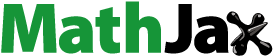
Abstract
Glasses based on the SiO2–Al2O3–MgO–K2O–B2O3–MgF2 system added with TiO2 were synthesized and characterized by differential scanning calorimetry (DSC), X-ray powder diffraction (XRD), Fourier transform infrared spectroscopy (FTIR) and scanning electron microscopy (SEM) techniques. The results showed that by increasing the TiO2 content, glass transition temperature (Tg), both the crystallization peak temperature (TpI and TpII) and activation energy (E) increased. The interconnected large blocky card-like crystals were identified as fluorophlogopite as a major crystalline phase for all three glass-ceramic specimens by (XRD) and subsequently confirmed by SEM. The Vickers hardness (Hv) value for glass-ceramic specimens decreases with progressively increasing TiO2 content (1–10 wt%) and Titania containing MGT-3 glass-ceramic specimen with interconnected large blocky card-like fluorophlogopite mica crystals possess lower Hv (4.26 GPa) as well as good machinability (m = 0.12) useable for machinable applications. The study shows that Titania promoted initial crystallization of glass and can be used as an effective nucleting agent.
1 Introduction
Glass-ceramics materials are polycrystalline solids, prepared by the controlled crystallization technique. After crystallization process of the mica composition based glass through heat treatment, interconnected mica crystals are developed in whole the glass matrix where the main crystalline phase is synthetic fluormica, named fluorophlogopite. Crystallization is a thermodynamically favorable and kinetically controlled process [Citation1]. The various nucleating agents are generally added into the glass to induce crystallization. Beall and his research group [Citation2] used TiO2, ZrO2, P2O5, Ta2O5, Fe2O3, and F as heterogeneous nucleating agents for crystallization of various silicate glass systems. These nucleating agents either accrues in a specific microphase of the phase separated base glass or support the phase separation. Cormier et al. [Citation3] have studied the role of different amount of TiO2 in magnesium aluminosilicate glass. Peixin et al. [Citation4] have studied the influence of TiO2 and ZrO2 on the crystallization of glass with the chemical composition of 45MgO–20B2O3–35SiO2 (wt%). Crystallization process is generally affected by nucleating agent via two mechanisms. At first, it can support the crystallization by forming a metastable liquid–liquid phase separation, which have to be the starting point for the internal crystallization of glass [Citation1]. Secondly, small crystalline precipitates containing titanium ion or zirconium ion can also act as heterogeneous nucleation sites for further crystallization of the glass matrix [Citation1,Citation5]. Cheng et al. [Citation6] studied the crystallization kinetics of a glass based on one type of mica, NaMg3AlSi3O10F2, with the addition of TiO2 as nucleating agent. After crystallization process of the mica composition based glass system through heat treatment, interconnected mica crystals are developed in whole the glass where the main crystalline phase is synthetic fluormica, named fluorophlogopite. Peixin et al. [Citation4] have been established that the crystallization of 45MgO–20B2O3–35SiO2 (wt%) glass led to the formation of a major Mg2B2O5 phase, and minor crystalline phases Mg3B2O6 and Mg2SiO4. According to their [Citation4] findings, both TiO2 and ZrO2 promoted the phase separation during the annealing process above the glass transition temperature (Tg). In the earlier work [Citation7–Citation11], authors had studied the kinetics as well as crystallization behavior, microstructure and mechanical properties of different glass system using CaF2, TiO2 as a nucleating agents and also using nano silica. Authors [Citation12] had studied the kinetics as well as crystallization behavior and microstructure with respect to B2O3 content in the barium fluorophologopite glass-ceramics based on the SiO2–Al2O3–MgO–BaO–MgF2 system. The aim of the present work is to investigate the possible role of TiO2 in the SiO2–Al2O3–MgO–K2O–B2O3–MgF2 glass system. In order to achieved the improvement of hardness and machinability. Titania promoted initial crystallization of glass and can be used as an effective nucleting agent to generate fluorophlogopite mica in these glass-ceramics useable for solid oxide fuel cell (SOFC) applications.
2 Experimental procedure
shows the composition of all glass batches (MGT-1, MGT-2 and MGT-3). The glasses have been labeled in accordance with their respective TiO2 contents (wt%). The raw materials used to prepared the glasses are SiO2 (99.8%), Al2O3 (99.3%), MgO (99.9%), K2CO3 (98%), H3BO3 (99.5%), MgF2 (99.9%), and TiO2 (99.5%) supplied by M/S Merck Specialties Private Limited, India. All batches were melted at 1500 °C for 2 h in an alumina crucible in air in an electrically heated raising hearth furnace followed by pouring on a steel plate. After release from the plate, the resultant glasses were immediately transferred to an annealing furnace operating 630 °C for 2 h to remove the internal stress and then cooled down slowly to room temperature. The anneal glasses were sized as required for different experimentations and subsequently heat-treatment at different temperature.
Table 1 Chemical compositions of base glass.
Differential scanning calorimetry of the glass powders weighing near about 50 mg in an alumina crucible were carried out in nitrogen atmosphere from room temperature to 1000 °C at heating rate 10 °C/min using α-alumina powder as a reference material with the help of a DSC instrument (Model STA 449C; NETZSCH-Gerätebau GmbH, Selb, Germany) to determine the glass transition temperature (Tg) and crystallization peak temperature (Tp) with a measurement error of ±1 °C. DSC of glass powders were carried out at four different heating rates (β) such as 5, 10, 15 and 20 °C/min to determined the crystallization kinetics.
The amorphous nature of glasses and qualitative analysis of crystalline phases in the glass-ceramics were investigated using a conventional Bragg–Brentano diffractometer (PANalytical PW3040/60, The Netherlands) with Ni-filtered Cu-Ka radiation (λ = 1.5406 Å) at 40 kV and 30 mA. The scan range was 5°–80° with a step size of 0.05°.
Fourier transform infrared transmission spectra (FTIR) of glass-ceramics were recorded in the wave number range 400–2000 cm−1 with the help of FTIR spectrometer (Alpha FTIR, Bruker, Germany) with a resolution of ±1 cm−1, in order to identify different bonds present in the glass ceramics and crystals structure.
The crystallization characteristics and microstructural studies of the heat-treated glass-ceramics samples were examined by scanning electron microscope (ZEISS EVO-MA 10, Germany). For this purpose the heat-treated and polished samples were etched with 2 vol.% HF aqueous solution for 6 min.
The hardness of the all glass-ceramic specimens was measured by micro-indentation on the polished surface of the specimens. Micro-indentations were taken using 160 microhardness testers (Carl Zeiss Jena, Germany) equipped with a conical Vickers indenter at an indent load of 40 g. Ten indents were taken for each sample with identical loading condition and average of this was used to calculate the hardness using the standard equation for the Vickers geometry.(1)
(1) where Hv is the Vickers hardness number (VHN) in kg/mm2, P is the applied load in kg, and d is the mean of the lengths diagonals of the indentation in mm. Baik et al. [Citation13,Citation14] introduced the machinability parameter m as,
(2)
(2) The machinability parameter m is an important tool to characterize machinable glass-ceramics.
3 Results and discussion
3.1 DSC analysis results
The glass to glass-ceramic conversion, the desired crystalline phases is a phase transformation process that requires control heat treatment. The heat treatment temperatures were selected from differential scanning calorimetry (DSC) study and the results have been demonstrated in . The endothermic minima correspond to glass transition while the two exothermic peaks correspond to the crystallization into the glass. Two exothermic peaks were observed for all the batches. showed that by increasing the TiO2 content (1–10 wt%), the glass transition temperature (Tg) and both the crystallization peak temperature (TpI and TpII) increased. The Tg obtained for MGT-1 glass specimen is 705 °C, whereas MGT-2 and MGT-3 have Tg value of 708 and 723 °C respectively. After the Tg two exothermic peaks were observed and they corresponds to stat the crystallization process. These exothermic peaks started for MGT-1 glass specimen were at 874 °C (TpI) and 938 °C (TpII) whereas for MGT-2 and MGT-3 glass specimens 882 °C (TpI) and 959 °C (TpII); 902 °C (TpI) and 980 °C (TpII) respectively. Agarwal [Citation15] proposed that when a modifier cation is added in silica containing glass melt, it surrounded by both bridging oxygen and non-bridging oxygen. These oxygen isolate the network-modifier cations from each other by providing screens that mask the positive charge. Besides this, modifier cations that are partly or wholly coordination by bridging oxygen are poorly shielded from each other's influence because the oxygen are mainly bonded to Si4+ cations by strong covalent bond. When metal oxide is added in the glass system, such as TiO2 in this case, the interaction of units will be accelerated by group [TiO4] due to its higher negative attractive force to some positive cations such as Mg+ and K+ under some suitable conditions. The result of this linking is that some different phase containing Mg+ and K+ can be observed by different characterizations methods.
DSC was performed for MGT-1, MGT-2 and MGT-3 glasses (nucleating agent-doped as 1–10 wt% TiO2) at different heating rate to determine the activation energy of crystallization and types of crystal growth occurs. The variations of TiO2 on the crystallization kinetics of the glass specimens under a given thermal cycle are essential to predict their behavior in practical applications. The model was developed by Matusita and Saka [Citation16] using the following modified form of the Kissinger equation.(3)
(3) where β is the heating rate, Tp is the crystallization peak temperature of the DSC maximum exotherm, R is the universal gas constant, E is the activation energy for crystallization and C is a constant. From the slope and intercept of the plot ln(Tp2/β) vs. 1/Tp given by Eq. Equation(3)
(3)
(3) , one can derived the E. The linear plots of ln(Tp2/β) vs. 1/Tp for first and second crystallization peak temperature (TpI and TpII) are shown in . From the value of activation energy (E), the Avrami exponent (n) was calculated using Augis–Bennett equation [Citation17].
(4)
(4) where, ΔT is the full width of the exothermic DSC peak at the half maximum intensity. The calculated E and n values are listed in . It can be seen that E for both first and second crystallization peak temperature (TpI and TpII) increased from ∼299 kJ/mol to ∼370 kJ/mol for TpI and ∼385 kJ/mol to ∼428 kJ/mol for TpII upon addition of 1–10 wt% TiO2. According to Johnson–Mehl–Avrami theory, Avrami exponent (n) depends on the crystallization manner [Citation12]. In present cases the values of Avrami exponent for first TpI were 2.91, 2.74 and 3.02 and second TpII were 2.61, 2.84, and 3.08 for the glass specimens MGT-1, MGT-2 and MGT-3 respectively (). The n values for the TpI and TpII suggest that the crystal growths are two-dimensional in nature at the time of crystallization process.
Table 2 Activation energy (E) and Avrami parameter (n) of the glass specimens.
3.2 XRD analysis results
shows the X-ray diffraction patterns of each glass-ceramics powder heat treated at 1080 °C. From this figure, it was clearly noted that the major or predominant crystalline phase for all specimens were fluorophlogopite mica, KMg3(AlSi3O10)F2 (Monoclinic system, with space group C2/m(12), JCPDS file no. 10-0494) along with forsterite [Mg2SiO4 – orthorhombic system, with space group Pbnm(62), JCPDS file no. 80-0783] and cordierite [Mg2Al4Si5O18 – hexagonal system, with space group P6/mcc(192), JCPDS file no. 82-1884] appeared as minor or secondary crystalline phases. The intensity and the number of this phases increase with increase in heat treatment temperature from 800 °C to 1080 °C as represented in . In the MGT-1 specimen, heat treated at 1080 °C, peaks of forsterite at 22.6°, 34.1°, 36.0° and 71.2° (2θ) appeared along with the fluorophlogopite mica at 30.2°, 39.8°, 41.3° and 60.3° (2θ) as a major crystalline phase. In the MGT-2 specimen, at the same heat treated temperature, five new peaks of fluorophlogopite mica appeared at 8.9°, 26.7°, 28.4°, 31.2° and 62.8° (2θ) and one new peak of cordierite at 11.3° (2θ) along with the fluorophlogopite. In MGT-3 (10 wt% TiO2) specimen heat treated at 1080 °C, two new peaks of cordierite appeared at 19.3°, 45.6° (2θ) and one peak of cordierite at 11.3° and fluorophlogopite at 62.8° (2θ) disappeared along with the all crystalline phases. From this analysis it was clearly observed that at the high heat treated temperature and higher amount of TiO2 (10 wt% TiO2) the glass specimens were crystallized completely to form glass-ceramic and this is an irreversible ceramization process.
3.3 FTIR analysis results
The FTIR transmission spectra in the range of 400–2000 cm−1 of the glass-ceramics specimens containing TiO2 (1–10 wt%), which are heat treated at 1080 °C for 2 h are depicted in . The glass-ceramics specimens are shown a peak at 472 cm−1, which is due to Si–O–Si bending vibration modes of [SiO4] unit along with the presence of Ti–O–Ti-stretching vibration in this region [Citation18–Citation20]. The transmission peak at 508 cm−1 may be attributed to the bending vibration of O–Si–O and Si–O–Si bond of [SiO4] units [Citation21]. The band at 649 cm−1 for all the spectra is assigned to Al–O–Al stretching vibration of [AlO6] units [Citation22]. It is known that bands in-between at 660–690 cm−1 are due to vibration in [TiO4] tetrahedron [Citation23]. The dissolved Ti4+ cations in silico-oxide network may lead the transmission band to shift toward high wavenumber. So the transmission peak at 685 and 721 cm−1 for all the spectra of are due to stretching vibration of –O–Si–O– in [SiO4] tetrahedron as well as stretching vibration of Si–O–Ti between [SiO4] and [TiO4] of the network. The peak near 851 cm−1 is a characteristic band for Al–O stretching vibration of [AlO4] units [Citation22]. The band position at 951 cm−1 is due to the B–O stretching vibration of [BO4] units in tri, tetra, and penta borate groups [Citation24]. The sharp peak observed at 1011 cm−1 corresponds to the fluorphlogopite. This peak is assigned to Si–O–Si asymmetric stretching vibration [Citation18–Citation20]. This intense peak was due to the presence of B2O3. This sharp broadening peak at 1011 cm−1 corresponds to the B–O stretching vibration of [BO4] units [Citation12,Citation25,Citation26]. The band at 1074 cm−1 is for the anti-symmetric stretching vibration of Si–O–Si of [SiO4] unit [Citation21,Citation24]. The peak position at 1227 cm−1 is attributed to B–O stretching vibration of trigonal BO4 units in boroxol rings [Citation27]. The peak position at 1259 cm−1 is assigned to anti-symmetric stretching vibration of Si–O–Si of [SiO4] unit. The band position attributed at 1429 cm−1 is due to the B–O vibration related to tri-[BO3], tetraborate [BO4] groups. The peak position observed at 1631 cm−1 is due to the H–O–H bending vibration for water molecule [Citation19].
3.4 SEM analysis results
Scanning electron microscopy photomicrographs of the glass-ceramic specimens heat treated at 1080 °C for 2 h are represented in . (a)–(c) depicts the microstructure of MGT-1 (TiO2–1 wt%), MGT-2 (TiO2–5 wt%) and MGT-3 (TiO2–10 wt%) glass-ceramic, respectively. All these photomicrographs show fluorophlogopite crystal dispersed throughout the glass matrix. MGT-1 glass-ceramic contains blocky card like mica flake crystals ((a)). When TiO2 (5 wt%) is added as a nucleating agent in MGT-2, uniform and dense blocky microstructure obtained where strand like fluorophlogopite mica crystal phase develops throughout the matrix ((b)). The crystal sizes are about 5–6 μm for all the glass-ceramic specimens. In (c), i.e., 10 wt% TiO2 containing glass-ceramic, interconnected large blocky card-like fluorophlogopite mica crystals predominant. This type of microstructure arrangements into the glass matrix has sufficient tendency to prevent the generation and growth of crack. The appearance of blocky crystals might be due to the formation of fluorophlogopite mica crystals for all the glass-ceramic specimens concerned.
3.5 Mechanical properties
shows the machining properties of the specimen's heat treatment in the temperature range 800–1080 °C for 2 h. According to , the hardness values reduce slightly on formation of fluorophlogopite mica crystal. The glass-ceramic specimen MGT-1 has hardness value of 5.33 GPa (at 800 °C) which falls to 5.17 GPa after heat treatment at 1080 °C for 2 h. Hence, when the heat treatment temperature progressively increases, Hv values gradually decrease. The glass-ceramic specimen MGT-3 has hardness value 4.73 GPa (at 800 °C) which reduces to 4.26 GPa after heat treatment at 1080 °C for 2 h. The large reduction of hardness value may be interpreted by the formation of interlocking blocky house of cards like microstructure [Citation28,Citation29]. also shows the machinability parameters (m) calculated from hardness values after heat treatment at different temperatures (800–1080 °C for 2 h). According to the result table, MGT-1 glass-ceramic specimen has the Hv value 5.17 GPa (at 1080 °C/2 h), but it does not have the machinability (m = 0.012) as it shows only surface crystallization into the glass matrix. By the heat treatment at 1080 °C for about 2 h, glass-ceramic specimen MGT-3 shows a lowest hardness value 4.26 GPa, indicating good machinability (m = 0.123). The above discussion clearly indicated that, as the TiO2 content increased (1–10 wt%) the machinablity of the specimens increased.
Table 3 Mechanical measurement properties of glass-ceramic specimens heat treated at different temperature.
4 Conclusions
The effect of TiO2 on properties of SiO2–Al2O3–MgO–K2O–BaO–MgF2 glass system has been investigated in this study in order to establish their applicability as machinable application. The variation in TiO2 content (1–10 wt%) has a marked influence on crystallization as well as crystal morphology. From DSC study, Tg and both the crystallization peak temperature (TpI and TpII) of the glass specimens were increased with the addition of TiO2 content (1–10 wt%) due to the high field strength of Ti4+ ion. The crystals formed were largely homogeneous and two-dimensional in nature for all the glass-ceramic specimens concern. The XRD analysis indicated the formation of fluorophlogopite mica crystal as a predominant phase along with forstertite and cordierite minor phases. The FTIR analysis confirmed the presence of Si–O–Si bending vibration modes of [SiO4] unit along with the presence of Ti–O–Ti-stretching vibration in the 472 cm−1 wave number region. The aspect ratio of fluorophlogopite mica crystals increased with increase of heat treatment temperature and higher TiO2 content in the composition of glass specimens as also identified by XRD and SEM study. The Hv value for glass-ceramic specimens decreases with progressively increasing TiO2 content and Titania containing (10 wt%) mica glass-ceramic with interconnected large blocky card-like fluorophlogopite mica crystals. It possess lower Hv (4.26 GPa) as well as good machinability (m = 0.12) which results in easy machining. TiO2 promoted initial crystallization of glass and can be used as an effective nucleating agent for machinable applications.
Acknowledgements
The authors would like to thank the UPE scheme of University Grants Commission (UGC) and the Center for Research in Nanoscience and Nanotechnology (CRNN), University of Calcutta for financial support. One of the authors, Debasis Pradip Mukherjee thanks the Center for Research in Nanoscience and Nanotechnology (CRNN), University of Calcutta, Kolkata, India, for providing the Senior Research Fellowship (SRF).
Notes
Peer review under responsibility of The Ceramic Society of Japan and the Korean Ceramic Society.
References
- D.G.GrossmanJ. Am. Ceram. Soc.5591972446449
- W.HölandG.BeallGlass-Ceramic Technology2002The American Ceramic SocietyWesterville
- L.CormierO.DargaudN.MenguyG.S.HendersonM.GuignardN.TrceraB.WattsCryst. Growth Des.1112011311319
- Z.PeixinS.ZhitongZ.JianhuaChin. J. Mater. Res.121998303
- G.CarlT.HöcheB.VoigtPhys. Chem. Glasses43C2002256258
- K.ChengJ.WanK.LiangJ. Am. Ceram. Soc.825199912121216
- D.P.MukherjeeS.K.DasCeram. Int.3912013571578
- D.P.MukherjeeS.K.DasJ. Non-Cryst. Solids368201398104
- D.P.MukherjeeT.DattaS.K.DasAIP Conf. Proc.15362013625626
- D.P.MukherjeeJ.K.SinhaS.K.DasAIP Conf. Proc.15362013639640
- D.P.MukherjeeS.K.DasCeram. Int.403201441274134
- D.P.MukherjeeS.K.DasCeram. Int.40820141245912470
- D.S.BaikK.S.NoJ.S.ChunY.J.YoonH.Y.ChoJ. Mater. Sci.30199518011806
- D.S.BaikK.S.NoJ.S.ChunH.Y.ChoJ. Mater. Process. Technol.6719975054
- A.AgarwalM.TomozawaJ. Am. Ceram. Soc.7831995827829
- K.MatusitaS.SakaJ. Non-Cryst. Solids391980741746
- J.A.AugisJ.E.BennettJ. Therm. Anal. Calorim.131978283292
- J.YuX.ZhaoJ.YuG.ZhongJ.HanQ.ZhaoJ. Mater. Sci. Lett.20200117451748
- N.A.ShafiM.M.MorsiJ. Mater. Sci.32199751855189
- V.L.BurdickD.E.DayJ. Am. Ceram. Soc.502196797101
- G.FuxiOptical Spectroscopic Properties of Glass1992Springer-VerlagBerlin
- V.StubičanR.RoyAm. Mineral.4619613251
- P.GonzálezS.SerraS.ListeS.ChiussiB.LeónM.Pérez-AmorJ. Non-Cryst. Solids3201–320039299
- E.I.KamitsosM.A.KarakassidesG.D.ChryssikosJ. Phys. Chem.915198710731079
- G.Pal SinghP.KaurS.KaurD.P.SinghMater. Phys. Mech.1220115863
- M.R.ReddyS.B.RajuN.VeerraiahBull. Mater. Sci.24120016368
- I.ArdeleanF.CiorcasM.PeteanuI.BratuV.IoncuMod. Phys. Lett. B142000653
- A.C.Fischer-CrippsJ. Am. Ceram. Soc.8411200126032606
- A.GoelD.U.TulyaganovV.V.KhartonA.A.YaremchenkoS.AgathopoulosJ.M.F.FerreiraJ. Am. Ceram. Soc.907200722362244