Abstract
Objective
Bisphosphonates like alendronate mainly exert their effects on osteoclasts. However, osteoblasts are also affected, but exposed to a much lower concentration in vivo than the osteoclasts. Given that the effects are dose-dependent, the intention of the study was to identify a therapeutically relevant concentration of alendronate for in vitro studies on osteoblasts.
Materials and methods
Primary human osteoblasts were incubated with alendronate (5, 20 and 100 µM) for 1, 3, 7 and 14 days. Proliferation and viability were assessed, and the effects on cellular growth and function were evaluated by multianalyte profiling of selected proteins in cell culture media using the Luminex 200TM.
Results
The viability was not affected by any of the dosages. Exposure to 5 µM alendronate had a neutral effect on osteoblast proliferation, and on secretion of osteogenic and inflammatory markers, while enhancing synthesis of a marker of angiogenesis. 20 µM alendronate induced a decline in proliferation and affected angiogenic and osteogenic biomarkers adversely. 100 µM alendronate reduced proliferation dramatically, and this dosage was excluded from further experiments.
Conclusion
A concentration of 5 µM alendronate exerted effects on human osteoblasts that may translate to those observed in vivo and could therefore be relevant for in vitro studies.
Introduction
The bisphosphonate (BP) alendronate (ALN) is the most widely used drug in treatment of osteoporosis [Citation1]. BPs have demonstrated to be efficacious by increasing bone mineral density (BMD) and reducing fracture rates [Citation2]. These effects are mainly attributed to inhibition of bone resorption mediated by the osteoclasts [Citation3]. However, the effect on BMD cannot completely explain the substantial reduction in fracture incidence in patients treated with BPs. There is a body of evidence that BPs also interact with the osteoblasts, and a stimulatory effect on osteoblast proliferation and maturation has been shown in vitro [Citation4]. Moreover, both in vitro and in vivo studies have demonstrated that BPs are capable of preventing osteoblast and osteocyte apoptosis [Citation5].
A multitude of in vitro studies have been performed to explore the mechanisms of action of BPs on osteoblasts and other cell types and how these potentially may explain positive or adverse effects observed in vivo [Citation6,Citation7]. These effects are dose-dependent, and it is challenging to identify a concentration that reflect the in vivo conditions. To approach this, it is essential to understand bone metabolism and the behaviour of BPs in the body, here exemplified by ALN. The BP is rapidly eliminated from the circulation, binds with high affinity to hydroxyapatite and tends to concentrate in the vicinity of active osteoclasts, rather than at sites of bone formation [Citation8]. In a rat study, more than 70% of the osteoclast surface was densely labelled 24 h after administration of a single dose of [3H]- ALN (0.4 mg/kg), in contrast to only 2% of the bone forming surface [Citation9]. When osteoclasts secrete proteolytic enzymes and hydrochloric acid for the purpose of resorption, ALN is released and rapidly engulfed by the osteoclasts [Citation10]. Hence, osteoclasts are obviously the cells exposed to the highest concentration of ALN. The level of ALN that can be achieved in the osteoclast resorption lacuna has been calculated to be as high as 10−4 M to 10−3 M (100–1000 µM) in newborn rats [Citation9].
The in vivo and in vitro actions of BPs are well-described for osteoclasts [Citation11,Citation12], whereas the effects on osteoblast function remain to be fully elucidated. As elaborated on above, the osteoclasts are exposed to substantially higher concentrations than osteoblasts in vivo [Citation13]. Notably, concentrations of BPs as low as 10−11 M have been shown to have an effect on osteoblasts, whereas concentrations above 10−5 M appear to be toxic [Citation14]. In addition, Li et al. found that the minimum inhibitory concentration of the BP zoledronic acid (ZA) on mouse osteoclasts was 10−6 M [Citation15].
In order to achieve a more comprehensive understanding of how BPs affect the cells involved in osteogenesis, the concentration of the drug applied in vitro should ideally be as close to relevant therapeutic concentrations as possible. Accordingly, the in vitro dosage that promotes similar effects in osteoblasts as described in vivo would be the preferable. The aim of the present study was to delineate the effects of different concentrations of ALN on human osteoblasts in vitro, and relate this to in vivo effects of ALN in order to identify a therapeutically relevant concentration or range of concentrations for in vitro studies.
Materials and methods
Study design
Primary human osteoblasts at passage 4 from tibia of a one-day old female donor (Cambrex BioScience, Walkersville, MD, USA) were grown in Lonza Osteoblast Growth Media (OGM) (Cambrex BioScience), containing ascorbic acid, foetal calf serum and gentamicin. Cells were subcultured at 37 °C in a humidified atmosphere of 5% CO2 prior to confluence, according to manufacturers’ instructions.
Cells were seeded in 12-well plates and incubated with ALN (Sigma-Aldrich Biotechnology, Saint Louis, MO, USA) dissolved in OGM at concentrations of 5, 20 and 100 µM. For protein quantification, cells and cell culture media were harvested after 1, 3, 7 or 14 days of incubation, with the last change of medium with or without ALN 24 hrs prior to harvest. Unexposed cells at each time point were used as control. Cells and cell culture supernatants were collected and stored at −80 °C until analysis.
Cell viability and proliferation
Cell viability was confirmed by monitoring the activity of lactate dehydrogenase (LDH) in cell culture medium. LDH was measured using the microplate-based Cytotoxicity Detection Kit (LDH; Boehringer, Mannheim, Germany). In accordance with the manufacturers’ protocol, 50 µL aliquots of cell culture medium was used, and the absorbance was read using a microplate reader (Elx800, BioTek, Bad Friedrichshall, Germany) at 450 nm.
The proliferation rate of the cells was measured by [3H]-thymidine incorporation and the MTT colorimetric assay. In the [3H]-thymidine incorporation assay, the cells (1.7 × 104 cells/well in 12-well plates) were incubated with cell culture medium containing 5, 20 and 100 µM ALN for 1 and 3 days (n = 3). The cells were pulsed with 1 μCi [3H]-thymidine/well 12 h prior to harvest, and upon harvest, the medium was removed, and the cells were washed twice with PBS and twice with 5% trichloroacetic acid (TCA) to remove unincorporated [3H]-thymidine. The cells were solubilized in 500 μl of 1 M sodium hydroxide (NaOH), and 200 μL of the solubilized cell solution was transferred to 4 mL scintillation fluid (Lumagel LSC BV; GE Groningen, Netherlands) and counted for 3 min in a liquid scintillation counter (Packard 1900 TR, Packard Instruments, Meriden, CT, USA).
The MTT colorimetric Cell Growth Assay (CT02 Chemicon, Merck KGaA, Darmstadt, Germany) was performed according to manufactures’ instruction. Cells (4 × 103 cells/well in 96-well plates) were harvested after 3 days of incubation with the various concentrations of ALN (n = 3). Unexposed cells were used as control. The absorbance was measured using an ELISA plate reader (ELx800, BioTek, Vermont, USA) at a test wavelength of 570 nm with reference wavelength of 630 nm.
Protein quantification in cell culture medium
Multianalyte profiling was performed using the Luminex 200TM system (Luminex Corporation, Austin, TX, USA), and acquired fluorescence data were analysed by the xPONENT 3.1 software (Luminex).
Prior to analysis, aliquots of the cell culture medium were concentrated 5 times using Microsep Centrifugal tubes with 3 kDa cut-off (Pall Life Science, Ann Armour, MI, USA). A simultaneous quantification of 29 cytokine and chemokine biomarkers in 25 µL of cell culture media were ascertained using the 29-Milliplex Human Cytokine Immunoassay kit (Millipore, Billerica, MA, USA). The evaluated biomarkers included epidermal growth factor (EGF), eotaxin, granulocyte colony-stimulating factor (G-CSF), granulocyte-macrophage colony-stimulating factor (GM-CSF), interferon alpha-2 (IFN-a2), IFN-γ, interleukin-1a (IL-1a), IL-1b, IL-1ra, IL-2, IL-3, IL-4, IL-5, IL-6, IL-7, IL-8, IL-10, IL-12p40, IL-13, IL-15, IL-17, interferon gamma-induced protein 10 (IP-10), monocyte chemoattractant protein-1 (MCP-1), macrophage inflammatory protein-1a (MIP-1a), MIP-1b, tumour necrosis factor-a (TNF-a), TNF-b and vascular endothelial growth factor (VEGF). In addition, the levels of osteoprotegerin (OPG), osteocalcin (OC), leptin, TNF-a, sclerostin, and fibroblast growth factor 23 (FGF-23)) were simultaneously determined in 25 µL of cell culture media using Milliplex Human Bone Panel Immunoassay kit, HBNMAG-51K-7plex (Millipore).
Luminex Multiplex Bead Immunoassays are solid phase sandwich immunoassays, which are designed to be analysed with a Luminex instrument. All the reagents and tools needed were provided in the kit and analyses were performed according to the manufacturers’ protocols. In brief, in each of the above-described multiplex kits, 25 μl cell culture medium was applied in 96 cell plates, diluted with assay buffer, and incubated over night at 2–8 °C with antibody coated fluorescent magnetic beads. After washing, analyte-specific biotinylated detector antibodies are added and incubated with the beads. Excess biotinylated detector antibodies were removed by washing and streptavidin conjugated to the fluorescent protein, R-Phycoerythrin (Streptavidin-RPE), was added and the mixture incubated. After the final washing step acquired fluorescence data were quantified based on the spectral properties of the beads and the amount of associated R-Phycoerythrin (RPE) fluorescence in a Luminex 200TM. The concentration (pg/mL) of each biomarker in the samples was determined based on standard curves for each of the individual analytes in the kit.
Statistical analysis
Statistical evaluation was performed using the software SigmaPlot 13.0 and 14.0 (Systat Software, San Jose, CA, USA); statistical significance was assessed by Student’s t-test and p-value set to .05, given a passed test of normality and equality.
Results
Viability and proliferation
There was no significant difference in the cell viability (LDH) between the cultures after exposure to 5, 20 or 100 µM of ALN at any of the time points tested (). Evaluation of thymidine incorporation indicated that there was a marked reduction in proliferation of the osteoblasts after three days of exposure to 100 µM ALN compared to 5 and 20 µM ALN (p ≤ .001). This correlates with the findings in the MTT assay, where incubation with 100 µM ALN for three days resulted in a diminished proliferation compared to incubation with 5 and 20 µM ALN (p ≤ .001). Moreover, the MTT assay disclosed a reduced proliferation of the cells exposed to 20 µM ALN for three days, compared to 5 µM ALN (p ≤ .05) ().
Table 1. The impact of ALN on osteoblast viability (LDH) and proliferation ([3H]-thymidine and MTT).
The effect of ALN on secretion of biomarkers relevant to osteogenesis
As a consequence of the abolished proliferation after exposure to 100 μM ALN for 3 days, we chose to focus on 5 and 20 μM for the remainder of the study. Following an initial rise in secretion of sclerostin from cells exposed to 5 μM ALN (p = .008), there was no change in the concentration compared to control after 14 days (). Sclerostin secretion increased gradually up to 14 days in cells incubated with 20 μM ALN (p = .044), however, not significantly compared to 5 µM ALN exposed cells. The release of granulocyte colony-stimulating factor (G-CSF) was transiently enhanced after incubation with 5 μM ALN (p = .026 day 3 and p = .025 day 7) (). After 14 days of exposure to 20 μM ALN, G-CSF was reduced by 50% compared to the cells incubated with 5 μM ALN (p = .020) and unexposed cells (p = .017).
Figure 1. Sclerostin in cell culture media from human osteoblasts. The cells were exposed to 5 μM or 20 μM ALN. Data are presented in % relative to unexposed control at each time point. *p ≤ 0.05 compared to control, † p ≤ .05 compared to 5 μM.
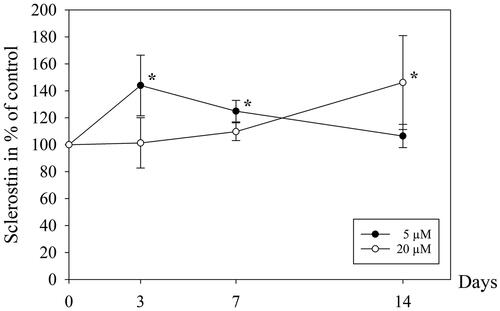
Figure 2. Granulocyte colony-stimulating factor (G-CSF) (a), vascular endothelial growth factor (VEGF) (b), monocyte chemoattractant protein (MCP-1) (c), interleukin-8 (IL-8) (d) and interferon-alpha2 (IFN-a2) (e) in cell culture media from human osteoblasts. The cells were exposed to 5 μM or 20 μM ALN. Data are presented in % relative to unexposed control at each time point. * p ≤ .05 compared to control, † p ≤ .05 compared to 5 μM.
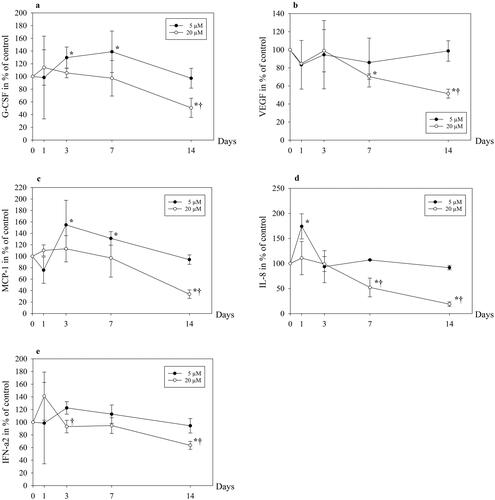
The effect of ALN on secretion of biomarkers relevant to angiogenesis
There was a non-significant initial reduction in the release of VEGF from osteoblasts after exposure to both 5 and 20 μM ALN. This was followed by an increase of VEGF to control level after 14 days of incubation with 5 μM ALN, and a reduction of VEGF by 50% in cells exposed to 20 μM ALN compared to 5 μM ALN (p = .003) and unexposed cells (p = .006) (). The secretion of MCP-1 rose to 155% of control after 3 days of incubation with 5 μM ALN (p = .019) (), with a subsequent decline to control level after 14 days. In cells exposed to 20 μM ALN, the level of MCP-1 was reduced by 70% compared to cells incubated with 5 μM after 14 days (p ≤ .001).
The effect of ALN on secretion of biomarkers relevant to inflammation
Compared to unexposed cells, there was a significant rise in the secretion of IL-8 after 1 day of exposure to 5 μM of ALN (p = .002) (). 20 μM ALN promoted a drop in IL-8 from day 3, resulting in 50% and 75% lower levels after 7 days (p = .007) and 14 days (p ≤ .001), respectively, compared to 5 μM. A transient non-significant increase in IFN-a2 was observed in cells incubated with 20 μM ALN (), thereafter IFN-a2 decreased, and was 30% lower after 3 days and 35% lower after 14 days when compared to 5 μM (p = .022 and .015, respectively).
Most of factors tested were not found to change significantly at any time point (leptin, OC, OPG, IFN-g, and IL-1ra), or the concentrations were lower than the set levels of detection for the analyses (FGF-23, EGF, eotaxin, IL-10, IL-12p40, IL-13, IL-15, IL-17, IL-1a, IL-1b, IL-2, IL-3, IL-4, IL-5, IL-6, IL-7, IP-10, MIP-1a, MIP-1b, TNF-a and TNF-b).
Discussion
In this study, exploring the in vitro effects of different concentrations of ALN on human osteoblasts, the viability was not affected by any of the dosages. Exposure to 5 µM ALN had a neutral effect on osteoblast proliferation, and on secretion of osteogenic and inflammatory markers, while enhancing synthesis of MCP-1, a marker of angiogenesis. 20 µM ALN induced a decline in proliferation and affected angiogenic and osteogenic biomarkers adversely after 14 days of exposure. ALN at a concentration of 100 µM reduced proliferation dramatically, and this dosage was excluded from further experiments.
Higher concentrations of ALN reduce proliferation
As previously mentioned, the positive skeletal effects of BPs are mainly mediated by suppression of osteoclastic activity. This is achieved through inhibition of the mevalonate pathway, ultimately leading to apoptosis of the osteoclasts [Citation16]. In vivo studies indicate that BPs also may affect osteoblasts and osteocytes [Citation17,Citation18]. As elaborated on in the introduction, osteoclasts are exposed to higher concentrations of BPs than cells of the osteoblastic lineage [Citation13]. Thus, when exploring the effects of BPs on osteoblasts in vitro, it is of importance to identify a dosage that is close to the in vivo concentration.
ALN in concentrations of 10−5 M or higher have been reported to affect osteoblasts adversely, inducing apoptosis and inhibiting cell differentiation [Citation19]. We observed no effect on viability after exposure to 5, 20 and 100 µM ALN for three days, as assessed by LDH activity. The effect on proliferation was, however, dose-dependent. In accordance with the findings of Garcia-Moreno et al. [Citation20], incubation with 100 μM ALN resulted in a steep decline in osteoblast proliferation after 3 days. The proliferation assessed with an MTT-assay was also reduced after 3 days of exposure to 20 μM ALN, compared to 5 μM. We did, however, not observe enhancement of osteoblast proliferation by 5 μM ALN. Probably an even lower dosage of ALN would be needed to exert this effect.
Notably, ALN has been shown to abolish the glucocorticoid-induced apoptosis in vertebral cancellous bone osteocytes and osteoblasts in mice [Citation21]. These findings suggest that the effect of BPs in glucocorticoid-induced osteoporosis and osteoporosis in general may be due, in part, to their ability to prevent osteocyte and osteoblast apoptosis [Citation3], this has also been confirmed in other studies [Citation22]. Preservation of the bone-forming function of mature osteoblasts and maintenance of the osteocytic network is decidedly of significance for normal function of bone. Thus, to mimic in vivo conditions, a dosage of 5 μM is clearly the better option.
Low dose of ALN has the least impact on biomarkers affecting osteogenesis
The Wnt signalling pathway is essential in bone formation, and bone homeostasis, as well as bone repair and regeneration following injury [Citation23]. This signalling pathway plays an important role in controlling the differentiation of mesenchymal stem cells (MSCs), in favour of the osteoblasts, as well as promoting osteoblast maturation and survival [Citation24]. Bone remodelling is constantly ongoing to replace old bone tissue by new bone tissue. Activation of the Wnt/β-catenin signalling pathway leads to increased proliferation and differentiation of osteoblast precursor cells, reduces apoptosis of mature osteoblasts, and promotes the ability of differentiated osteoblasts to inhibit osteoclast differentiation [Citation25]. Sclerostin, which is a protein predominantly secreted by osteocytes, is a potent antagonist to the Wnt pathway [Citation26], and as such potentially inhibits bone formation and stimulates bone resorption [Citation24]. We found that 5 μM ALN induced a temporary rise in secretion of sclerostin after 3 days of exposure, whereas 20 μM ALN induced a gradual rise in sclerostin throughout the duration of the experiment. In postmenopausal osteoporotic women treated with ZA, an early rise in sclerostin serum levels was observed [Citation27]. On the other hand, no significant alteration in circulating sclerostin levels was seen in a retrospective observational study after long-term treatment of postmenopausal women with the oral BPs ALN and risedronate [Citation28].
Furthermore, we found that ALN at a dosage of 5 μM promoted a transient increase in release of G-CSF from osteoblasts, followed by a decline to control level after 14 days. 20 μM ALN induced a pronounced decrease in G-CSF, with a 50% reduction after 14 days of exposure. A rise in G-CSF has also been reported in mice in response to a BP [Citation29]. G-CSF has been mainly associated with the recruitment of stem cells from bone marrow [Citation30,Citation31]. Effects on osteoclast and osteoblast activity have also been reported, the data are, however, diverging [Citation32–34]. The significance of our findings regarding G-CSF under in vivo conditions is uncertain.
Low dose of ALN promotes angiogenesis
The development of a microvasculature and microcirculation is critical for the homeostasis and regeneration of living bone, without which, the tissue would simply degenerate and die [Citation35,Citation36]. Thus, it is essential that drugs used in the treatment of osteoporosis do not inhibit angiogenesis. This complex process is orchestrated by multiple factors and mechanisms, which when in balance will contribute to insure a sufficient supply of nutrients and minerals. Osteoblasts have been shown to secrete VEGF, an essential angiogenic growth factor which is critical in the initial stages of wound healing and bone repair [Citation37]. ALN in the concentration range 10−5–10−3 M has been reported to enhance VEGF secretion from osteoblasts [Citation38], whereas 10−12–10−6 M of ZA and ALN reduced the VEGF secretion from osteoblastic cell lines in a dose-dependent manner [Citation19]. We observed no significant changes in VEGF secretion from osteoblasts exposed to 5 μM ALN, however a pronounced decline occurred after exposure to 20 μM ALN, which reduced VEGF by 50% compared to 5 μM ALN.
MCP-1 has been recognized as another important angiogenic chemokine, which is involved in induction of VEGF-A gene expression [Citation39]. We noted a substantial rise in MCP-1 after 3 days of incubation with 5 μM ALN, thereafter levelling off to control values after 14 days. In contrast, there was a marked drop in secretion of MCP-1 after 14 days of exposure to 20 μM ALN. Accordingly, our data indicate that ALN in a dosage of 5 μM could favour angiogenesis, whereas 20 μM seemed to affect it negatively.
5 μM Aln with marginal impact on inflammatory biomarkers after 14 days of exposure
The osteoblasts are capable of producing a wide range of cytokines and growth factors that are involved in bone damage and repair [Citation40,Citation41]. These substances include among others IL-1, IL-6, IL-8, TNF-a, IFN-a and IFN-g which interact in balancing bone metabolism [Citation42]. The expression of several proinflammatory cytokines from osteoblasts and osteoblast-like cells are affected by lower concentrations of BPs in vitro [Citation43,Citation44]. We have previously shown that 5 μM ALN stimulated release of proinflammatory cytokines [Citation45]. In the present study, an initial spike in the expression of IL-8 was seen after exposure to 5 μM ALN, whereas 20 μM induced a decline after 7 and 14 days. This is at odds with another study, showing that 100 μM ALN promoted an increase in the secretion of IL-8 [Citation46]. IL-8 has for decades been known as an important cytokine regarding development of inflammation, mainly through its activation of neutrophils [Citation47].
Release of IFN-a2 was also affected differently by the low and high dosages, 20 μM evoking an initial rise, followed by a decline, whereas the cells exposed to 5 μM ALN responded in a similar manner as control cells. The cytokine IFN-a2 is mainly accredited as an important factor in the immune response to a microbial infection [Citation48], but has also been applied in the treatment of several malignant diseases [Citation49]. Moreover, it has been suggested as an inhibitor of osteoclasts differentiation [Citation50].
In this study we have compared the effects of 5, 20 and 100 µM of ALN on osteoblasts in vitro, addressing effects on viability, proliferation, and the release of factors affecting inflammation, angiogenesis and osteogenesis. We intended to identify a therapeutically relevant concentration of ALN for in vitro studies on osteoblasts. Taken together, we observed that osteoblasts exposed to 5 μM ALN responded in a similar manner as control cells after 14 days, whereas the higher dosages negatively affected proliferation and factors regarding angiogenesis and osteogenesis. Therefore, it is reasonable to propose that ≤ 5 μM ALN in vitro are concentrations that best mimic observations made in vivo.
Osteonecrosis of the jaw (BRONJ) and atypical femur fractures are rare adverse effects of BPs. Under these circumstances, the concentration of ALN is probably higher. These adverse effects tend to occur after long-term treatment and subsequent accumulation of ALN in bone. Schaudinn et al. applied energy-dispersive X-ray spectroscopy (EDS) to estimate the concentrations of ALN in jaw bone by measurement of percent nitrogen incorporation [Citation51]. They observed that ALN concentrations correlated with both duration of therapy and BRONJ stage. Notably, in an inflammatory setting, peripheral blood mononuclear cells seem to increase their release of pro-inflammatory cytokines (TNF-a and IFN-g) as a response to internalizing BPs [Citation52]. Moreover, a local acidic environment, i.e. periodontitis, has been reported to amplify the dose-dependent cytotoxic effects of BPs, potentially triggering further osteolysis and an increased concentration of BPs [Citation53]. It should be recalled that there may be differences between individuals and between skeletal sites that affect the in vitro response to ALN. Hence, when conducting in vitro studies on primary human osteoblasts, both skeletal site of origin and donor age are variables of significance [Citation54].
Conclusion
With only marginal changes in viability, proliferation and secretion of factors of growth, angiogenesis and inflammation, incubation with 5 μM ALN had the least negative impact on the osteoblasts of the concentrations tested in this study. Altogether, based on the current available literature and our own findings, we suggest that ≤ 5 µM is a therapeutically relevant concentration of ALN for in vitro studies on primary human osteoblasts.
Acknowledgements
The authors would like to thank the staff at the Department of Oral Surgery and Oral Medicine, and at the Oral Research Laboratory for their patience, advice and support (Faculty of Dentistry, University of Oslo).
Disclosure statement
No potential conflict of interest was reported by the author(s).
References
- Black DM, Rosen CJ. Clinical practice. Postmenopausal osteoporosis. N Engl J Med. 2016;374(3):254–262.
- Barrionuevo P, Kapoor E, Asi N, et al. Efficacy of pharmacological therapies for the prevention of fractures in postmenopausal women: a network meta-Analysis. J Clin Endocrinol Metab. 2019;104(5):1623–1630.
- Plotkin LI, Weinstein RS, Parfitt AM, et al. Prevention of osteocyte and osteoblast apoptosis by bisphosphonates and calcitonin. J Clin Invest. 1999;104(10):1363–1374.
- Kaiser T, Teufel I, Geiger K, et al. Bisphosphonates modulate vital functions of human osteoblasts and affect their interactions with breast cancer cells. Breast Cancer Res Treat. 2013;140(1):35–48.
- Maruotti N, Corrado A, Neve A, et al. Bisphosphonates: effects on osteoblast. Eur J Clin Pharmacol. 2012;68(7):1013–1018.
- Jung J, Park JS, Righesso L, et al. Effects of an oral bisphosphonate and three intravenous bisphosphonates on several cell types in vitro. Clin Oral Investig. 2018;22(7):2527–2534.
- Acil Y, et al. Cytotoxic and inflammatory effects of alendronate and zolendronate on human osteoblasts, gingival fibroblasts and osteosarcoma cells. J Craniomaxillofac Surg. 2018;46(4):538–546.
- Turek J, Ebetino FH, Lundy MW, et al. Bisphosphonate binding affinity affects drug distribution in both intracortical and trabecular bone of rabbits. Calcif Tissue Int. 2012;90(3):202–210.
- Sato M, Grasser W, Endo N, et al. Bisphosphonate action. Alendronate localization in rat bone and effects on osteoclast ultrastructure. J Clin Invest. 1991;88(6):2095–2105.
- Drake MT, Clarke BL, Khosla S. Bisphosphonates: mechanism of action and role in clinical practice. Mayo Clin Proc. 2008;83(9):1032–1045.
- Fisher JE, Rosenberg E, Santora AC, et al. In vitro and in vivo responses to high and low doses of nitrogen-containing bisphosphonates suggest engagement of different mechanisms for inhibition of osteoclastic bone resorption. Calcif Tissue Int. 2013;92(6):531–538.
- Alakangas A, Selander K, Mulari M, et al. Alendronate disturbs vesicular trafficking in osteoclasts. Calcif Tissue Int. 2002;70(1):40–47.
- Clézardin P. Mechanisms of action of bisphosphonates in oncology: a scientific concept evolving from antiresorptive to anticancer activities. Bonekey Rep. 2013;2:267.
- Rogers MJ, Gordon S, Benford HL, et al. Cellular and molecular mechanisms of action of bisphosphonates. Cancer. 2000;88(S12):2961–2978.
- Li P, Zhao Z, Wang L, et al. Minimally effective concentration of zoledronic acid to suppress osteoclasts in vitro. Exp Ther Med. 2018;15(6):5330–5336.
- Luckman SP, Hughes DE, Coxon FP, et al. Nitrogen-containing bisphosphonates inhibit the mevalonate pathway and prevent post-translational prenylation of GTP-binding proteins, including ras. J Bone Miner Res. 1998;13(4):581–589.
- Plotkin LI, Buvinic S, Balanta-Melo J. In vitro and in vivo studies using non-traditional bisphosphonates. Bone. 2020;134:115301.
- Koyama C, Hirota M, Okamoto Y, et al. A nitrogen-containing bisphosphonate inhibits osteoblast attachment and impairs bone healing in bone-compatible scaffold. J Mech Behav Biomed Mater. 2020;104:103635.
- Ishtiaq S, Edwards S, Sankaralingam A, et al. The effect of nitrogen containing bisphosphonates, zoledronate and alendronate, on the production of pro-angiogenic factors by osteoblastic cells. Cytokine. 2015;71(2):154–160.
- García-Moreno C, Serrano S, Nacher M, et al. Effect of alendronate on cultured normal human osteoblasts. Bone. 1998;22(3):233–239.
- Plotkin LI, Bivi N, Bellido T. A bisphosphonate that does not affect osteoclasts prevents osteoblast and osteocyte apoptosis and the loss of bone strength induced by glucocorticoids in mice. Bone. 2011;49(1):122–127.
- Bellido T, Plotkin LI. Novel actions of bisphosphonates in bone: preservation of osteoblast and osteocyte viability. Bone. 2011;49(1):50–55.
- Xu H, Duan J, Ning D, et al. Role of wnt signaling in fracture healing. BMB Rep. 2014;47(12):666–672.
- Delgado-Calle J, Sato AY, Bellido T. Role and mechanism of action of sclerostin in bone. Bone. 2017;96:29–37.
- Baron R, Kneissel M. WNT signaling in bone homeostasis and disease: from human mutations to treatments. Nat Med. 2013;19(2):179–192.
- Poole KES, van Bezooijen RL, Loveridge N, et al. Sclerostin is a delayed secreted product of osteocytes that inhibits bone formation. Faseb J. 2005;19(13):1842–1844.
- Catalano A, Morabito N, Basile G, et al. Zoledronic acid acutely increases sclerostin serum levels in women with postmenopausal osteoporosis. J Clin Endocrinol Metab. 2013;98(5):1911–1915.
- Chung YE, Lee SH, Lee S-Y, et al. Long-term treatment with raloxifene, but not bisphosphonates, reduces circulating sclerostin levels in postmenopausal women. Osteoporos Int. 2012;23(4):1235–1243.
- Otsuka H, Yagi H, Endo Y, et al. Nitrogen-containing bisphosphonate induces a newly discovered hematopoietic structure in the omentum of an anemic mouse model by stimulating G-CSF production. Cell Tissue Res. 2017;367(2):297–309.
- Tay J, Levesque JP, Winkler IG. Cellular players of hematopoietic stem cell mobilization in the bone marrow niche. Int J Hematol. 2017;105(2):129–140.
- Petit I, Szyper-Kravitz M, Nagler A, et al. G-CSF induces stem cell mobilization by decreasing bone marrow SDF-1 and up-regulating CXCR4. Nat Immunol. 2002;3(7):687–694.
- Roseren F, et al. Systemic administration of G-CSF accelerates bone regeneration and modulates mobilization of progenitor cells in a rat model of distraction osteogenesis. Int J Mol Sci. 2021;22(7):3505.
- Yu H, Zhang T, Lu H, et al. Granulocyte colony-stimulating factor (G-CSF) mediates bone resorption in periodontitis. BMC Oral Health. 2021;21(1):299.
- Christopher MJ, Link DC. Granulocyte colony-stimulating factor induces osteoblast apoptosis and inhibits osteoblast differentiation. J Bone Miner Res. 2008;23(11):1765–1774.
- Filipowska J, Tomaszewski KA, Niedźwiedzki Ł, et al. The role of vasculature in bone development, regeneration and proper systemic functioning. Angiogenesis. 2017;20(3):291–302.
- Grosso A, Burger MG, Lunger A, et al. It takes two to Tango: coupling of angiogenesis and osteogenesis for bone regeneration. Front Bioeng Biotechnol. 2017;5:68.
- Hu K, Olsen BR. The roles of vascular endothelial growth factor in bone repair and regeneration. Bone. 2016;91:30–38.
- Manzano-Moreno FJ, Ramos-Torrecillas J, Melguizo-Rodríguez L, et al. Bisphosphonate modulation of the gene expression of different markers involved in osteoblast physiology: possible implications in bisphosphonate-related osteonecrosis of the jaw. Int J Med Sci. 2018;15(4):359–367.
- Hong KH, Ryu J, Han KH. Monocyte chemoattractant protein-1-induced angiogenesis is mediated by vascular endothelial growth factor-A. Blood. 2005;105(4):1405–1407.
- Murtaugh MP, Baarsch MJ, Zhou Y, et al. Inflammatory cytokines in animal health and disease. Vet Immunol Immunopathol. 1996;54(1–4):45–55.
- Loi F, Córdova LA, Pajarinen J, et al. Inflammation, fracture and bone repair. Bone. 2016;86:119–130.
- Schett G. Effects of inflammatory and anti-inflammatory cytokines on the bone. Eur J Clin Invest. 2011;41(12):1361–1366.
- Giuliani N, et al. Bisphosphonates inhibit IL-6 production by human osteoblast-like cells. Scand J Rheumatol. 1998;27(1):38–41.
- Giannasi C, Niada S, Farronato D, et al. Nitrogen containing bisphosphonates impair the release of bone homeostasis mediators and matrix production by human primary pre-osteoblasts. Int J Med Sci. 2019;16(1):23–32.
- Krüger TB, Herlofson BB, Lian AM, et al. Alendronate and omeprazole in combination reduce angiogenic and growth signals from osteoblasts. Bone Rep. 2021;14:100750.
- Krüger TB, Herlofson BB, Landin MA, et al. Alendronate alters osteoblast activities. Acta Odontol Scand. 2016;74(7):550–557.
- Rajarathnam K, Sykes BD, Kay CM, et al. Neutrophil activation by monomeric interleukin-8. Science. 1994;264(5155):90–92.
- Paul F, Pellegrini S, Uzé G. IFNA2: the prototypic human alpha interferon. Gene. 2015;567(2):132–137.
- Antonelli G, Scagnolari C, Moschella F, et al. Twenty-five years of type I interferon-based treatment: a critical analysis of its therapeutic use. Cytokine Growth Factor Rev. 2015;26(2):121–131.
- Avnet S, Cenni E, Perut F, et al. Interferon-alpha inhibits in vitro osteoclast differentiation and renal cell carcinoma-induced angiogenesis. Int J Oncol. 2007;30(2):469–476.
- Schaudinn C, Gorur A, Webster P, et al. Quantification by energy dispersive x-ray spectroscopy of alendronate in the diseased jaw bone of patients with bisphosphonate-related jaw osteonecrosis. Oral Surg Oral Med Oral Pathol Oral Radiol. 2012;114(4):480–486.
- Clézardin P. Bisphosphonates' antitumor activity: an unravelled side of a multifaceted drug class. Bone. 2011;48(1):71–79.
- Otto S, Pautke C, Opelz C, et al. Osteonecrosis of the jaw: effect of bisphosphonate type, local concentration, and acidic milieu on the pathomechanism. J Oral Maxillofac Surg. 2010;68(11):2837–2845.
- Martínez ME, del Campo MT, Medina S, et al. Influence of skeletal site of origin and donor age on osteoblastic cell growth and differentiation. Calcif Tissue Int. 1999;64(4):280–286.