DC, Gopalkrishnan RV, Wu Q, Jankowsky E, Pyle AM, Fisher PB. mda-5: An interferon-inducible putative RNA helicase with double-stranded RNA-dependent ATPase activity and melanoma growth-suppressive properties. Proc Natl Acad Sci U S A 2002; 99:637 - 42; http://dx.doi.org/10.1073/pnas.022637199; PMID: 11805321
- Zhang H, Kolb FA, Brondani V, Billy E, Filipowicz W. Human Dicer preferentially cleaves dsRNAs at their termini without a requirement for ATP. EMBO J 2002; 21:5875 - 85; http://dx.doi.org/10.1093/emboj/cdf582; PMID: 12411505
- Luo D, Kohlway A, Vela A, Pyle AM. Visualizing the Determinants of Viral RNA Recognition by Innate Immune Sensor RIG-I. Structure 2012; In Press http://dx.doi.org/10.1016/j.str.2012.08.029; PMID: 23022350
- Luo D, Xu T, Watson RP, Scherer-Becker D, Sampath A, Jahnke W, et al. Insights into RNA unwinding and ATP hydrolysis by the flavivirus NS3 protein. EMBO J 2008; 27:3209 - 19; http://dx.doi.org/10.1038/emboj.2008.232; PMID: 19008861
- Büttner K, Nehring S, Hopfner KP. Structural basis for DNA duplex separation by a superfamily-2 helicase. Nat Struct Mol Biol 2007; 14:647 - 52; http://dx.doi.org/10.1038/nsmb1246; PMID: 17558417
- Sengoku T, Nureki O, Nakamura A, Kobayashi S, Yokoyama S. Structural basis for RNA unwinding by the DEAD-box protein Drosophila Vasa. Cell 2006; 125:287 - 300; http://dx.doi.org/10.1016/j.cell.2006.01.054; PMID: 16630817
- Bono F, Ebert J, Lorentzen E, Conti E. The crystal structure of the exon junction complex reveals how it maintains a stable grip on mRNA. Cell 2006; 126:713 - 25; http://dx.doi.org/10.1016/j.cell.2006.08.006; PMID: 16923391
- Andersen CB, Ballut L, Johansen JS, Chamieh H, Nielsen KH, Oliveira CL, et al. Structure of the exon junction core complex with a trapped DEAD-box ATPase bound to RNA. Science 2006; 313:1968 - 72; http://dx.doi.org/10.1126/science.1131981; PMID: 16931718
- Del Campo M, Lambowitz AM. Structure of the Yeast DEAD box protein Mss116p reveals two wedges that crimp RNA. Mol Cell 2009; 35:598 - 609; http://dx.doi.org/10.1016/j.molcel.2009.07.032; PMID: 19748356
- Collins R, Karlberg T, Lehtiö L, Schütz P, van den Berg S, Dahlgren LG, et al. The DEXD/H-box RNA helicase DDX19 is regulated by an alpha-helical switch. J Biol Chem 2009; 284:10296 - 300; http://dx.doi.org/10.1074/jbc.C900018200; PMID: 19244245
- Lau PW, Guiley KZ, De N, Potter CS, Carragher B, MacRae IJ. The molecular architecture of human Dicer. Nat Struct Mol Biol 2012; 19:436 - 40; http://dx.doi.org/10.1038/nsmb.2268; PMID: 22426548
- Lau PW, Potter CS, Carragher B, MacRae IJ. Structure of the human Dicer-TRBP complex by electron microscopy. Structure 2009; 17:1326 - 32; http://dx.doi.org/10.1016/j.str.2009.08.013; PMID: 19836333
- Wang Y, Sheng G, Juranek S, Tuschl T, Patel DJ. Structure of the guide-strand-containing argonaute silencing complex. Nature 2008; 456:209 - 13; http://dx.doi.org/10.1038/nature07315; PMID: 18754009
- Fire A, Xu S, Montgomery MK, Kostas SA, Driver SE, Mello CC. Potent and specific genetic interference by double-stranded RNA in Caenorhabditis elegans. Nature 1998; 391:806 - 11; http://dx.doi.org/10.1038/35888; PMID: 9486653
- Faehnle CR, Joshua-Tor L. Argonautes confront new small RNAs. Curr Opin Chem Biol 2007; 11:569 - 77; http://dx.doi.org/10.1016/j.cbpa.2007.08.032; PMID: 17928262
- Tolia NH, Joshua-Tor L. Slicer and the argonautes. Nat Chem Biol 2007; 3:36 - 43; http://dx.doi.org/10.1038/nchembio848; PMID: 17173028
- Shabalina SA, Koonin EV. Origins and evolution of eukaryotic RNA interference. Trends Ecol Evol 2008; 23:578 - 87; http://dx.doi.org/10.1016/j.tree.2008.06.005; PMID: 18715673
- Carthew RW, Sontheimer EJ. Origins and Mechanisms of miRNAs and siRNAs. Cell 2009; 136:642 - 55; http://dx.doi.org/10.1016/j.cell.2009.01.035; PMID: 19239886
- Liu Q, Paroo Z. Biochemical principles of small RNA pathways. Annu Rev Biochem 2010; 79:295 - 319; http://dx.doi.org/10.1146/annurev.biochem.052208.151733; PMID: 20205586
- Sashital DG, Doudna JA. Structural insights into RNA interference. Curr Opin Struct Biol 2010; 20:90 - 7; http://dx.doi.org/10.1016/j.sbi.2009.12.001; PMID: 20053548
- Hammond SM, Bernstein E, Beach D, Hannon GJ. An RNA-directed nuclease mediates post-transcriptional gene silencing in Drosophila cells. Nature 2000; 404:293 - 6; http://dx.doi.org/10.1038/35005107; PMID: 10749213
- Zamore PD, Tuschl T, Sharp PA, Bartel DP. RNAi: double-stranded RNA directs the ATP-dependent cleavage of mRNA at 21 to 23 nucleotide intervals. Cell 2000; 101:25 - 33; http://dx.doi.org/10.1016/S0092-8674(00)80620-0; PMID: 10778853
- Iwasaki S, Kobayashi M, Yoda M, Sakaguchi Y, Katsuma S, Suzuki T, et al. Hsc70/Hsp90 chaperone machinery mediates ATP-dependent RISC loading of small RNA duplexes. Mol Cell 2010; 39:292 - 9; http://dx.doi.org/10.1016/j.molcel.2010.05.015; PMID: 20605501
- Miyoshi T, Takeuchi A, Siomi H, Siomi MC. A direct role for Hsp90 in pre-RISC formation in Drosophila. Nat Struct Mol Biol 2010; 17:1024 - 6; http://dx.doi.org/10.1038/nsmb.1875; PMID: 20639883
- Bernstein E, Caudy AA, Hammond SM, Hannon GJ. Role for a bidentate ribonuclease in the initiation step of RNA interference. Nature 2001; 409:363 - 6; http://dx.doi.org/10.1038/35053110; PMID: 11201747
- Ketting RF, Fischer SE, Bernstein E, Sijen T, Hannon GJ, Plasterk RH. Dicer functions in RNA interference and in synthesis of small RNA involved in developmental timing in C. elegans. Genes Dev 2001; 15:2654 - 9; http://dx.doi.org/10.1101/gad.927801; PMID: 11641272
- Knight SW, Bass BL. A role for the RNase III enzyme DCR-1 in RNA interference and germ line development in Caenorhabditis elegans. Science 2001; 293:2269 - 71; http://dx.doi.org/10.1126/science.1062039; PMID: 11486053
- Xie Z, Johansen LK, Gustafson AM, Kasschau KD, Lellis AD, Zilberman D, et al. Genetic and functional diversification of small RNA pathways in plants. PLoS Biol 2004; 2:E104; http://dx.doi.org/10.1371/journal.pbio.0020104; PMID: 15024409
- Liu Q, Feng Y, Zhu Z. Dicer-like (DCL) proteins in plants. Funct Integr Genomics 2009; 9:277 - 86; http://dx.doi.org/10.1007/s10142-009-0111-5; PMID: 19221817
- Doros L, Yang J, Dehner L, Rossi CT, Skiver K, Jarzembowski JA, et al. DICER1 mutations in embryonal rhabdomyosarcomas from children with and without familial PPB-tumor predisposition syndrome. Pediatr Blood Cancer 2012; 59:558 - 60; PMID: 22180160
- Foulkes WD, Bahubeshi A, Hamel N, Pasini B, Asioli S, Baynam G, et al. Extending the phenotypes associated with DICER1 mutations. Hum Mutat 2011; 32:1381 - 4; http://dx.doi.org/10.1002/humu.21600; PMID: 21882293
- Hill DA, Ivanovich J, Priest JR, Gurnett CA, Dehner LP, Desruisseau D, et al. DICER1 mutations in familial pleuropulmonary blastoma. Science 2009; 325:965; http://dx.doi.org/10.1126/science.1174334; PMID: 19556464
- Macrae IJ, Zhou K, Li F, Repic A, Brooks AN, Cande WZ, et al. Structural basis for double-stranded RNA processing by Dicer. Science 2006; 311:195 - 8; http://dx.doi.org/10.1126/science.1121638; PMID: 16410517
- Noland CL, Ma E, Doudna JA. siRNA repositioning for guide strand selection by human Dicer complexes. Mol Cell 2011; 43:110 - 21; http://dx.doi.org/10.1016/j.molcel.2011.05.028; PMID: 21726814
- Wang HW, Noland C, Siridechadilok B, Taylor DW, Ma E, Felderer K, et al. Structural insights into RNA processing by the human RISC-loading complex. Nat Struct Mol Biol 2009; 16:1148 - 53; http://dx.doi.org/10.1038/nsmb.1673; PMID: 19820710
- Betancur JG, Tomari Y. Dicer is dispensable for asymmetric RISC loading in mammals. RNA 2012; 18:24 - 30; http://dx.doi.org/10.1261/rna.029785.111; PMID: 22106413
- Tsutsumi A, Kawamata T, Izumi N, Seitz H, Tomari Y. Recognition of the pre-miRNA structure by Drosophila Dicer-1. Nat Struct Mol Biol 2011; 18:1153 - 8; http://dx.doi.org/10.1038/nsmb.2125; PMID: 21926993
- Chakravarthy S, Sternberg SH, Kellenberger CA, Doudna JA. Substrate-specific kinetics of Dicer-catalyzed RNA processing. J Mol Biol 2010; 404:392 - 402; http://dx.doi.org/10.1016/j.jmb.2010.09.030; PMID: 20932845
- Yoneyama M, Kikuchi M, Matsumoto K, Imaizumi T, Miyagishi M, Taira K, et al. Shared and unique functions of the DExD/H-box helicases RIG-I, MDA5, and LGP2 in antiviral innate immunity. J Immunol 2005; 175:2851 - 8; PMID: 16116171
- Yoneyama M, Kikuchi M, Natsukawa T, Shinobu N, Imaizumi T, Miyagishi M, et al. The RNA helicase RIG-I has an essential function in double-stranded RNA-induced innate antiviral responses. Nat Immunol 2004; 5:730 - 7; http://dx.doi.org/10.1038/ni1087; PMID: 15208624
- Sun YW. RIG-I, a human homolog gene of RNA helicase, is induced by retinoic acid during the differentiation of acute promyelocytic leukemia cell. Thesis, Shanghai Institute of Hematology, China (1997).
- Cui Y, Li M, Walton KD, Sun K, Hanover JA, Furth PA, et al. The Stat3/5 locus encodes novel endoplasmic reticulum and helicase-like proteins that are preferentially expressed in normal and neoplastic mammary tissue. Genomics 2001; 78:129 - 34; http://dx.doi.org/10.1006/geno.2001.6661; PMID: 11735219
- Bruns AM, Horvath CM. Activation of RIG-I-like receptor signal transduction. Crit Rev Biochem Mol Biol 2012; 47:194 - 206; http://dx.doi.org/10.3109/10409238.2011.630974; PMID: 22066529
- Kawai T, Akira S. Antiviral signaling through pattern recognition receptors. J Biochem 2007; 141:137 - 45; http://dx.doi.org/10.1093/jb/mvm032; PMID: 17190786
- Satoh T, Kato H, Kumagai Y, Yoneyama M, Sato S, Matsushita K, et al. LGP2 is a positive regulator of RIG-I- and MDA5-mediated antiviral responses. Proc Natl Acad Sci U S A 2010; 107:1512 - 7; http://dx.doi.org/10.1073/pnas.0912986107; PMID: 20080593
- Komuro A, Horvath CM. RNA- and virus-independent inhibition of antiviral signaling by RNA helicase LGP2. J Virol 2006; 80:12332 - 42; http://dx.doi.org/10.1128/JVI.01325-06; PMID: 17020950
- Fujita T, Onoguchi K, Onomoto K, Hirai R, Yoneyama M. Triggering antiviral response by RIG-I-related RNA helicases. Biochimie 2007; 89:754 - 60; http://dx.doi.org/10.1016/j.biochi.2007.01.013; PMID: 17379377
- Seth RB, Sun L, Chen ZJ. Antiviral innate immunity pathways. Cell Res 2006; 16:141 - 7; http://dx.doi.org/10.1038/sj.cr.7310019; PMID: 16474426
- Kübler K, Gehrke N, Riemann S, Böhnert V, Zillinger T, Hartmann E, et al. Targeted activation of RNA helicase retinoic acid-inducible gene-I induces proimmunogenic apoptosis of human ovarian cancer cells. Cancer Res 2010; 70:5293 - 304; http://dx.doi.org/10.1158/0008-5472.CAN-10-0825; PMID: 20551064
- Poeck H, Besch R, Maihoefer C, Renn M, Tormo D, Morskaya SS, et al. 5′-Triphosphate-siRNA: turning gene silencing and Rig-I activation against melanoma. Nat Med 2008; 14:1256 - 63; http://dx.doi.org/10.1038/nm.1887; PMID: 18978796
- Zitvogel L, Kroemer G. Anticancer immunochemotherapy using adjuvants with direct cytotoxic effects. J Clin Invest 2009; 119:2127 - 30; PMID: 19620780
- Schlee M, Roth A, Hornung V, Hagmann CA, Wimmenauer V, Barchet W, et al. Recognition of 5′ triphosphate by RIG-I helicase requires short blunt double-stranded RNA as contained in panhandle of negative-strand virus. Immunity 2009; 31:25 - 34; http://dx.doi.org/10.1016/j.immuni.2009.05.008; PMID: 19576794
- Hornung V, Ellegast J, Kim S, Brzózka K, Jung A, Kato H, et al. 5′-Triphosphate RNA is the ligand for RIG-I. Science 2006; 314:994 - 7; http://dx.doi.org/10.1126/science.1132505; PMID: 17038590
- Binder M, Eberle F, Seitz S, Mücke N, Hüber CM, Kiani N, et al. Molecular mechanism of signal perception and integration by the innate immune sensor retinoic acid-inducible gene-I (RIG-I). J Biol Chem 2011; 286:27278 - 87; http://dx.doi.org/10.1074/jbc.M111.256974; PMID: 21659521
- Kato H, Takeuchi O, Sato S, Yoneyama M, Yamamoto M, Matsui K, et al. Differential roles of MDA5 and RIG-I helicases in the recognition of RNA viruses. Nature 2006; 441:101 - 5; http://dx.doi.org/10.1038/nature04734; PMID: 16625202
- Peisley A, Lin C, Wu B, Orme-Johnson M, Liu M, Walz T, et al. Cooperative assembly and dynamic disassembly of MDA5 filaments for viral dsRNA recognition. Proc Natl Acad Sci U S A 2011; 108:21010 - 5; http://dx.doi.org/10.1073/pnas.1113651108; PMID: 22160685
- Ranjith-Kumar CT, Murali A, Dong W, Srisathiyanarayanan D, Vaughan R, Ortiz-Alacantara J, et al. Agonist and antagonist recognition by RIG-I, a cytoplasmic innate immunity receptor. J Biol Chem 2009; 284:1155 - 65; http://dx.doi.org/10.1074/jbc.M806219200; PMID: 19019822
- Wang Y, Ludwig J, Schuberth C, Goldeck M, Schlee M, Li H, et al. Structural and functional insights into 5′-ppp RNA pattern recognition by the innate immune receptor RIG-I. Nat Struct Mol Biol 2010; 17:781 - 7; http://dx.doi.org/10.1038/nsmb.1863; PMID: 20581823
- Lu C, Xu H, Ranjith-Kumar CT, Brooks MT, Hou TY, Hu F, et al. The structural basis of 5′ triphosphate double-stranded RNA recognition by RIG-I C-terminal domain. Structure 2010; 18:1032 - 43; http://dx.doi.org/10.1016/j.str.2010.05.007; PMID: 20637642
- Cui S, Eisenächer K, Kirchhofer A, Brzózka K, Lammens A, Lammens K, et al. The C-terminal regulatory domain is the RNA 5′-triphosphate sensor of RIG-I. Mol Cell 2008; 29:169 - 79; http://dx.doi.org/10.1016/j.molcel.2007.10.032; PMID: 18243112
- Mi Z, Fu J, Xiong Y, Tang H. SUMOylation of RIG-I positively regulates the type I interferon signaling. Protein Cell 2010; 1:275 - 83; http://dx.doi.org/10.1007/s13238-010-0030-1; PMID: 21203974
- Gack MU, Nistal-Villán E, Inn KS, García-Sastre A, Jung JU. Phosphorylation-mediated negative regulation of RIG-I antiviral activity. J Virol 2010; 84:3220 - 9; http://dx.doi.org/10.1128/JVI.02241-09; PMID: 20071582
- Gack MU, Shin YC, Joo CH, Urano T, Liang C, Sun L, et al. TRIM25 RING-finger E3 ubiquitin ligase is essential for RIG-I-mediated antiviral activity. Nature 2007; 446:916 - 20; http://dx.doi.org/10.1038/nature05732; PMID: 17392790
- Jiang X, Kinch LN, Brautigam CA, Chen X, Du F, Grishin NV, et al. Ubiquitin-induced oligomerization of the RNA sensors RIG-I and MDA5 activates antiviral innate immune response. Immunity 2012; 36:959 - 73; http://dx.doi.org/10.1016/j.immuni.2012.03.022; PMID: 22705106
- Zeng W, Sun L, Jiang X, Chen X, Hou F, Adhikari A, et al. Reconstitution of the RIG-I pathway reveals a signaling role of unanchored polyubiquitin chains in innate immunity. Cell 2010; 141:315 - 30; http://dx.doi.org/10.1016/j.cell.2010.03.029; PMID: 20403326
- Hou F, Sun L, Zheng H, Skaug B, Jiang QX, Chen ZJ. MAVS forms functional prion-like aggregates to activate and propagate antiviral innate immune response. Cell 2011; 146:448 - 61; http://dx.doi.org/10.1016/j.cell.2011.06.041; PMID: 21782231
- Bamming D, Horvath CM. Regulation of signal transduction by enzymatically inactive antiviral RNA helicase proteins MDA5, RIG-I, and LGP2. J Biol Chem 2009; 284:9700 - 12; http://dx.doi.org/10.1074/jbc.M807365200; PMID: 19211564
- Leung DW, Amarasinghe GK. Structural insights into RNA recognition and activation of RIG-I-like receptors. Curr Opin Struct Biol 2012; 22:297 - 303; http://dx.doi.org/10.1016/j.sbi.2012.03.011; PMID: 22560447
- Halic M, Moazed D. 22G-RNAs in transposon silencing and centromere function. Mol Cell 2009; 36:170 - 1; http://dx.doi.org/10.1016/j.molcel.2009.10.010; PMID: 19854125
- Gu W, Shirayama M, Conte D Jr., Vasale J, Batista PJ, Claycomb JM, et al. Distinct argonaute-mediated 22G-RNA pathways direct genome surveillance in the C. elegans germline. Mol Cell 2009; 36:231 - 44; http://dx.doi.org/10.1016/j.molcel.2009.09.020; PMID: 19800275
- Claycomb JM, Batista PJ, Pang KM, Gu W, Vasale JJ, van Wolfswinkel JC, et al. The Argonaute CSR-1 and its 22G-RNA cofactors are required for holocentric chromosome segregation. Cell 2009; 139:123 - 34; http://dx.doi.org/10.1016/j.cell.2009.09.014; PMID: 19804758
- Nakamura M, Ando R, Nakazawa T, Yudazono T, Tsutsumi N, Hatanaka N, et al. Dicer-related drh-3 gene functions in germ-line development by maintenance of chromosomal integrity in Caenorhabditis elegans. Genes Cells 2007; 12:997 - 1010; http://dx.doi.org/10.1111/j.1365-2443.2007.01111.x; PMID: 17825044
- Sijen T, Steiner FA, Thijssen KL, Plasterk RH. Secondary siRNAs result from unprimed RNA synthesis and form a distinct class. Science 2007; 315:244 - 7; http://dx.doi.org/10.1126/science.1136699; PMID: 17158288
- Pak J, Fire A. Distinct populations of primary and secondary effectors during RNAi in C. elegans. Science 2007; 315:241 - 4; http://dx.doi.org/10.1126/science.1132839; PMID: 17124291
- Caruthers JM, McKay DB. Helicase structure and mechanism. Curr Opin Struct Biol 2002; 12:123 - 33; http://dx.doi.org/10.1016/S0959-440X(02)00298-1; PMID: 11839499
- Lu R, Yigit E, Li WX, Ding SW. An RIG-I-Like RNA helicase mediates antiviral RNAi downstream of viral siRNA biogenesis in Caenorhabditis elegans. PLoS Pathog 2009; 5:e1000286; http://dx.doi.org/10.1371/journal.ppat.1000286; PMID: 19197349
- Tabara H, Yigit E, Siomi H, Mello CC. The dsRNA binding protein RDE-4 interacts with RDE-1, DCR-1, and a DExH-box helicase to direct RNAi in C. elegans. Cell 2002; 109:861 - 71; http://dx.doi.org/10.1016/S0092-8674(02)00793-6; PMID: 12110183
- Ma E, MacRae IJ, Kirsch JF, Doudna JA. Autoinhibition of human dicer by its internal helicase domain. J Mol Biol 2008; 380:237 - 43; http://dx.doi.org/10.1016/j.jmb.2008.05.005; PMID: 18508075
- Nykänen A, Haley B, Zamore PD. ATP requirements and small interfering RNA structure in the RNA interference pathway. Cell 2001; 107:309 - 21; http://dx.doi.org/10.1016/S0092-8674(01)00547-5; PMID: 11701122
- Welker NC, Pavelec DM, Nix DA, Duchaine TF, Kennedy S, Bass BL. Dicer’s helicase domain is required for accumulation of some, but not all, C. elegans endogenous siRNAs. RNA 2010; 16:893 - 903; http://dx.doi.org/10.1261/rna.2122010; PMID: 20354150
- Welker NC, Maity TS, Ye X, Aruscavage PJ, Krauchuk AA, Liu Q, et al. Dicer’s helicase domain discriminates dsRNA termini to promote an altered reaction mode. Mol Cell 2011; 41:589 - 99; http://dx.doi.org/10.1016/j.molcel.2011.02.005; PMID: 21362554
- Peisley A, Lin C, Wu B, Orme-Johnson M, Liu M, Walz T, et al. Cooperative assembly and dynamic disassembly of MDA5 filaments for viral dsRNA recognition. Proc Natl Acad Sci U S A 2011; 108:21010 - 5; http://dx.doi.org/10.1073/pnas.1113651108; PMID: 22160685
- Levin MK, Wang YH, Patel SS. The functional interaction of the hepatitis C virus helicase molecules is responsible for unwinding processivity. J Biol Chem 2004; 279:26005 - 12; http://dx.doi.org/10.1074/jbc.M403257200; PMID: 15087464
- Berke IC, Modis Y. MDA5 cooperatively forms dimers and ATP-sensitive filaments upon binding double-stranded RNA. EMBO J 2012; 31:1714 - 26; http://dx.doi.org/10.1038/emboj.2012.19; PMID: 22314235
- Okonechnikov K, Golosova O, Fursov M, UGENE team. Unipro UGENE: a unified bioinformatics toolkit. Bioinformatics 2012; 28:1166 - 7; http://dx.doi.org/10.1093/bioinformatics/bts091; PMID: 22368248
- Notredame C, Higgins DG, Heringa J. T-Coffee: A novel method for fast and accurate multiple sequence alignment. J Mol Biol 2000; 302:205 - 17; http://dx.doi.org/10.1006/jmbi.2000.4042; PMID: 10964570