Abstract
Phase separation control is an important factor to prepare a porous monolith by an aqueous sol–gel reaction. Here, we report a surfactant-free synthesis method to obtain hydrophobic polymethylsilsesquioxane aerogels by copolymerizing a cationic-functionalized alkoxysilane N-trimethoxysilylpropyl-N,N,N-trimethylammonium chloride. The resultant materials have low-density, high visible-light transmittance, and high thermal insulating equivalent to those of prepared under the presence of surfactant.
1 Introduction
Transparent silica aerogels are expected to be used as the main component of daylighting windows with high thermal insulation [Citation1 Citation2013;Citation4]. Optical and thermal insulating properties of those porous materials are derived from a well-controlled microstructure with a typical length scale in a hundred nanometer or less. To achieve better performances, many researchers have been trying to tune the pore size and morphology of skeletal structures in a desired manner. Apart from the research to brush up the excellent physical properties for applications, there is another trend in silica aerogel research. Since the ultrafine structure of the silica aerogel is too friable and easily destroyed even by small mechanical shocks or deformations, the mechanical strength has to be improved. As a further disadvantage, the final drying process usually needs a supercritical liquid under a high pressure condition, because those sensitive microstructures are collapsed by the capillary force during evaporative drying [Citation5]. These problems have been obstacles to the massive industrial production and commercial use of transparent silica aerogels for a long time.
Up to now, various approaches have been proposed to improve the mechanical strength of silica aerogels. Aging processes after gelation and surface modification of the skeleton by organic polymers are the examples of effective techniques [Citation6 Citation2013;Citation10]. However, these methods deteriorate other properties such as visible-light transparency and thermal insulation due to morphological changes of the microstructure. There are also reports aerogels with non-silica compositions such as organic polymers, which have unique mechanical properties [Citation11 Citation2013;Citation14]. For example, polyurethane aerogels have flexibility against bending and thiol-ene network polymer aerogels show a thermoresponsive shape-memory effect. However, these aerogels do not have visible-light transmittance due to their relatively coarsened and less homogeneous microstructures compared to silica counterparts.
Our group also has been trying to increase the mechanical strength of aerogels mainly for the purpose of thermal insulation [Citation15,Citation16]. Instead of the silica composition, we found the polymethylsilsesquioxane (PMSQ) network gives transparent aerogels and drastically improves strength and flexibility [Citation17,Citation18]. Each silicon atom in the network not only has siloxane bonds but also one dangling methyl group, which results in flexibility of the polysiloxane network and elasticity due to a repulsive interaction between the methyl groups. This high strength and flexibility enables to obtaining aerogel-like xerogels as large as several hundred square centimeters in area with 10 cm in thickness via evaporative drying at ambient conditions thorough temporal shrinkage by the capillary force and subsequent spring-back [Citation19]. However, such large PMSQ xerogel monoliths need careful and extended washing of surfactant that is used to control phase separation of the hydrophobic PMSQ network in the course of the aqueous sol–gel reaction, because the residual surfactant causes serious shrinkage and cracks in the monoliths during drying. For this reason, a surfactant-free synthesis process is desired. In the case of emulsion polymerization, there have been many reports on soap-free synthesis [Citation20]. Among them, co-polymerization with a small amount of hydrophilic monomers is a simple and effective method in aqueous media.
In this paper, we report a surfactant-free synthesis of PMSQ aerogels using a hydrophilic alkoxysilane as the co-precursor for controlling the phase separation. We synthesized PMSQ aerogels by employing methyltrimethoxysilane (MTMS, (a)) under the presence of a small amount of N-trimethoxysilylpropyl-N,N,N-trimethylammonium chloride (TMAC, (b)) with a quaternary ammonium salt site as one of the starting components, instead of surfactant n-hexadecyltrimethylammonium chloride (CTAC, (c)). The ionic sites introduced by TMAC are expected to give local hydrophilic portions into the hydrophobic PMSQ network, which contributes to the inhibition of macroscopic phase separation in aqueous sol–gel media and allows fabrication of monolithic gels with a homogeneous microstructure.
2 Experiment
2.1 Materials
Acetic acid, urea, methanol, and 2-propanol were purchased from Hayashi Pure Chemical Ind., Ltd., Japan. Methyltrimethoxysilane (MTMS) and N-trimethoxysilylpropyl-N,N,N-trimethylammonium chloride (TMAC) in 50% methanol solution was obtained from Shin-Etsu Chemical Co., Ltd., Japan and Gelest, Inc., USA, respectively. Surfactant n-hexadecyltrimethylammonium chloride (CTAC) was from Tokyo Chemical Ind. Co., Ltd., Japan. All reagents were used as received.
2.2 Sample preparation procedure
The outline of sample preparation is also shown in . A typical preparation procedure is as follows; 3 g of urea was dissolved in 10 mL of 5 mM acetic acid, and then 5 mL of MTMS and 1 mL of TMAC in 50 % methanol solution were added and stirred at room temperature for 30 min to allow the hydrolysis of the alkoxysilanes. Subsequently, the resultant solution was transferred to a reaction vessel and allowed to gel in a closed condition at 60 °C. After gelation occurred in ∼4 h under a raised pH by hydrolysis of urea, the gel was kept at the same temperature for 4 days for aging. The resulting wet gel was immersed in methanol for washing, and subsequently immersed in 2-propanol for solvent exchange. This alcogel was dried via a supercritical CO2 drying process under the condition of 80 °C and 14 MPa for 10 h to obtain aerogels. For comparison, the same experiment was carried out with using 3 g of urea, 10 mL of 5 mM acetic acid, 5 mL of MTMS, and 0.4 g of CTAC as a starting composition. In these experiments, the starting compositions other than the amount of TMAC solution and CTAC were fixed.
2.3 Characterization
The pore structure was observed with an FE-SEM JSM-6700F (JEOL Ltd., Japan). Bulk density was obtained by measuring the volume and weight of a carved gel. For light transmittance measurements, a UV–vis spectrometer V-670 (JASCO Corp., Japan) equipped with an integrating sphere ISN-723 was employed. Direct-hemispherical transmittance was recorded, and obtained transmittance data at 550 nm were normalized into those corresponding the thickness of 10 mm using the Lambert–Beer equation. To assess the molecular structure of obtained PMSQ networks, solid-state NMR and Fourier transform infrared (FTIR) measurements were performed. 29Si solid-state cross-polarization/magic angle spinning (CP/MAS) NMR measurements were performed on an NMR spectrometer Avance III 800 (Bruker Corp., Germany) operated under a static magnetic field of 18.8 T. The contact time for the 1H–29Si cross-polarization was fixed at 5.5 ms and the rate of sample spinning was 15 kHz. The 29Si chemical shift was expressed relative to tetramethylsilane (Me4Si) by using the resonance line at −9.66 ppm for hexamethylcyclotrisiloxane crystals as an external reference. For FTIR measurements, IRAffinity-1 (Shimadzu Corp., Japan) with an attenuated total reflection (ATR) attachment was used. A total of 100 scans were recorded with a resolution of 4 cm−1. Mechanical properties of aerogels were measured by a material tester EZGraph (Shimadzu Corp., Japan). Aerogel samples shaped into cuboid were compressed using a load cell of 5 kN. The uniaxial compression measurements were performed at a crosshead speed of 0.5 mm s−1. Young’s modulus has been calculated using the slope of stress–strain curves between 0.1 and 0.2 MPa stress. Thermal conductivity was measured with HFM 436 Lambda (Netzsch GmbH, Germany) by measuring the heat flow at the central part of the specimen. Contact angle of water was measured with Drop Master DM-561Hi (Kyowa Interface Science Co., Ltd., Japan).
3 Results and discussion
Photographs of the gels obtained with TMAC or the surfactant CTAC in typical starting compositions (see Section 2) before and after supercritical drying and a micrograph by FESEM of the aerogels with using TMAC are shown in . Some aerogels prepared by copolymerization with TMAC are slightly yellowish, while those prepared under the presence of CTAC are colorless [Citation19,Citation21]. However, the light transmittance at the wavelength of 550 nm of the sample prepared with 1.0 mL of TMAC in 50 % methanol solution shows the maximum at 87%, which is not different from the case using the surfactant CTAC. The size of the microstructure is not significantly different as well, and it was found that crack-free transparent aerogels can be obtained by this surfactant-free method.
Fig. 1 Chemical structure of (a) methyltrimethoxysilane (MTMS), (b) N-trimethoxysilylpropyl-N,N,N-trimethylammonium chloride (TMAC), and (c) n-hexadecyltrimethylammonium chloride (CTAC).
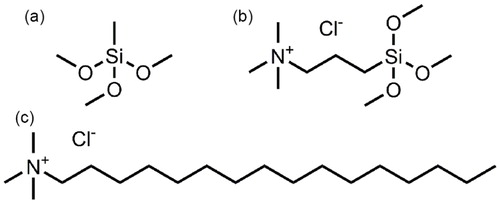
When the amount of TMAC in 50% methanol solution was varied, a change in the physical properties of the obtained aerogels was observed (). This change was similar to that observed in PMSQ aerogels prepared with CTAC as we reported before [Citation21]. When the amount of TMAC solution added is too small, the phase separation is not sufficiently suppressed and the structure becomes coarsened, so that aerogels with low visible-light transmittance were obtained. Conversely, when the amount of TMAC solution is too large, significant syneresis and drying shrinkage were observed in the obtained monoliths, due to the organic groups with large steric hindrance. Aerogels with high visible-light transmittance and free of cracks have successfully been obtained in the range of 0.5–1 mL of TMAC solution.
Fig. 2 (a) Photograph of the PMSQ aerogels synthesized without additive (left), with using 1.0 mL of TMAC in 50 % methanol solution (center) and 3.0 g of CTAC (right). Other starting compositions are 5.0 mL of MTMS, 10 mL of 5 mM HOAc aq., and 3.0 g of urea. (b) Photograph of the PMSQ aerogels synthesized with using 3.0 g of CTAC (left) and 1.0 mL of TMAC solution (right). (c) FESEM image of the PMSQ aerogel synthesized with using 1.0 mL of TMAC solution.
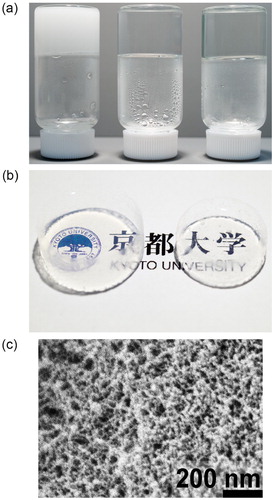
Molecular structure analysis of the aerogels obtained by the surfactant-free method was carried out. The 29Si solid-state CP/MAS NMR and FTIR spectra shown in are comparisons of the PMSQ aerogels obtained by the surfactant-free method and by the conventional method using CTAC. From the NMR spectrum, it was observed that T3 and T2 peaks (−67 and −57 ppm) slightly shifted to the higher magnetic field side in the sample prepared using TMAC [Citation22,Citation23]. This is probably because distortion in the siloxane bond angles has reduced under the presence of TMAC. The FTIR results also showed a slight change in the peak position of linear/branched siloxane (1025 cm−1) [Citation23,Citation24]. From both results, it was found that TMAC was incorporated into the network of PMSQ, and its steric repulsions of the quaternary ammonium functional group slightly changed the structure of polysiloxane network.
Fig. 3 Physical properties of aerogels derived with varied volume of TMAC in 50% methanol solution; (a) bulk density, (b) visible-light transmittance at 550 nm, and (c) Young’s modulus.

Fig. 4 (a) 29Si solid-state CP/MAS NMR and (b) FTIR spectra of the PMSQ aerogels synthesized with using TMAC and CTAC.
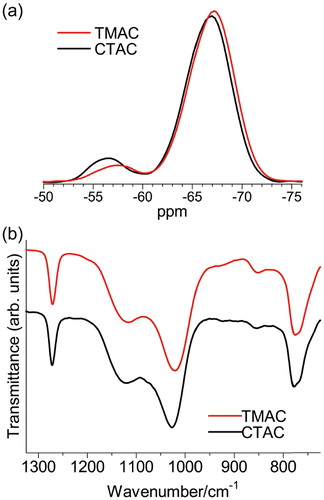
It has been found that PMSQ gels synthesized using the surfactant CTAC can be dried by evaporation to xerogels that have equivalent properties to aerogels by supercritical drying. Likewise, it was also investigated whether xerogels comparable to the corresponding aerogel could be obtained as monolith by the surfactant-free method. As a result, we were able to obtain some crack-free xerogels, but they showed some irreversible shrinkage after drying (Movie S1). In addition, as compared with the case of using the surfactant CTAC, the gel obtained by the surfactant-free method was apt to crack during drying. This is presumably due to the fact that the steric repulsions of the quaternary ammonium functional moiety are so large that siloxane chain tends to be more open and less branched in the PMSQ polymer network, which adversely affects the cracking and shrinkage behaviors due to the capillary force. In the case of the PMSQ aerogel prepared using CTAC, it was found that mechanical properties were improved by immersing in an aqueous solution containing PMSQ oligomers with heating like a hot water treatment, whereas a clear change was not observed in the samples via the surfactant-free method. This is also because the steric hindrance makes silanol groups less accessible, which prevents the skeletal structure from surface reinforcement by precipitation of the PMSQ oligomers. The preparation of PMSQ aerogel by the surfactant-free method is simple in that this method does not require a surfactant with respect to controlling the phase separation, but still needs improvements to strengthen the fine skeletons in a different way.
The water droplet contact angle of the aerogels obtained by the surfactant-free method was 122 ± 2°. Water repellency was observed, while it was inferior to the samples obtained using CTAC (140 ± 3°). This difference is derived from the introduction of the hydrophilic group. However, the aerogels by the surfactant-free method are still hydrophobic and stable against water enough to float on water for at least one week. Since the proportion of the ionic site occupied in the silsesquioxane network is not large, the aerogels are sufficiently stable without hydrophobizing treatments. Finally, we also prepared a large-area panel of aerogel via the surfactant-free method, and the thermal conductivity was measured by the heat-flow method. The value was 13.6 mW m−1 K−1. This is almost the same with that of samples produced by using CTAC and that of conventional silica aerogels [Citation19,Citation25]. Still there is a good advantage to prepare large-area thermal insulating panels via supercritical drying, that is, the washing process can be simplified by using this surfactant-free method.
4 Conclusions
Polymethylsilsesquioxane aerogel monoliths have been obtained by copolymerizing MTMS and an alkoxysilane with a quaternary ammonium functional group, without using surfactant. This new process toward PMSQ aerogels offers a simplified process especially in terms of washing. The obtained aerogel has low-density and high visible-light transmittance equivalent to those of the materials prepared under the presence of surfactant. In addition, the thermal conductivity is as low as that of conventional silica aerogels and PMSQ aerogels prepared with surfactant. Although low-density, crack-free xerogels could be obtained by evaporative drying at an ambient condition, small irreversible shrinkage remained while those prepared with surfactant do not show the shrinkage. Further improvements such as copolymerization of other different alkoxysilanes may be necessary. We believe that our surfactant-free method will become another clue to effectively control the phase separation, by which further controls over the pore structure in different materials would be possible.
Supplementary Material
Download JPEG Image (131.7 KB)Supplementary Material
Download MP4 Video (3.8 MB)Acknowledgments
This study was carried out with the NMR spectrometer in the JURC at the Institute for Chemical Research, Kyoto University. Financial support from a Grant-in-Aid for Scientific Research (KAKENHI, No. 15K17909 and 25·1089) administrated by the Japan Society for the Promotion of Science (JSPS) and the Ministry of Education, Culture, Sports, Science and Technology (MEXT), Japan. Also, partially supported by Advanced Low Carbon Technology Research and Development Program (ALCA) administrated by Japan Science and Technology Agency (JST).
Additional information
Notes on contributors
Gen Hayase
Present address: Frontier Research Institute for Interdisciplinary Sciences, Tohoku University, 6-3 Aramaki-aza Aoba, Aoba-ku, Sendai, 980-8578, Japan
References
- M. Reim W. Korner J. Manara S. Korder M. Arduini-Schuster H.P. Ebert J. Fricke Solar Energy 79 2005 131 139
- L.W. Hrubesh J. Non-Cryst. Solids 225 1998 335 342
- J. Fricke T. Tillotson Thin Solid Films 297 1997 212 223
- N. Hüsing U. Schubert Angew. Chem. Int. Ed. 37 1998 23 45
- G.W. Scherer J. Am. Ceram. Soc. 73 1990 3 14
- S. Hæreid J. Anderson M.A. Einarsrud D.W. Hua D.M. Smith J. Non-Cryst. Solids 185 1995 221 226
- B.N. Nguyen M.A.B. Meador M.E. Tousley B. Shonkwiler L. McCorkle D.A. Scheiman A. Palczer ACS Appl. Mater. Interfaces 1 2009 621 630
- M.A.B. Meador L.A. Capadona L. McCorkle D.S. Papadopoulos N. Leventis Chem. Mater. 19 2007 2247 2260
- M.A.B. Meador E.F. Fabrizio F. Ilhan A. Dass G. Zhang P. Vassilaras J.C. Johnston N. Leventis Chem. Mater. 17 2005 1085 1098
- M.-A. Einarsrud E. Nilsen J. Non-Cryst. Solids 226 1998 122 128
- N. Leventis C. Chidambareswarapattar A. Bang C. Sotiriou-Leventis ACS Appl. Mater. Interfaces 6 2014 6872 6882
- B.T. Michal W.A. Brenn B.N. Nguyen L.S. McCorkle M.A.B. Meador S.J. Rowan Chem. Mater. 28 2016 2341 2347
- J.C. Williams M.A.B. Meador L. McCorkle C. Mueller N. Wilmoth Chem. Mater. 26 2014 4163 4171
- M.A.B. Meador E.J. Malow R. Silva S. Wright D. Quade S.L. Vivod H. Guo J. Guo M. Cakmak ACS Appl. Mater. Interfaces 4 2012 536 544
- G. Hayase K. Kugimiya M. Ogawa Y. Kodera K. Kanamori K. Nakanishi J. Mater. Chem. A 2 2014 6525 6531
- G. Hayase K. Nonomura K. Kanamori A. Maeno H. Kaji K. Nakanishi Chem. Mater. 28 2016 3237 3240
- K. Kanamori M. Aizawa K. Nakanishi T. Hanada J. Sol-Gel Sci. Technol. 48 2008 172 181
- K. Kanamori M. Aizawa K. Nakanishi T. Hanada Adv. Mater. 19 2007 1589 1593
- G. Hayase K. Kanamori A. Maeno H. Kaji K. Nakanishi J. Non-Cryst. Solids 434 2016 115 119
- A.R. Goodall M.C. Wilkinson J. Hearn J. Polym. Sci. A Polym. Chem. 15 1977 2193 2218
- G. Hayase K. Kanamori K. Nakanishi Microporous Mesoporous Mater. 158 2012 247 252
- R.B. Taylor B. Parbhoo D.M. Fillmore A.L. Smith The Analytical Chemistry of Silicones 1991 John Wiley & Sons New York 347 419
- Z. Olejniczak M. Leczka K. Cholewa-Kowalska K. Wojtach M. Rokita W. Mozgawa J. Mol. Struct. 744 2005 465 471
- H. Dong J.D. Brennan Chem. Mater. 18 2006 4176 4182
- L.W. Hrubesh R.W. Pekala J. Mater. Res. 9 1994 731 738
Appendix A
Supplementary data
Supplementary data associated with this article can be found, in the online version, at http://dx.doi.org/10.1016/j.jascer.2017.02.003.
Appendix A
Supplementary data
The following is Supplementary data to this article: