Abstract
Nanoparticles have been recognized as an abiotic stress factor in the last decade. Although they are extensively used in nanotechnology, the possible effects of nanoparticles on plants are still unclear. Therefore the aim of the present study was to determine the dose dependent effects of 13 nm-sized aluminum oxide nanoparticles (Al2O3 NPs) on wheat roots correlating with programmed cell death related characteristics. Exposure to different concentrations of Al2O3 NPs (5, 25 and 50 mg ml–1) decreased the mitotic indices dose dependently and caused chromosomal abnormalities such as c-mitosis, monopolar metaphase and stickiness after 96 h. Loss of plasma membrane integrity and irregular microtubule aggregations were determined. Nuclear deformations and TUNEL positive reactions indicating programmed cell death were also observed. Al2O3 NP-induced caspase 3, 8 and 9-like activities which control programmed cell death were observed in all concentrations. According to our results Al2O3 NPs induced programmed cell death in wheat roots after 96 h.
1. Introduction
Programmed cell death (PCD) is a conserved and genetically regulated process responsible for the elimination of undesirable and injured cells in multicellular eukaryotes. In plants, PCD has a critical role in the control of developmental processes as well as environmental stress responses (Gunawardena, Citation2008). Developmentally regulated PCD occurs in various types of plant tissues and organs, such as embryonic suspensors, anther tapeta, nucelli, synergids, antipodals, as well as tracheal elements, root caps and trichomes and during leaf and flower senescence (Yen and Yang Citation1998; Fukuda Citation2000; Rogers Citation2005; Brighigna et al. Citation2006; Bar-Dror et al. Citation2011; Papini et al. Citation2010, Citation2011; Vardar and Ünal Citation2012 Bartoli et al. Citation2016). Stress-induced PCD is triggered by biotic and abiotic stimuli such as pathogen attack, heat, cold, ultraviolet (UV) light, pesticides and heavy metals (Wang et al. Citation1996; Greenberg Citation1996; Cho and Seo Citation2005; Gechev et al. Citation2006; Lord and Gunawardena Citation2011; Nawkar et al. Citation2013). PCD is essential for plant development and survival, and has common cellular responses such as shrinkage of cytoplasm, cytoskeletal disorder, reduction of mitochondrial membrane potential, release of cytochrome c from mitochondria, chromatin condensation, DNA fragmentation and caspase-like activities (Piszczek and Gutman Citation2007; Gadjev et al. Citation2008; Aytürk and Vardar Citation2014; Vardar et al. Citation2016).
In the last decade, nanoparticles (NPs) have been recognized as an abiotic stress factor for living organisms (Miralles et al. Citation2012). NPs are described as being materials less than 100 nm in diameter (Roco Citation2003). Their physico-chemical properties, such as their chemical, electrical, magnetic, and optical features, are due to their surface characteristics and high reactivity (Nel et al. Citation2006; Remédios et al. Citation2012). It has been predicted that the rapid development of nanotechnology will cause an increase of their presence in the environment in the near future.
Therefore, increased production and use of nanomaterials may lead to the emergence of a new type of waste (nano-waste) (Bystrzejewska-Piotrowska et al. Citation2009). Accumulation of NPs in the aquatic, terrestrial, and atmospheric environment has the possibility to cause a new potential problem to the environment (Asztemborska et al. Citation2015). In industry, aluminum oxide (Al2O3) NPs are one of the most commonly used NPs in the production of cosmetics, cutting tools, packaging materials, glass-metal and plastic products (Hanemann and Szabó Citation2010; Miralles et al. Citation2012).
Considering the rapid environmental dispersion and accumulation of NPs, most living organisms are under risk. Although a number of researchers are examining the possible comparative effects of Al2O3 NPs on animals (Kim et al. Citation2009; Di Virgilio et al. Citation2010), studies are limited on the mechanisms of NP toxicity in plants, which are sessile organisms, closely related to the substrate, and necessarily affected by these emerging contaminants (Ruffini Castiglione et al. Citation2014). Besides rare reports can be attainable exhibiting the relation between NP and PCD.
The objective of this study is to evaluate the dose dependent effects of 13 nm-sized Al2O3 NPs on wheat correlating with the PCD related characteristics.
2. Material and methods
2.1. Plant material and seedling growth
Wheat (Triticum aestivum L. cv Demir 2000) seeds were obtained from the Central Research Institute For Field Crops (Ankara, Turkey). Seeds were surface-sterilized and germinated on moistened filter paper in Petri dishes under fluorescent tubes giving an irradiance of 5000 lux (day/night 16:8), temperature of 23 ± 2°C, and relative humidity of 45–50%. The germinated seeds, which reached 0.5–1 cm root elongation, were treated with different doses of 13 nm-sized Al2O3 NPs (5, 25 and 50 mg ml–1) for 96 h at room temperature. Different doses of Al2O3 NPs were diluted from a stock solution (100 mg ml–1) of commercial Al2O3 nanopowder (Sigma-Aldrich, 718475, St Louis, MO, USA) by dispersing NPs through ultrasonication (230 V/50–60 Hz) in distilled water for 30 min. Distilled water was used for the control group. For each independent experimental group, 30 seeds were used. All of the analyses were experienced with three replicates. Analysis of variance of all the experimental data was performed with SPSS 21.0. One-way analyses of variance (ANOVA) with Tukey’s post hoc HSD were done. The mean differences are significant at p<0.05 levels.
2.2. Root meristem activity and chromosome behavior
The control and Al2O3 NP-treated roots were fixed in acetic-alcohol (1:3, v/v) for 24 h and stored in 70% alcohol at + 4°C for further use. The root tips were hydrolyzed in 1 N HCI at 60°C for 20 min and transferred to basic fuchsin for 2 h in the dark. Squash preparations were performed in 2% aceto-orcein. Randomly selected areas on the slides were monitored to detect the number of dividing cells, chromosome abnormalities and micronuclei formation. In control and treated groups, 10 well-spread preparations were selected and approximately 1100 cells were scored. The mitotic index (MI) was calculated as the ratio of the number of dividing cells to the total number of cells, multiplied by 100. Meanwhile the frequency of chromosome abnormalities and micronuclei was calculated as the number of aberrant cells per 100 cells examined. The preparations were photographed with the Kameram software (Argenit, Istanbul, Turkey), assisted by a Kameram digital camera (Argenit, Istanbul, Turkey) and an Olympus BX-51 light microscope (Argenit, Istanbul, Turkey).
2.3. Determination of loss of plasma membrane integrity
The loss of plasma membrane integrity was evaluated by Evans blue staining method in control and Al2O3 NP-treated roots (Pandey et al. Citation2013). The intact barley roots were rinsed in running tap water and stained with 0.25% (w/v) Evans blue solution for 30 min. After rinsing the excess dye in distilled water for 10 min, 10 root tips of equal length (10 mm) were excised. The control and treated roots were immersed in 1 ml of 1% (w/v) sodium dodecyl sulfate (SDS) solution for 15 min. The solutions were centrifuged at 13,500 g for 10 min and the supernatant was used for measurement at 600 nm spectrophotometrically.
2.4. Determination of nuclear morphology and DNA fragmentation
The control and Al2O3 NP-treated roots were fixed by 4% paraformaldehyde in 0.1 M phosphate buffer (PBS-pH 7.0) for 24 h and stored in 70% alcohol at + 4°C for further analyses. In order to determine alterations in nucleus morphology induced by Al2O3 NPs, 4',6-Diamidine-2' phenylindole dihydrochloride (DAPI) staining was performed according to Schweizer (Citation1976). The fixed roots were stained with 1 μg ml–1 DAPI for 1 h in the dark. Samples were rinsed with PBS and root squash preparations were made in 45% acetic-acid.
TUNEL (terminal deoxynucleotidyl transferase (TdT)-mediated dUTP nick end labeling) reaction is the labelling of free 3′OH termini that identifies the DNA strand breaks. The fixed roots were dehydrated and embedded in paraffin. The cross sections of roots were cut at 6–8 μm thick. The TUNEL reaction was performed using the ApopTag® Plus Fluorescein In situ Apoptosis Detection kit according to the manufacturer’s manual (Chemicon, Temecula, CA, USA).
2.5. Microtubule immunostaining
Wheat roots were processed for immunostaining of microtubules using a modified method from Kumagai et al. (Citation2001). The fixed roots were rinsed in 50 mM PBS (pH 6.8) for 30 min. Cell walls were digested with 0.5% cellulose (25 min), 2% driselase (15 min) and 1% cellulysin (16 min) dissolved in 0.4 M mannitol, respectively. After digestion roots were squashed on poly-L-lysine coated slides. The air-dried samples were permeabilized in ice-cold methanol for 10 min and rehydrated in buffer containing 1% Triton X-100, 0.1 M PİPES, 2 mM EGTA, 1 mM MgSO4 and 0.4 M mannitol (pH 6.9) for 30 min. Samples were incubated with rabbit anti-α-tubulin antibody (IgG, Sigma-Aldrich) diluted 1/50 in buffer for 1 h at 37°C in a dark moist chamber. Samples were rinsed with buffer and then incubated with fluorescein Goat Anti-Rabbit IgG (Sigma-Aldrich) diluted 1/24 at 37°C for 30 min. After removing the secondary antibody, slides were washed with buffer three times for 2 min and mounted with 1.4-diazabisiklo-(2,2,2) (DABCO).
All of the stained roots cells were analyzed and photographed with KAMERAM software, assisted by a KAMERAM fluorescent camera and an Olympus BX-51 fluorescence microscope.
2.6. Determination of caspase-like activities
According to Lombardi et al. (Citation2007), control and Al2O3 NP-treated root tips were homogenized with cold extraction buffer (50 mM HEPES-KOH – pH 7, 10% sucrose, 0.1% CHAPS, 5 mM DTT, and 1 mM EDTA). The homogenates were kept on ice for 10 min and centrifuged at 14,000 rpm (+4°C) for 10 min. Protein concentration was determined at 280 nm using a nanophotometer (Implen). Caspase-3, -8 and -9 like activities were evaluated with using Caspase Colorimetric Activity Assay Kit (Chemicon) with p-NA (p-nitroaniline)-labeled substrates DEVD, IETD and LEHD, respectively. Equal amounts of protein extracts were incubated at 37°C for 2 h with substrates in accordance with manufacturer’s instructions. The enzyme activities were measured at 405 nm with a microtiter plate. Comparison of the absorbance of treated group with the control allows detection of the fold increase in caspase-like activity.
3. Results
To evaluate the programmed cell death induced by 13 nm-sized aluminum oxide nanoparticles (Al2O3 NPs), cytological and biochemical analyses were performed after 96 h treatment in wheat roots. The mitotic indices (MI) of control and Al2O3 NP (5, 25 and 50 mg ml–1) treated wheat roots are presented in Table . The frequency of dividing cells in the wheat root tips was found to decrease with increasing doses. The MI was 20.94% in the control, and 15.90, 13.15, and 12.82% in 5, 25 and 50 mg ml–1 treated plants, respectively. The reduction in mitotic activity was more efficient in the highest concentration, 50 mg ml–1. In addition, Al2O3 NP treatments induced micronuclei formation and chromosomal abnormalities (Table , Figure ). The most often encountered Al2O3 NP effect was micronuclei formation which occurs mostly from chromosomal aberrations. Although chromosomal abnormalities such as c-mitosis and monopolar metaphase were common in all Al2O3 NP treatments, stickiness was only observed in the 25 mg ml–1 treatment. In monopolar metaphase, monopolar mitotic spindles were generated and chromosomes lined up in a metaphase-like position causing abnormal chromosome segregation. Additionally, inhibition of spindle formation caused c-mitosis in which chromosomes dispersed to cytoplasm in metaphase. Sticky metaphase, which was observed only in the 25 mg ml–1 treatment, constituted sticky and clustered chromosomes aligning in the equator of the cell. The ratios of total abnormalities were 9.6, 20.3, and 9.4‰ in 5, 25, and 50 mg ml–1 treatments, respectively.
Table 1. The effects of Al2O3 NP (5, 25 and 50 mg ml–1) on the mitotic index, chromosome abnormalities and micronuclei in wheat roots after 96 h. The data with different letter are significantly different according to Tukey’s post-hoc HSD test for independent samples at p < 0.05. Values represent means ± SD.
Figure 1. Micronuclei and chromosomal abnormalities in Al2O3 NP (5, 25 and 50 mg ml–1) treated wheat roots after 96 h. (a) Micronuclei in the 5 mg ml–1 (arrows) treatment; (b) C-mitosis in the 25 mg ml–1 treatment; (b) stickiness in the 25 mg ml–1 treatment; (c) monopolar metaphase in the 25 mg ml–1 treatment; (d) monopolar metaphase in the 50 mg ml–1 treatment. Scale bar = 10 μm.
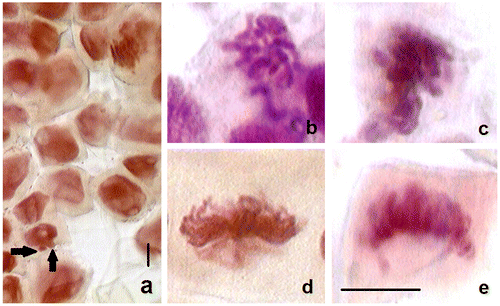
Considering the chromosomal abnormalities such as monopolar metaphase and c-mitosis in relation to spindle defects, immunostaining of microtubule structures was performed. Based on our results α-tubulin spots were recorded in the Al2O3 NP-treated cells. These spots were significantly accumulated in irregular aggregations in 25 and 50 mg ml–1 treatments (Figure ).
Figure 2. Immunostaining of microtubule structures in control and Al2O3 NP (5, 25 and 50 mg ml–1) treated wheat roots after 96 h. (a) Control; (b) 5 mg ml–1; (c) 25 mg ml–1; (d) 50 mg ml–1. Arrows show microtubule disruption. Scale bar = 50 μm.
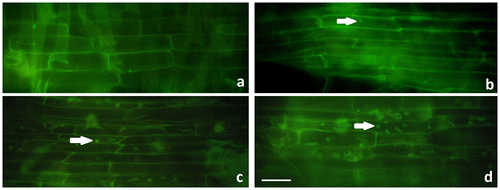
Loss of plasma membrane integrity, which is one of the important signals of cell death, was evaluated by Evans blue assay. The dye uptake by deformed membranes was increased approximately twofold in all Al2O3 NP concentrations (Figure ).
Figure 3. Loss of plasma membrane integrity in control and Al NP-treated wheat roots after 96 h. All data are significantly different from the control at the p < 0.05 level.
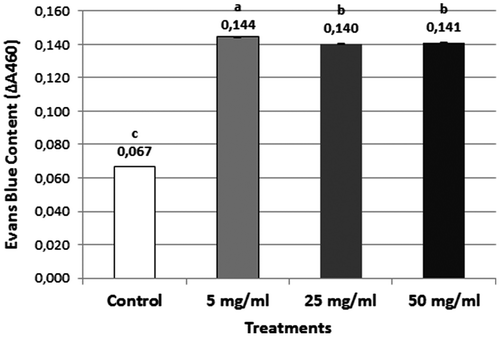
Nucleus deformations regarding to programmed cell death (PCD) were also observed in Al2O3 NP-treated wheat root cells labeled by DNA fluorochrome DAPI after 96 h (Figure ). The spherical nuclei of root cells emitted bright blue fluorescence and the chromatin was dispersed regularly. After 5 mg ml–1 Al2O3 NP treatment, nuclei lost their spherical shape and some invaginations were observed. In 25 and 50 mg ml–1 Al2O3 NP treatments; nuclear disintegration and extruded chromatin material from the nucleus were also detected. Furthermore nucleus morphology was completely disrupted in 50 mg ml–1.
Figure 4. Control and Al2O3 NP (5, 25 and 50 mg ml–1) treated wheat root cells stained with DAPI. (a) Spherical nucleus in control. (b–d) Nucleus degenerations (arrow) in (b) the 5 mg ml–1 treatment; (c) the 25 mg ml–1 treatment; and (d) the 50 mg ml–1 treatment. Scale bar = 10 μm.
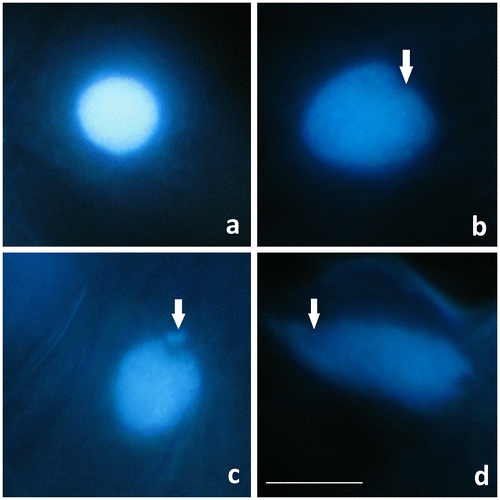
The TUNEL reaction, which labels the 3′OH ends of DNA strand breaks, indicates the existence of intra-nucleosomal DNA cleavage causing PCD. Although TUNEL staining was negative in control roots, the TUNEL positive reaction was significant in the nuclei of the wheat root cells in all concentrations of Al2O3 NP treatments after 96 h (Figure ).
Figure 5. TUNEL staining of control and Al2O3 NP (5, 25 and 50 mg ml–1) treated wheat roots after 96 h. a. Control (no reaction), b. 5 mg ml–1 c. 25 mg ml–1 d. 50 mg ml–1. Arrows show TUNEL positive nuclei. Scale bar = 50 μm.
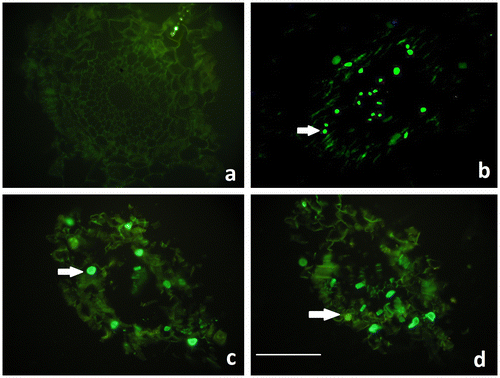
It is widely known that caspase-3, -8 and -9 are responsible for the apoptotic pathway in animals. Although there was no caspase-3 like activities at 5 mg ml–1, caspase-8 and -9 like activities were significant. According to the results caspase-3, -8 and -9 like activities increased in the 25 mg ml–1 treatment, suggesting intensive cell death. In the 50 mg ml–1 treatment caspase-8 and -9 lost their intensity, suggesting that the cell death process was proceeding to the advanced stage. However caspase-3 like activities were still intense (Figure ).
4. Discussion
Programmed cell death (PCD) is a functional process, which occurs as a defensive strategy to remove mutated, infected or damaged cells during development or under environmental stress (Vardar and Ünal Citation2011; Wang et al. Citation2011). Therefore we aimed to study possible toxic effects leading to PCD in wheat root cells following 13 nm-sized Al2O3 NP treatment.
Mitotic index (MI) is an important parameter which indicates the frequency of cell division. It is also an alternative method to correlate growth inhibition, genotoxic effects and cell death (Yumurtacı et al. Citation2007; Sobieh et al. Citation2016). In the present study the MI decreased dose dependently after Al2O3 NP treatment. In addition, micronuclei and chromosomal aberrations such as c-mitosis, stickiness and monopolar metaphase were observed. Considering all the Al2O3 NP concentrations the highest ratio of abnormalities was monitored in the 25 mg ml–1 treatment. To the best of our knowledge no previous data have been reported concerning effects of Al2O3 NP treatment on mitotic index and chromosomal abnormalities. However, Ruffini Castiglione et al. (Citation2011) revealed chromosomal abnormalities and reduction of MI in root cells of Zea mays and Vicia norbonensis after short-term exposure of TiO2 NPs. Similarly mitotic abnormalities, chromosome aberrations and micronucleus formation were also presented in Allium cepa roots after chitosan-capped Ag NP (Pesnya Citation2013) and Ag NP (Sobieh et al. Citation2016) treatments. Previously Yanık and Vardar (Citation2015) reported that Al2O3 NP treatment inhibited the root elongation in wheat, time and dose dependently. With respect to our results, the inhibition of root elongation in wheat (Yanık and Vardar Citation2015) may be a consequence of MI reduction and chromosomal abnormalities.
After multiple types of environmental stress factors the evaluated chromosomal abnormalities may be caused by structural or spindle abnormalities (Nilan and Vig Citation1976). In the present study frequently observed c-mitosis and monopolar metaphases may be considered as defects in mitotic spindles. As evidenced by the immunostaining of microtubule structures, Al2O3 NP treatment caused irregular aggregations in the wheat root cells. Although there is no available information about microtubule-NP interaction in plants, much attention has been paid to the regulation of the actin and microtubule cytoskeletal network in animal and human cell cultures. The common knowledge is that NP treatment alters the dynamic structure of cytoskeletal network including actin and tubulin, as we monitored (Gonzalez et al. Citation2015; Mao et al. Citation2015).
According to our results Al2O3 NP treatment induced significant cellular changes that were recognized as signs of PCD. Loss of plasma membrane integrity, which is one of the advanced signs of PCD, was determined in all concentrations. It has been known that stress conditions induce generation of excess reactive oxygen species (ROS). Increased production of ROS leads to oxidative stress and affects biomolecules such as proteins, carbohydrates, DNA and lipids. Formation of lipid peroxides leads to changes in the permeability of the plasma membrane and Evans blue stain accumulates in the cell (Poborilova et al. Citation2013). Nuclear changes and DNA fragmentations are the most widely evaluated and sensitive parameters of PCD (Vardar et al. Citation2016). DAPI and TUNEL assay confirmed nuclear disintegration, chromatin extrusion and DNA strand breaks after Al2O3 NP treatment.
PCD is a type of controlled cell breakdown and occurs with the contribution of caspases (cysteine-containing aspartate-specific proteases) in animals (Woltering et al. Citation2002). No functional homologs of animal caspases have been characterized in plants. However, caspase-like activities with special plant proteases such as metacaspases and vacuolar processing enzymes have been detected in plants during development and stress (Vardar and Ünal Citation2008; Wang et al. Citation2012; Kang et al. Citation2013). Multiple experiments representing caspase-like activities in plants can be evaluated by using caspase substrates and inhibitors from animal systems (Del Pozo and Lam Citation1998; Aytürk and Vardar Citation2014). In detailed studies it has been revealed that caspase-3, -8 and -9 are located at critical positions in animal apoptotic pathway (Lockshin et al. Citation1998; McIlwain et al. Citation2013). While the extrinsic apoptotic pathway is started by caspase-8 activation, the intrinsic (mitochondrial) pathway is started by caspase-9 activation (Shiozaki et al. Citation2002; Samraj et al. Citation2006). Furthermore, both caspase-8 and caspase-9 activate caspase-3, which causes chromatin condensation and DNA fragmentation (Riedl and Shi Citation2004; Cullen and Martin Citation2009). In the present study caspase-3, -8 and -9 like activities were detected. It can be suggested that Al2O3 NP treatment triggered both intrinsic and extrinsic cell death pathways and caspase-3 like activities confirm DNA fragmentation revealed by TUNEL. PCD signs after Al2O3 NP (<50 nm particle size) treatment were previously mentioned in the tobacco BY-2 cell suspension culture determined by Evans blue assay, TUNEL assay and caspase-3 like activity (Poborilova et al., Citation2013). The distinction of our study is that we obtained our results from intact roots which are more resistant to stress than cell lines.
5. Conclusion
It is expected that nanotechnology will be an important technology in the future (Chinnamuthu and Boopathi Citation2009). In the long term the possible toxic effects of nanoparticles on aquatic, terrestrial, and atmospheric environment are still unclear. For this reason, detailed studies on biochemical, physiological and molecular effects of nanoparticles in living organisms are critically needed (Asztemborska et al. Citation2015). The presented study aimed to reveal PCD evidences after Al2O3 NP induction. Al2O3 NPs caused inhibition of MI, chromosomal abnormalities, loss of plasma membrane integrity, nuclear dissolution, internucleosomal fragmentation and caspase -3, -8 and -9 like activities leading to cell death, briefly. NP-induced PCD responses should be under consideration, for protection of living organisms and environment. Physiological and molecular characterization of potential impacts of NPs will lead in the future to a better understanding of the mechanisms of NP toxicity and tolerance in environmental and agricultural systems.
Funding
This study is supported by Research Foundation of Marmara University (BAPKO) [grant number FEN-A-110915-0431].
Disclosure statement
No potential conflict of interest was reported by the authors.
References
- Asztemborska M, Steborowski R, Kowalska J, Bystrzejewska-Piotrowska G. 2015. Accumulation of aluminium by plants exposed to nano- and microsized particles of Al2O3. Int J Environ Res. 9(1):109–116.
- Aytürk Ö, Vardar F. 2014. Aluminum-induced caspase-like activities in some Gramineae species. Adv Food Sci. 37(2):71–75.
- Bar-Dror T, Dermastia M, Kladnik A, Znidaric MT, Novak MP, Meir S, Burd S, Philosoph-Hadas S, Ori N, Sonego L, et al. 2011. Programmed cell death occurs asymmetrically during abscission in tomato. Plant Cell. 23(11):4146–4163. 10.1105/tpc.111.092494
- Bartoli G, Felici C, Ruffini Castiglione M. 2016. Female gametophyte and embryo development in Helleborus bocconei Ten. (Ranunculaceae). Protoplasma. 254(1):491–504. 10.1007/s00709-016-0969-8
- Brighigna L, Milocani E, Papini A, Vesprini JL. 2006. Programmed cell death in the nucellus of Tillandsia (Bromeliaceae). Caryologia. 59(4):334–339. 10.1080/00087114.2006.10797935
- Bystrzejewska-Piotrowska G, Golimowski J, Urban L. 2009. Nanoparticles: their potential toxicity, waste and environmental management. Waste Manag. 29(9):2587–2595. 10.1016/j.wasman.2009.04.001
- Chinnamuthu CR, Boopathi PM. 2009. Nanotechnology and agroecosystem. Madras Agric J. 96(1–6):17–31.
- Cho UH, Seo NH. 2005. Oxidative stress in Arabidopsis thaliana exposed to cadmium is due to hydrogen peroxide accumulation. Plant Sci. 168(1):113–120. 10.1016/j.plantsci.2004.07.021
- Cullen SP, Martin SJ. 2009. Caspase activation pathways: some recent progress. Cell Death Differ. 16(7):935–938. 10.1038/cdd.2009.59
- Del Pozo O, Lam E. 1998. Caspases and programmed cell death in the hypersensitive response of plants to pathogens. Curr Biol. 8(20):1129–1132. 10.1016/S0960-9822(98)70469-5
- Di Virgilio AL, Reigosa M, Arnal PM, Lorenzo F, de Mele M. 2010. Comparative study of the cytotoxic and genotoxic effects of titanium oxide and aluminium oxide nanoparticles in Chinese hamster ovary (CHO-K1) cells. J Hazard Mater. 177(1–3):711–718. 10.1016/j.jhazmat.2009.12.089
- Fukuda H. 2000. Programmed cell death of tracheary elements as a paradigm in plants. Plant Mol Bio. 44(3):245–253. 10.1023/A:1026532223173
- Gadjev I, Stone JM, Gechev TS. 2008. Programmed cell death in plants: new insights into redox regulation and the role of hydrogen peroxide. Int Rev Cell Mol Bio. 270:87–144. 10.1016/S1937-6448(08)01403-2
- Gechev TS, Van Breusegem F, Stone JM, Denev I, Laloi C. 2006. Reactive oxygen species as signals that modulate plant stress responses and programmed cell death. Bioassays. 28(11):1091–1101. 10.1002/(ISSN)1521-1878
- Gonzalez L, Puzzonia MS, Raffaele Ricci R, Aureli F, Guarguaglini G, Cubadda F, Leyns L, Cundari E, Kirsch-Volders M. 2015. Amorphous silica nanoparticles alter microtubule dynamics and cell migration. Nanotoxicology. 9(6):729–736. 10.3109/17435390.2014.969791
- Greenberg JT. 1996. Programmed cell death: a way of life for plants. PNAS. 93(22):12094–12097. 10.1073/pnas.93.22.12094
- Gunawardena AH. 2008. Programmed cell death and tissue remodelling in plants. J Exp Bot. 59(3):445–451.
- Hanemann T, Szabó DV. 2010. Polymer-nanoparticle composites: from synthesis to modern applications. Materials. 3(6):3468–3517. 10.3390/ma3063468
- Kang TH, Kim DY, Seo YW. 2013. Identification and expression analysis of wheat vacuolar processing enzymes (VPEs). Plant Breed Biotech. 1(2):148–161. 10.9787/PBB.
- Kim YJ, Choi HS, Song MK, Youk DY, Kim JH, Ryu JC. 2009. Genotoxicity of aluminium oxide (Al2O3) nanoparticle in mammalian cell lines. Mol Cell Toxicol. 5(2):172–178.
- Kumagai F, Yoneda A, Tomida T, Sano T, Nagata T, Hasezawa S. 2001. Fate of nascent microtubules organized at the M/G1 interface, as visualized by synchronized tobacco BY-2 cells stably expressing GFP-tubulin:Time-sequence observations of the reorganization of cortical microtubules in living plant cells. Plant Cell Physiol. 42(7):723–732. 10.1093/pcp/pce091
- Lockshin RA, Zakeri Z, Tilly J. 1998. When cells die. NY, USA: John Wiley and Sons.
- Lombardi L, Ceccarelli N, Picciarelli P, Lorenzi R. 2007. Caspase-like proteases involvement in programmed cell death of Phaseolus coccineus suspensor. Plant Sci. 172(3):573–578. 10.1016/j.plantsci.2006.11.002
- Lord C, Gunawardena A. 2011. Environmentally induced programmed cell death in leaf protoplasts of Aponogeton madagascariensis. Planta. 233(2):407–421. 10.1007/s00425-010-1304-9
- Mao Z, Xu B, Ji X, Zhou K, Zhang X, Chen M, Han X, Tang Q, Wang X, Xia Y. 2015. Titanium dioxide nanoparticles alter cellular morphology via disturbing the microtubule dynamics. Nanoscale. 7(18):8466–8475. 10.1039/C5NR01448D
- McIlwain DR, Berger T, Mak TW. 2013. Caspase functions in cell death and disease. Cold Spring Harb Pers Biol. 5(4):a008656.
- Miralles P, Church TL, Harris AT. 2012. Toxicity, uptake, and translocation of engineered nanomaterials in vascular plants. Environ Sci Technol. 46(17):9224–9239. 10.1021/es202995d
- Nawkar GM, Maibam P, Park JH, Sahi VP, Lee SY, Kang CH. 2013. UV-induced cell death in plants. Int J Mol Sci. 14(1):1608–1628. 10.3390/ijms14011608
- Nel A, Xia T, Li N. 2006. Toxic potential of materials at the nano levels. Science. 311(5761):622–627. 10.1126/science.1114397
- Nilan RA, Vig BK. 1976. Plant test systems for detection of chemical mutagens. In: Hollaender A, editor. Chemical mutagens; principles an methods for their detection. New York, NY: Plenum Press. p. 143–170.
- Pandey P, Srivastava RK, Dubey RS. 2013. Salycilic acid alleviates aluminum toxicity in rice seedlings better than magnesium and calcium by reducing aluminum uptake, suppressing oxidative damage and increasing antioxidative defense. Ecotoxicology. 22(4):656–670. 10.1007/s10646-013-1058-9
- Papini A, Tani G, Di Falco P, Brighigna L. 2010. The ultrastructure of the development of Tillandsia (Bromeliaceae) trichome. Flora. 205(2):94–100. 10.1016/j.flora.2009.02.001
- Papini A, Mosti S, Milocani E, Tani G, Di Falco P, Brighigna L. 2011. Megasporogenesis and programmed cell death in Tillandsia (Bromeliaceae). Protoplasma. 248(4):651–662. 10.1007/s00709-010-0221-x
- Pesnya DS. 2013. Cytogenetic effects of chitosan-capped silver nanoparticles in the Allium cepa test. Caryologia. 66(3):275–281. 10.1080/00087114.2013.852342
- Piszczek E, Gutman W. 2007. Caspase-like Proteases and their role in programmed cell death in plants. Acta Physiol Plant. 29(5):391–398. 10.1007/s11738-007-0086-6
- Poborilova Z, Opatrilova R, Babula P. 2013. Toxicity of aluminium oxide nanoparticles demonstrated using a BY-2 plant cell suspension culture model. Environ Exp Bot. 91:1–11. 10.1016/j.envexpbot.2013.03.002
- Remédios C, Rosário F, Bastos V. 2012. Environmental nano-particles interactions with plants: morphological, physiological, and genotoxic aspects. J Botany. Artcile ID: 751686.
- Riedl SJ, Shi Y. 2004. Molecular mechanisms of caspase regulation during apoptosis. Nat Rev Mol Cell Biol. 5(11):897–907. 10.1038/nrm1496
- Roco MC. 2003. Broader societal issue of nanotechnology. J Nanoparticle Res. 5(3–4):181–189. 10.1023/A:1025548512438
- Rogers HJ. 2005. Cell death and organ development in plants. Curr Top Dev Biol. 71:225–261. 10.1016/S0070-2153(05)71007-3
- Ruffini Castiglione M, Giorgetti L, Geri C, Cremonini R. 2011. The effects of nano-TiO2 on seed germination, development and mitosis of root tip cells of Vicia narbonensis L. and Zea mays L. J Nanopart Res. 13(6):2443–2449. 10.1007/s11051-010-0135-8
- Ruffini Castiglione M, Cremonini GR, Bottega S, Spanò C. 2014. Impact of TiO2 nanoparticles on Vicia narbonensis L.: potential toxicity effects. Protoplasma 251(6): 1471–1479. 10.1007/s00709-014-0649-5
- Samraj AK, Keil E, Ueffing N, Schulze-Osthoff K, Schmitz I. 2006. Loss of caspase-9 provides genetic evidence for the type I/II concept of CD95 mediated apoptosis. J Biol Chem. 281(40):29652–29659. 10.1074/jbc.M603487200
- Schweizer D. 1976. Reverse fluorescent chromosome banding with chromomycin and DAPI. Chromosoma. 58(4):307–324. 10.1007/BF00292840
- Shiozaki EN, Chai J, Shi Y. 2002. Oligomerization and activation of Caspase-9 induced by Apaf-1 CARD. PNAS. 99(7):4197–4202. 10.1073/pnas.072544399
- Sobieh SS, Kheiralla ZMH, Rushdy AA, Yakob NAN. 2016. In vitro and in vivo genotoxicity and molecular response of silver nanoparticles on different biological model systems. Caryologia. 69(2):147–161. 10.1080/00087114.2016.1139416
- Vardar F, Ünal M. 2008. Proteolytic enzymes in plant programmed cell death. Turk J Sci Rev. 1(1):65–78.
- Vardar F, Ünal M. 2011. Immunolocalization of lipoxygenase in the anther wall cells of Lathyrus undulatus Boiss. during programmed cell death. Not Bot Hort Agrobo. 39(1):71–78.
- Vardar F, Ünal M. 2012. Ultrastructural aspects and programmed cell death in the tapetal cells of Lathyrus undulatus Boiss. Acta Biol Hung. 63(1):52–66. 10.1556/ABiol.63.2012.1.5
- Vardar F, Çabuk E, Aytürk Ö, Aydın Y. 2016. Determination of aluminum induced programmed cell death characterized by DNA fragmentation in Gramineae species. Caryologia. 69(2):111–115. 10.1080/00087114.2015.1109954
- Wang H, Li J, Bostock RM, Gilchrist DG. 1996. Apoptosis: a functional paradigm for programmed plant cell death induced by a host-selective phytotoxin and invoked during development. The Plant Cell. 8(3):375–391. 10.1105/tpc.8.3.375
- Wang J, Li X, Liu Y, Zhao X, Chen C, Tian F. 2011. MEK/ERK inhibitor U0126 enhanced salt stress-induced programmed cell death in Thellungiella halophila suspension-cultured cells. Plant Growth Regul. 63(3):207–216. 10.1007/s10725-010-9517-2
- Wang X, Wang X, Feng H, Tang C, Bai P, Wei G, Huang L, Kang Z. 2012. TaMCA4, a novel wheat metacaspase gene functions in programmed cell death induced by the fungal pathogen Puccinia striiformis f. sp. Tritici. Mol Plant Microb Interact. 25(6):755–764. 10.1094/MPMI-11-11-0283-R
- Woltering EJ, Bent A, Hoeberichts FA. 2002. Do plant caspases exist? Plant Physiol. 130(4):1764–1769. 10.1104/pp.006338
- Yanık F, Vardar F. 2015. Toxic effects of aluminum oxide (Al2O3) nanoparticles on root growth and development in Triticum aestivum. Water Air Soil Pollut. 226:296–308. 10.1007/s11270-015-2566-4
- Yen CH, Yang CH. 1998. Evidence for programmed cell death during leaf senescence in plants. Plant Cell Physiol. 39(9):922–927. 10.1093/oxfordjournals.pcp.a029455
- Yumurtacı A, Vardar F, Ünal M. 2007. Inhibition of barley root growth by Actinomycin D: effects on mitotic activity, protein content and peroxidase activity. Fresen Environ Bull. 16(8):917–921.