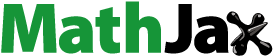
ABSTRACT
Oxygen measurement and control system is critical for minimizing corrosion in nuclear systems. Oxygen measurement and control tools use lead-bismuth eutectic (LBE) and pure lead as a coolant or as a spallation target. Oxygen can be supplied by either gas phase (H2O or O2) or solid phase (PbO dissolution); thus, oxygen control includes both gas phase and solid phase methods. This article focuses on oxygen concentration measurement and control of lead-bismuth eutectic in a small, static experimental facility. This facility was developed for oxygen sensor calibration and gas/solid phase control systems test programs. The oxygen sensor with Nano Cu/Cu2O closely the Nernstian behavior down to 195°C; the oxygen sensor measurement accuracy satisfied the requirements of subsequent experiments. The gas phase control system (verified according to different type of mass transfer, such as air, H2O, gas injection, and coverage) and the solid phase control system were very successful in small experimental devices. Accurate oxygen concentration control was achieved with both the gas and solid phase control systems.
1. Introduction
LBE and pure lead are usually used as the coolant in LFR and ADS. Pure lead/LBE has good neutron characteristics, safety performance, natural circulation abilities, and so on. However, the pure lead/LBE coolant also has drawbacks that include corrosion, generation of polonium (a highly poisonous substance), and structural instability [Citation1,Citation2]. The corrosion of LBE and pure lead is a physical or a physicochemical process involving species dissolution, oxidation and transport, chemical reactions, and new phase formation. To protect the structures from corrosion, oxygen should infiltrate the coolant, but the oxygen concentration should not be too high since high oxygen concentration can generate lead oxide that can block the channel. Thus, it is necessary to maintain the oxygen concentration within a range between the dissolution limit of the protective oxide film to avoid fast dissolution of the metal and the solubility limit of oxygen to avoid the formation of lead oxide [Citation3]. Therefore, we should control the oxygen concentration in order to ensure the safety of the reactor. Oxygen-control includes the gas phase method and the solid phase method [Citation4].
High-efficiency control of oxygen concentration in LBE requires a mass-transfer device that enriches oxygen, i.e. replacing oxygen consumed by steel oxidation. Furthermore, the device should remove oxygen – especially for conditioning the LBE at the beginning of operation, including the maintenance phase and incidental contamination. A rough classification of such devices according to the underlying type of mass transfer is given in [Citation5].
Table 1. Classification of devices for oxygen transfer to HLM according to the underlying type of mass transfer [Citation5].
There have been many studies on gas phase oxygen control [Citation5,Citation6]. Gas/liquid mass transfer is important for high-efficiency oxygen enrichment and removal. Oxygen can be supplied by direct gas injection, mixed with an inert carrier gas (argon or helium), or by controlling the partial pressure of a hydrogen/water mixture in the cover gas. Direct injection of oxygen and hydrogen gases mixed with an inert carrier gas (helium or argon) is a straightforward method for controlling the oxygen level in a liquid metal/alloy. The method has been applied to many liquid lead/LBE systems, including test loops. It is difficult to supply oxygen at the correct level by injecting oxygen directly because of the low oxygen target level needed in the system. However, such low concentration levels can be achieved with reaction systems, e.g. hydrogen and water.
The development of oxygen control systems is critical for nuclear systems using LBE and pure lead either as a coolant or as a spallation target. The principle of gas phase control is based on gas/liquid equilibrium between the cover gas and the LBE liquid when the oxygen concentration in the liquid is below the saturation concentration. According to the equilibrium relationship between gas and liquid, the oxygen concentration in the lead-bismuth eutectic can be calculated via the partial pressure of oxygen in the gas [Citation7,Citation8].
The main component of a solid phase oxygen control system is the mass exchanger with PbO particles [Citation9]. The mass exchanger consists of a volume filled with structurally stable and heat-resistant PbO pebbles. This system is normally installed in a by-pass loop at the coldest section of the non-isothermal LBE loop. When LBE flows through the PbO pebble bed, it initially extracts oxygen from the mass exchanger at a level equal to the oxygen solubility at the temperature of the mass exchanger. The oxygen concentration in the system can be controlled by regulating the temperature and the LBE flow rate in the mass exchanger. The development of PbO MXs was initiated at IPPE, Russia, in the 1970s. The serviceability of PbO MXs was demonstrated during this initial feasibility study. A number of problems were encountered during the operation of PbO MXs, however [Citation10]. One major problem is the so-called ‘poisoning of PbO,’ which involves the reaction of dissolved impurities (mainly Fe) in LBE with PbO, which forms a stable oxide (mainly Fe3O4) on the surface of the PbO. The solid PbO particles also have to be kept mechanically and thermally stable. Furthermore, poisoning of the PbO particles by dissolved impurities in LBE should be avoided during operation.
This paper focuses on oxygen concentration measurement and control of lead-bismuth eutectic in a small, static experimental facility. The oxygen sensor, gas phase control system, and solid phase oxygen system were tested and verified in a small lead-bismuth experimental device. The oxygen sensor was tested for measurement accuracy of oxygen saturation in LBE. The gas phase control system (verified according to different principles, air, H2O, gas injection, and coverage) was tested and verified for automatic control logic, control accuracy, oxidation, and reduction mechanism, and so on. The solid phase control device was tested and verified in a small experimental device for control accuracy, automatic control logic, and performance of lead oxide particles. These experimental results provide technical support for the subsequent development of practical tools.
2. Experimental
2.1. Experimental apparatus
The LBE small, static equipment is shown in : The equipment includes a steel crucible, Cu/Cu2O oxygen sensor, the solid phase mass exchanger, gas oxygen control system, integral lifting bellows, and heating mechanism. The amount of LBE in the experimental tank is 3 L, and the LBE temperature in the experimental tank is controllable with high precision; the temperature fluctuation is ±1°C, and the maximum operating temperature is 650°C. The device has performed oxygen sensor calibration experiments, oxidation and reduction mechanism research, gas oxygen control system experimental verification, solid phase mass exchanger experimental verification, and LBE static corrosion experiments.
2.2. Oxygen sensor
The oxygen concentration in the LBE of the experimental apparatus was measured by a solid-electrolyte type of oxygen sensor. The oxygen sensors were designed for use in liquid lead and lead alloys (). The most common reference electrodes for the application in liquid lead alloys are the air reference system and the metal/metal-oxide system. The sensors with the gas type reference electrode (RE) are more complicated than those with the solid and liquid type REs because of additional gas supply system in the structure. The solid type RE made of the mixture of solid metal powder and metal oxide powder containing a continuous gas phase between the solid powder was studied recently [Citation11] We use the Cu/Cu2O as the reference system for the measurement of the oxygen potential. The sensor cell is made of a solid-electrolyte conductor: zirconia (ZrO2) partially stabilized with yttria (8.5 mass% Y2O3). These form a tube that is closed at one end. The outer diameter of the tube is 8 mm, and the length is typically 350 mm; the wall thickness is 1 mm. The reference electrode inside the electrolyte tube consists of the mixture of Nano copper metal powder and Nano Cu2O powder for increase the contact area with YSZ and reduce the contact resistance. The housing of the sensors is made of Type 316L stainless steel and consists of the sensor head and a sheath that is screwed together with the sensor head. The oxygen sensor Cu/Cu2O reference electrode has been tested, and the signal is stable with very good measurement accuracy.
2.3. Oxygen control systems
External views of the gas phase control systems are shown in . The Ar, Ar/5%H2, and air were mixed in an oxygen control system by 3 flow controllers to obtain the required oxygen and hydrogen concentration in the cover gas and injected gas. When selecting Ar, Ar/5%H2, or H2O to oxygen control in the cover gas or injected gas, the gas passed through a moisturizer – the water vapor pressure was controlled by a precision thermostat. The tape of gas flow controllers is using SEVENSTAR CS200A, The maximum range is 500sccm. The Ar, Ar/5%H2, or air were oxygen-controlled as cover gas or injected gas. The gas was then directed to the experimental tank. Simultaneously, there were 2 ways for gas to enter the experimental tank: cover the surface of the liquid LBE or be injected directly into the liquid LBE. The gas injection device is a quarter-inch pipe with a lot of pores around 1 mm at the end pipe.
The solid phase oxygen control device includes lead oxide particles, mass exchanger, and control system is shown in . The lead oxide particles have a diameter of 9 mm and a density of 9.0 g/cm3. The number of lead oxide particles loaded in the mass exchanger is 20. The concentration of oxygen in the LBE is controlled via the gas pressure in the mass exchanger and the experimental tank.
2.4. Experimental procedure
Since the oxygen sensor uses YSZ as the electrolyte material, the lead-bismuth eutectic melting and cooling process prevents the influence on the oxygen sensor YSZ, and the oxygen sensor is fixed on the bellows to facilitate the adjustment of the liquid level of the oxygen sensor in the LBE When the LBE melting and solidification process, first, we performed a detailed oxygen sensor calibration experiment under saturated oxygen concentration conditions to ensure the accuracy of the oxygen sensor measurement. The verification results show that the oxygen sensor measurement accuracy meets the requirements. Secondly, we carry out gas phase control system (verified according to different principles, Air, H2O, gas injection, coverage) is tested and verified in automatic control logic, control accuracy, oxidation and reduction mechanism, etc. Furthermore, the solid phase control device is tested and verified in a small experimental device for control accuracy, automatic control logic, and performance of lead oxide particles. After the solid phase oxygen control experiment, we took out the lead oxide particles in the mass exchanger and analyzed it to observe the integrity and poisoning of the lead oxide particles.
3. Results and discussion
3.1. Calibration of oxygen sensor
The EMF was measured every 10 s using an Agilent 34970A with high input resistance (>10 GΩ). The EMF (E) was calculated according to the Nernst Equation [Citation12–Citation21]:
Inserting the values for the constants: n = 4, R = 8.31451 J/(mol K), and F = 96,485.31 C/mol with T in K yields the electromotive force E (volts), where is the oxygen partial pressure, and
is the oxygen partial pressures in LBE.
The Gibbs energy data used is
The oxygen partial pressure in Cu2O can be obtained by EquationEquation (2)(2)
(2) .
The oxygen partial pressure in PbO can be given by
So, the relationship between oxygen concentration and voltage and temperature is shown in EquationEquation (5)(5)
(5)
The oxygen sensor calibration experiments were usually performed in an oxygen-saturated environment. The pure lead-bismuth eutectic was present in the form of PbO in an oxygen-saturated environment. PbO activity can be expressed as:
For Cu/Cu2O vs. Pb/PbO, the relationship between voltage and temperature can be expressed as:
Since the oxygen sensor uses YSZ as the electrolyte material, the lead-bismuth eutectic melting and cooling process minimizes the influence on the oxygen sensor YSZ. The oxygen sensor is fixed on the bellows to facilitate the adjustment of the liquid level of the oxygen sensor in the LBE during the LBE melting and solidification process. The Cu/Cu2O oxygen sensor was calibrated in the saturated oxygen concentration lead-bismuth eutectic. The calibration results are shown in . The measurement temperature range was from 200°C to 500°C in steps of 25°C. The temperature was held constant for 50 minutes for each step during the transitions, and the temperature was decreased at a rate of 1°C/min to keep the LBE/PbO as close to equilibrium as possible during the transitions.
Figure 5. Calibration of the Nano Cu/Cu2O sensor with the YPSZ electrolyte in oxygen-saturated liquid lead showing the temperature (blue line), the calculated oxygen concentration by measured voltage (black line), and the calculated oxygen concentration by Delta G (red line).
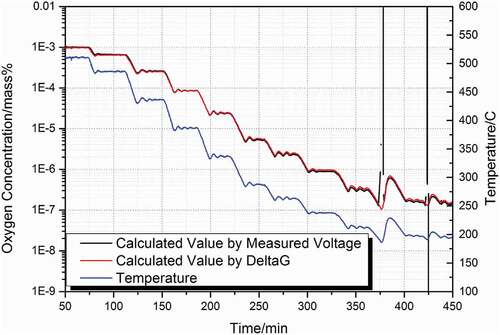
The EMF and temperature were measured every 10 seconds using an Agilent 34970A and selecting a high input resistance (> 10 GΩ). The stability of the oxygen sensors was carried out in stagnant, oxygen-saturated LBE at each temperature for 50 minutes. The EMF signal fluctuations were only ±0.1 mV over 50 minutes of temperature fluctuation (±1°C). These settings were chosen to reduce the noise uptake from the environment in which the test was performed. We then analyzed the experimental points collected during the calibration with time changes (). Between 195°C and 500°C, the EMF signal was stable, and the fluctuations were small. The oxygen sensor with Cu/Cu2O closely the Nernstian behavior down to 195°C, because of the use of nanoscale powders.
3.2. Gas phase control
The results of the gas oxygen control experiment are shown in . The oxygen concentration is controlled by injecting Ar, air, or Ar+5%H2 at different atmospheric ratios (a). We also included cover gas of Ar, air, and Ar+5%H2 at different ratios (b) as well as cover gas of Ar, H2O, Ar+5%H2 at different atmospheric ratios (c). The lead-bismuth eutectic temperature was 500°C, and the oxygen concentration was controlled by Ar, air, or H2O, Ar+5%H2 at different atmospheric ratios. When the oxygen concentration was higher than the set target oxygen concentration, the lead-bismuth eutectic reduction was performed by Ar+5%H2; when the oxygen concentration was lower than the set target oxygen concentration value, the ratio of Air or H2O was adjusted to supplement the oxygen. This oxidized or reduced the liquid lead alloys according to the following reactions [Citation1]:
Figure 6. The lead-bismuth eutectic temperature was 500°C, and the oxygen concentration was controlled by injecting Ar, air, and Ar+5%H2 at different atmospheric ratios (a). The cover gas of Ar, air, and Ar+5%H2 at different atmosphere ratios (b). The cover gas of Ar, H2O, and Ar+5%H2 at different atmospheric ratios (c).
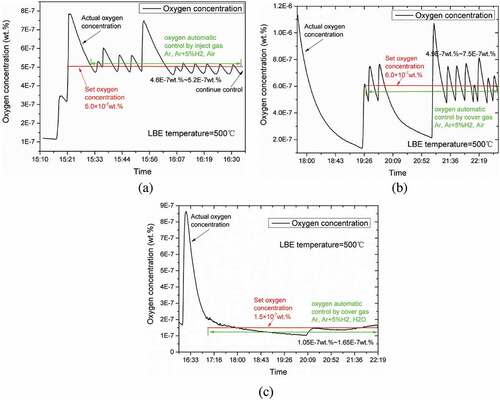
The target oxygen concentration was set to 1.5 × 10−7–6.0 × 10−7 wt.%, and the oxygen concentration precision control was realized using automatic control software. Different oxygen concentrations were controlled with oxygen supplementation by air; H2O, Ar, air, or Ar+5%H2 were controlled by PID logic, oxygen concentration is adjusted by LBE temperature, gas flow rate and gas oxygen partial pressure. The Ar, H2O, and Ar+5%H2 were controlled by the oxygen partial pressure in the atmosphere. Stable operation of the automatic control system continued for 1 hour; the automatic control experiment was ongoing.
The control accuracy of the experimental results are compared in panels (a) and (b) of . It is easier to perform precise oxygen concentration control via gas injection than cover, and the oxygen concentration control is more accurate. This is because the oxidation and reduction by gas injection is more efficient, and the reaction is more complete. From the experimental results in (b) and (c) of , oxygen concentration control (b) and (c) include both cover gases. The difference is that (b) has a ternary gas of Ar, air, Ar+5%H2, and in (c) the ternary gas is Ar, H2O, and Ar+5%H2. The results clearly show that precise oxygen concentration control can be more easily tested with Ar, H2O, and Ar+5% H2 in a small experimental facility – there is no need to quickly adjust the composition ratio of the ternary gas. The main reason is that H2O can achieve a lower gas oxygen partial pressure than air to prevent excessive oxygenation. The biggest problem with air oxygenation is that the oxidation rate is too fast: It is easy to supplement excessive oxygen, and it is impossible to control. In general, the gas injection method is more efficient in oxidation reduction. The gas utilization rate is higher, and the oxygen concentration is easier to accurately control. In a small experimental facility, Ar, H2O, and Ar+5%H2 can achieve a lower partial pressure of gas oxygen for more precise control.
3.3. Solid phase control
The main component of a solid phase oxygen control system is the mass exchanger. The mass exchanger consists of a volume filled with structurally stable and heat-resistant PbO pebbles. The solid phase oxygen control device uses lead oxide particles to dissolve and supplement oxygen for LBE by regulating the temperature and the LBE flow rate at the mass exchanger. The reduction adopts Ar+5%H2. The results of the solid phase oxygen control experiments are shown in . The lead-bismuth eutectic temperature was 400°C. When the oxygen concentration was reduced by Ar+5%H2 – and the oxygen concentration was reduced to the target value of 9.7 × 10−7 wt.% – the oxygen was controlled by the automatic control of the LBE via the solid oxygen control mass exchanger.
Figure 7. The solid phase oxygen control device used PbO particles to dissolve and supplement oxygen, and the reduction adopted Ar+5%H2. The lead-bismuth eutectic temperature was 400°C. The oxygen was controlled by the automatic control of the LBE via the solid oxygen control mass exchanger.
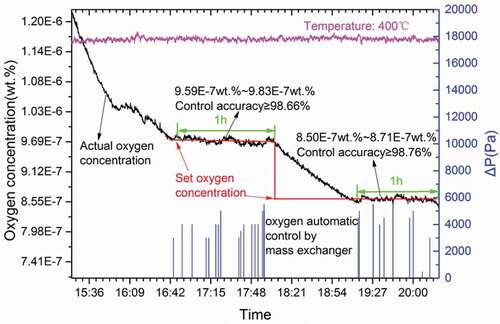
The LBE was circulated through the lead oxide dissolution zone in the mass exchanger by controlling the difference in gas pressure between the mass exchanger and the experimental tank. The final oxygen concentration was controlled at 9.59 × 10−7–9.83 × 10−7 wt.%. The Ar+5%H2 was added to reduce the oxygen concentration. When the oxygen concentration was reduced to 8.6 × 10−7 wt.%, the oxygen was controlled by the automatic control of the LBE through the solid oxygen control, oxygen concentration controlled by PID logic, which is adjusted by gas pressure and LBE temperature. The blue curve in shows the control system operation process. When the oxygen concentration was lowered, the gas pressure in the mass exchanger was increased, and the LBE flowed through the lead oxide particles. The LBE then had an excessive oxygen concentration. Because the delta gas pressure between mass exchange and tank is changes slowly, the LBE flows slowly and the flow condition is negligibly small. Flow out of the mass exchanger increased the oxygen content of the lead in the experimental tank. The final oxygen concentration was controlled to 8.5 × 10−7–8.71 × 10−7 wt.% with stable operation of the automatic control system over 1 hour; the current automatic control experiment was ongoing.
PbO particles after testing are shown in . The surface topography of the PbO particles before and after the test are obvious; no particle breakage occurred even after the PbO particles were dissolved and washed after 1000 h in LBE. This indicates that the mechanical properties of PbO particles meet the requirements. After the experiment, the surfaces of the lead oxide particles exhibited obvious cavities after dissolution.
The PbO might react with dissolved impurities in LBE to form oxides on the particles’ surface. This could slow the dissolution process. These spheres might be contaminated by impurities dissolved in LBE. This was expected due to the high oxygen concentration during the experiment and the short experimental time. A high oxygen concentration allowed compound formation in the bulk with subsequent consumption of iron or nickel dissolved in LBE. This reduced the probability of surface contamination. There was no evidence of Fe3O4 formation by impurities on the particles’ surface. To prove this hypothesis, SEM (scanning electron microscopy) and EDS (energy dispersive spectrometry) analysis were performed to identify contamination (). The results demonstrated no evidence of any oxide layers (Fe3O4) on the particle surfaces. In addition, there was no evidence of poisoning of the PbO particles by particle impurities. This verifies that the mass exchanger design can effectively avoid PbO particle poisoning.
4. Conclusions
This article focused on oxygen concentration measurement and control of lead-bismuth eutectic in a small, static experimental facility. The novel oxygen sensor, gas phase control system, and solid phase oxygen system were tested and verified in a small lead-bismuth experimental device.
The Nano Cu/Cu2O provided an electric potential output generally in agreement with the theoretical lines between 195°C and 500°C. Stability testing of the oxygen sensors was performed in stagnant oxygen-saturated LBE at each temperature for 50 minutes. The EMF signal fluctuations were only ±0.1 mV when the temperature fluctuation was ±1°C. The oxygen sensor with Nano Cu/Cu2O closely the Nernstian behavior down to 195°C.
The gas phase control system (verified according to different principles, air, H2O, gas injection, and coverage) was very successful for small experimental devices. Accurate oxygen concentration control was achieved via the gas phase control system.
The solid phase control device was tested and verified in a small experimental device for control accuracy, automatic control logic, and performance of lead oxide particles. The solid phase control system offers accurate oxygen concentration control. Simultaneously, SEM and EDS revealed no evidence of surface contamination in the PbO particles. This verified that the mass exchanger design can effectively avoid poisoning in PbO particles.
Disclosure statement
No potential conflict of interest was reported by the authors.
References
- Fazio C. Handbook on lead-bismuth eutectic alloy and lead properties, materials compatibility, thermal-hydraulics and technologies. In: J-L. Courouau, editor. Chemistry control and monitoring systems. Vol. 4. Paris: OECD; 2015. p. 185–187.
- Gromov BF. The problems of technology of the heavy liquid metal coolants (Lead-bismuth eutectic, lead). Proceedings of the Conference of the Heavy Liquid Metal Coolants in Nuclear Technology; Obninsk, Russian Federation; 1998.
- Zrodnikov AV. Heavy liquid metal coolant lead-bismuth eutectic and lead technology. At Energy. 2004;97(2):534–537.
- Nam HO, Lim J, Han DY, et al. Dissolved oxygen control and monitoring implementation in the liquid lead-bismuth eutectic loop: HELIOS. J Nucl Mater. 2008;376:381–385.
- Schroer C, Wedemeyer O, Konys J. Gas/liquid oxygen-transfer to flowing lead alloys. Nucl Eng Des. 2011;241:1310–1318.
- Courouau JL. Impurities and oxygen control in lead alloys. J Nucl Mater. 2002;301:53–59.
- Ganesan RT, Gnanasekaran T, Srinivasa RS. Diffusivity, activity and solubility of oxygen in liquid lead and lead-bismuth eutectic alloy by electrochemical methods. J Nucl Mater. 2006;349:133–149.
- Lefhalm CH, Knebel JU, Mack KJ. Kinetics of gas phase oxygen control system (OCS) for stagnant and flowing Pb-Bi systems. J Nucl Mater. 2001;296:301–304.
- Kondo M, Takahashi M, Miura K, et al. Study on control of oxygen concentration in lead-bismuth flow using lead oxide particles. J Nucl Mater. 2006;357:97–104.
- Lim J. Active oxygen control by a PbO mass exchanger in the liquid lead–bismuth eutectic loop: MEXICO. J Nucl Sci Technol. 2016;54:131–137.
- Pribadi MA, Kondo M, Minoru T. Performance of solid electrolyte oxygen sensor with solid and liquid reference electrode for liquid metal. Sens Actuators B Chem. 2017;241:1261–1269.
- Marino A, Lim J, Keijers S. A temperature dependence of dissolution rate of a lead oxide mass exchanger in lead-bismuth eutectic. J Nucl Mater. 2014;450:270–277.
- Lim J, Mariën A, Rosseel K. Accuracy of potentiometric oxygen sensors with Bi/Bi2O3 reference electrode for use in liquid LBE. J Nucl Mater. 2012;429:270–275.
- Courouau JL. Electrochemical oxygen sensors for on-line monitoring in lead-bismuth alloys: status of development. J Nucl Mater. 2004;335:254–259.
- Konys J. Development of oxygen meters for the use in lead-bismuth eutectic. J Nucl Mater. 2001;296:289–294.
- Sung HL, Song TY. Kinetics of gas phase oxygen control system and oxygen concentration measurement in liquid Pb and LBE. J Ind Eng Chem. 2007;13:602–607.
- Müller G, Heinzel A, Schumacher G, et al. Control of oxygen concentration in liquid lead and lead–bismuth. J Nucl Mater. 2003;321:256–262.
- Konys J, Muscher H, Voß Z, et al. Oxygen measurements in stagnant lead-bismuth eutectic using electrochemical sensors. J Nucl Mater. 2004;335:249–253.
- Brissonneau L. Oxygen control systems and impurity purification in LBE: learning from DEMETRA project. J Nucl Mater. 2011;415:348–360.
- Schroer C, Konys J, Verdaguer A, et al. Design and testing of electrochemical oxygen sensors for service in liquid lead alloys. J Nucl Mater. 2011;415:338–347.
- Mariën A, Lim J, Rosseel K, et al. Solid electrolytes for use in lead-bismuth eutectic cooled nuclear reactors. J Nucl Mater. 2012;427:39–45.