Abstract
Low phosphorus (LP) limits crop growth and productivity in the majority of arable lands worldwide. Here, we investigated the changes in physiological and biochemical traits of Tibetan wild barleys (Hordeum vulgare L. ssp. spontaneum) XZ99 (LP tolerant), XZ100 (LP sensitive), and cultivated barley ZD9 (moderately LP tolerant) under two phosphorus (P) levels during vegetative stage. These genotypes showed considerable differences in the change of biomass accumulation, root/shoot dry weight ratio, root morphology, organic acid secretion, carbohydrate metabolism, ATPase (Adenosine triphosphatase) activity, P concentration and accumulation under LP in comparison with CK (control) condition. The higher LP tolerance of XZ99 is associated with more developed roots, enhanced sucrose biosynthesis and hydrolysis of carbohydrate metabolism pathway, higher APase (Acid phosphatase) and ATPase activity, and more secretion of citrate and succinate in roots when plants are exposed to LP stress. The results prove the potential of Tibetan wild barley in developing barley cultivars with high tolerance to LP stress and understanding the mechanisms of LP tolerance in plants.
INTRODUCTION
Phosphorus (P) is an essential macronutrient element, significantly affecting plant growth and metabolism. The low phosphorus (LP) availability to plants results not only from naturally P-deficient soil, but also from its rapid association with cations [particularly with aluminum (Al) and iron (Fe)] and organic compounds. Thus, P is one of the major plant nutrition problems limiting crop growth and development in both acidic and calcareous soils (Raghothama Citation1999). Although the amount of total P is abundant in many soils, the available amount of P is commonly low for plant uptake (Vance et al. Citation2003) and plants frequently encounter a scarcity of phosphate (Pi) in agricultural soils (Raghothama Citation1999). Amelioration of P deficiency by application of large quantities of fertilizers is not economically sustainable and also leads to environmental pollution. It is therefore important to identify genetic resources that have high tolerances and to understand the mechanisms of LP tolerance in plants.
Plant genotypes differ widely in ultimate yield when grown at identical nutrient levels (Qingren et al. Citation1999). In view of P, these differences are the outcome of an integrated effect of many root- and shoot-associated mechanisms of P-efficient and P-inefficient genotypes (Qingren et al. Citation1999). However, it is difficult to define specific plant mechanisms responsible for differential yield between P-efficient and P-inefficient genotypes (Ozturk et al. Citation2005), because these mechanisms induced by deficiency vary greatly with the kind of nutrient and plant, and many of them are still poorly understood (Qingren et al. Citation1999). One or several of these mechanisms often explain the higher tolerance of P-efficient genotypes to P deficiency in comparison with P-inefficient genotypes, and have been discussed by many research groups (Vance et al. Citation2003; Lambers et al. Citation2006; Lynch and Brown Citation2006; Lynch et al. Citation2008).
Plant species and genotypes of a given species have developed diverse adaptive mechanisms in response to persistently low levels of available P in the rhizosphere. To maintain relatively normal growth under P-deficient conditions, plants develop several morphological, physiological and biochemical adaptations, such as root system modification (Lynch Citation2007), increased organic acid exudation (Ryan et al. Citation2001) and production of acid phosphatases (Bozzo et al. Citation2002), and induced carbohydrate metabolism-related enzyme activities (Ciereszko and Barbachowska Citation2000).
Modification in the root system is a well-documented response to P starvation (Hammond and White 2007). A hallmark of plant response to P deficiency is greater stimulation of root growth at the expense of shoot growth (Hermans et al. Citation2006). Consequently, a particular plant species explores more soil and acquires more nutrients by enhancing the total root surface area throughout the soil profile (Gahoonia and Nielsen Citation2004). Under LP conditions, a biochemical adaptation can occur for the P-efficient plants, i.e. more production of root exudates (Ryan et al. Citation2001) and acid phosphatase (APases) which mobilizes various inorganic and organic P forms in the rhizosphere, and thus P uptake is enhanced (Bozzo et al. Citation2002). Transgenic tobacco (Nicotiana tabacum L.) plants overproducing citrate enhanced P uptake and increased P utilizing efficiency (López-Bucio et al. Citation2000). In order to maximize external Pi acquisition and internal Pi use, plants produce and exude APases under LP stress (Bozzo et al. Citation2002).
In addition, changes in the synthesis, translocation and degradation of carbohydrate (sucrose), sucrose synthases (SuSy), sucrose-phosphate synthases (SPS) and acid invartase (AI) have been shown to be differentially expressed during Pi starvation (Ciereszko and Barbachowska Citation2000). Plants require very active loading, unloading and utilization of sucrose in the source (leaf) and sink (root) tissue under LP, for normal growth and development. The SPS enzyme is involved in sucrose synthesis in the cytosol from triose-phosphate (Quick Citation1996). Furthermore, SuSy was found to be localized in the companion cells of the phloem in both loading and unloading zones of maize (Zea mays L.) and citrus (Citrus paradisi Macf.) (Nolte and Koch Citation1993). Likewise, AI, which is present in the cell wall, apoplast and vacuole, causes hydrolysis of sucrose into hexoses (Halford et al. Citation2011). The enzyme H+-ATPase uses ATP (Adenosine triphosphate) to pump protons out of the cell, thereby generating pH and an electrochemical proton (H+) gradient potential difference across the plasmalemma (Palmgren and Harper Citation1999). Yan et al. (Citation2002) reported that cluster roots of white lupin (Lupinus albus L. cv Amiga) increased catalytic activity of a plasma membrane H+ ATPase in P deficient conditions that might be responsible for the increase in H+ extrusion.
Barley (Hordeum vulgare L.) is the fourth largest cereal crop in terms of planting areas in the world, after wheat (Triticum aestivum L.), maize and rice (Oryza sativa L.) (Able et al. Citation2007). It is particularly sensitive to acid soil as well as LP in comparison with other cereal crops (Tanaka et al. Citation1984). Consequently, it is quite imperative to identify highly tolerant/efficient genotypes for use in breeding programs to develop barley cultivars with high P efficiency. Tibetan annual wild barley (Hordeum vulgare L. ssp. spontaneum) is the ancestor of cultivated barley and contains valuable novel genes for barley improvement (Dai et al. Citation2012). In our previous work, we identified the two accessions from Tibetan wild barley, i.e. XZ99 and XZ100, which showed high tolerance and sensitivity to LP stress, respectively (Nadira et al. Citation2014). However, the basic physiological, biochemical and molecular mechanisms involved in LP tolerance are still unclear. Thus, further studies are required to test the hypothesis that Tibetan wild barley XZ99 has a different mechanism of low-P tolerance from cultivated barley (ZD9). The present study attempts to provide novel insight into the effects of LP on growth and metabolism of Tibetan wild and cultivated barleys. This research would be useful to understand the adaptation mechanisms of Tibetan wild barley genotype to LP stress and provide an approach for the genetic improvement of P utilization efficiency in cultivated barley.
MATERIALS AND METHODS
Plant materials and experimental design
A greenhouse hydroponic experiment was performed at Zijingang Campus, Zhejiang University, Hangzhou, China. According to a previous study (Nadira et al. Citation2014), two Tibetan wild barley (Hordeum vulgare L. ssp. spontaneum) genotypes, XZ99 (LP tolerant) and XZ100 (LP sensitive), and a cultivated barley (Hordeum vulgare L), ZD 9 (moderately LP tolerant), were used in the experiment. Healthy seeds were surface sterilized by soaking in 3% hydrogen peroxide (H2O2) for 30 min, thoroughly rinsed in tap water and then germinated in sterilized moist sand in an incubator at 20 ± 1°C. When seedlings grew to the second leaf (10 d old), uniform healthy plants were selected and transplanted into 5-L pots containing 4.5 L basic nutrient solution (BNS) for initial growth. The composition of the BNS was as described by Wu et al. (Citation2003). The pH of the solution was adjusted to 5.8 ± 0.1 with sodium hydroxide (NaOH) or hydrochloric acid (HCl) as required. Half-concentration solution was supplied to plants in the first week, and then changed to full concentration from the second week. The container was covered with a plate with six evenly spaced holes (two plants per hole). Two treatments were initiated on the 14th day after transplantation: (1) BNS containing 500 μM KH2PO4 (Potassium dihydrogen phosphate) (Control, CK); (2) Low phosphorus (LP), 10 µM KH2PO4. The modified nutrient solution was formulated as P-free by replacing all the phosphorus of BNS with nitrates or chlorides. The nutrient solution was continuously aerated with pumps and renewed once a week.
Plant sampling
The plants were sampled on the 20th day after exposure to LP. Plants were collected and rinsed with tap water three times to remove surface ions, and then blotted dry with tissue paper which was used in biochemical analysis. For root morphology study, we used five seedlings from different treatments within different genotypes. After measurement of plant height and root length, plants were separated into roots and shoots, and dried at 105°C for 3 h, followed by drying at 80°C for 72 h, and then weighed. Then the shoot and root samples were ground to pass through a 0.42-mm screen (40-mesh) for phosphorus concentration determination.
Phosphorus determination
For P determination, acid digestion of the samples was employed. Dry powdered tissues were digested through an acid mixture of HNO3 (Nitric acid) (60%) and HClO4 (Perchloric acid) (60%) (2.5:1) in an automatic distillation (FOSS, 4400 Kjeltic Auto Distillation) system, and the final volume was made to be 25 mL. P concentrations in shoots and roots were estimated by the vanadate-molybdate yellow color method (Chapman and Pratt Citation1961) using a spectrophotometer (SHIMADZU, UV-2410PC, Japan).
Calculation of various growth parameters and phosphorus uptake
The following variables were measured: dry weight of shoot and root, shoot and root P concentration and accumulation. Phosphorus accumulation was calculated from the following formula:
Root morphology
Root surface area, volume, diameter and number of root tips of barley plants were determined using root automatism scan apparatus (MIN MAC, STD1600+), equipped with WinRHIZO software offered by Regent Instruments Co. One measurement consisted of five plants.
Determination of enzyme activities relevant to carbohydrate metabolism
Extraction and analysis of SuSy, SPS and AI were performed according to the methods detailed by Ruan et al. (Citation2003) with minor modification. For SuSy activity assay, the reaction mixtures (0.25 mL) contained: 50 mM Mops-NaOH (Mops-sodium hydroxide) (pH 7.5), 15 mM MgCl2 (Magnesium chloride) 15 mM KCI (Potassium chloride), 20 mM fructose, 12 mM UDP-glucose (Uridine diphosphate glucose), and 150 μL of desalted extract. Samples were incubated for 15 min at 37°C and the reactions terminated by the addition of 1 N NaOH, followed by boiling for 10 min. The reaction mixture (0.5 mL) for SPS assay activity contained: 50 mM Mops (pH 7.5), 15 mM MgCl2, 4 mM NaF (Sodium fluoride), 8 mM Glu6P (Glucose 6-phosphate), 12 mM UDPG, 8 mM Fru6P (Fructose 6-phosphate), 0.3 mM EDTA (Ethylenediaminetetra acetic Acid) and 150 μL of desalted extract. Samples were incubated for 20 min at 37°C, the reactions were terminated by addition of 1 N NaOH (0.1 mL), and unreacted fructose and Fru6P were destroyed by boiling for 10 min. After cooling at room temperature, absorbance was measured at 480 nm. Enzyme activity was calculated using sucrose as standard and expressed as µM l h−1 mg−1 protein.
For acid invertase activity, leaf samples were ground into a fine slurry with 100 mM Na/acetate buffer (pH 4.5) and centrifuged; the pellet was washed twice with extraction buffer. Reaction mixtures containing 100 mM Na/acetate buffer (pH 4.5) and 100 mM sucrose were incubated at 37°C for 30 min. Total soluble protein contents was measured with bovine serum albumin as standard Bradford (Citation1976).
Determination of intracellular acid phosphatases (APase) and ATPase activity
Intracellular acid phosphatase (APase) activity was determined in shoots and roots of all genotypes at 3 weeks after exposure to LP stress. Leaf and root samples (0.5 g) were separately ground in liquid nitrogen, extracted in 50 mM Na-acetate buffer, pH 5.0 with 1 mM DTT (Dithiothreitol) and centrifuged at 12,000 g for 10 min at 4°C (Ciereszko et al. Citation2002). Enzyme extract was incubated for 15 min with 6 mM p-nitrophenyl phosphate (ρNPP) (in 100 mM Na-acetate buffer, pH 5.0) at 37°C, then the reaction was stopped by adding 0.5 M NaOH and absorbance was read at 410 nm. The intracellular activity was expressed as µM ρNP min−1g−1 fresh weight (FW).
ATPase activity was determined by measuring the release of Pi using the activity assay kit (Jiancheng Bio Co., Nanjing, China; http://www.njjcbio.com/html/search.php).
Collection of exudates and measurement of organic acids
For the collection of root exudates, plants were grown in 1-L plastic pots containing complete nutrient solution. The low and normal P levels contained 10 μM and 500 mM KH2PO4, respectively. After 12 d of growth, roots of seedlings were rinsed in distilled water and deionized water three times, respectively, then blotted dry with tissue paper. The plants were grown in a growth chamber with a controlled temperature of 20 ± 1°C and a photoperiod of 12 h light and 12 h dark. After 24 h, 200 mL of the culture solution was collected, purified by anion-cation exchange resin, eluted by 15 mL 1 M HCl, and concentrated to a final volume of 1.2 mL with a rotary evaporator at 40°C. The concentrated solution was filtered through a 0.22-μm membrane and stored at 4°C. Standards of organic acids (Sigma, St. Louis, America) and concentrated solution were measured by high performance liquid chromatography (Waters 2695–2996, USA) with an Elite-C18 chromatographic column (5 μM; 46 mm × 200 mm) at 25°C. The mobile phase was 10 mM (NH4)2HPO4 (Diammonium hydrogen phosphate) at pH 2.5, the flow rate was 0.8 mL min−1 and the injection volume was 20 μL. The detection wavelength was set at 214 nm.
Statistical analysis
All of the data presented are mean values for each treatment. An analysis of variance was conducted between the treatments. Two-way analysis of variance (ANOVA) was carried out between Tibetan wild and cultivated barley plants under control and treatments and followed by the least significant difference (LSD) multiple range test (P < 0.05), using SAS 9.2 (SAS Institute Inc., Cary, NC, USA) statistical software. Origin Pro version 8.0 (Origin lab corporation, Wellesley Hills, Wellesley, MA, USA) was used to prepare the graphs.
RESULTS
Root and shoot length and dry weight
Under LP conditions, shoot height of all genotypes was significantly inhibited relative to control, with XZ99 (decreased by 8.88%) being less affected than XZ100 and ZD9 (decreased by 33.33% and 21.66%, respectively) (). However, root length of ZD9 was increased under LP; there were no significant difference for XZ99 and a significant reduction for XZ100, when compared with control. For shoot dry weight, XZ100 showed a significant reduction under LP, while no significant difference was found in XZ99 or ZD9 relative to control. By contrast, root dry weight of XZ99 and ZD9 was significantly increased under LP, although XZ100 showed a marked reduction. For example, plant dry weight under LP decreased by 16.70% in XZ100, but increased by 30.87% and 44.08% in XZ99 and ZD9, respectively, compared with their controls.
Figure 1 Variation in response of three barley genotypes XZ99, XZ100 and ZD9 for shoot height and shoot dry weight (left alignment) and root length and root dry weight (right alignment). Seedlings were grown for 20 d on complete nutrient solution with Control: CK (500 μM) and Low phosphorus: LP (10 μM). Data are mean ± standard error of four replicates. Different letters indicate significant differences (P < 0.05) among the genotypes within each P level. * indicates significant differences (P < 0.05) between the two P levels for the same genotype.
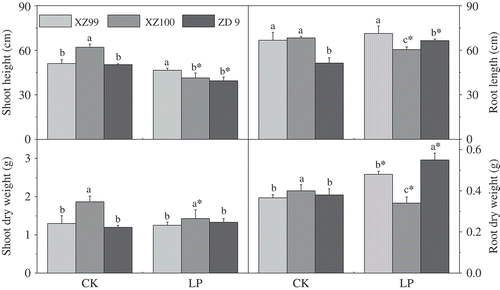
Phosphorus concentration and accumulation
There was a significant (P < 0.05) difference in shoot and root P concentration among the three genotypes under both control and LP (). Under LP, XZ100 had significantly lower and higher P concentration in shoots and roots than XZ99 and ZD9, respectively, indicating less P remobilization from roots to shoots. The difference in P remobilization among the three genotypes can also be confirmed in the data of P accumulation. Hence, there was no significant difference in shoot P accumulation under both LP and control, and XZ99 had more root P accumulation than XZ100 and ZD9 under control, while XZ100 had the highest root P accumulation under LP.
Table 1 Effects of P (Phosphorus) levels on P concentration and accumulation of three barley genotypes. Seedlings were grown for 20 d on complete nutrient solution with Control: CK (500 μM) and Low phosphorus: LP (10 μM)
Root morphology
The root morphological parameters of three barley genotypes under the two P treatments are shown in . In comparison with control, all genotypes showed larger root surface areas, number of root tips and root surface area/volume ratio under LP, although the increased extent differed among genotypes. By contrast, root diameter and volume were less affected by LP in comparison with control, although XZ100 had a significantly larger root diameter under LP than control. It should be noted that XZ99 and ZD9 showed a dramatic increase of root tips under LP, indicating LP stress induces the development of new roots in the barley genotypes tolerant to LP.
Table 2 Effects of P (Phosphorus) levels on the root morphology of three barley (Hordeum vulgare L. ssp. spontaneum) genotypes. Seedlings were grown for 20 d on complete nutrient solution with Control: CK (500 μM) and Low phosphorus: LP (10 μM)
Activities of acid invertase (AI), sucrose synthase (SuSy) and sucrose phosphate synthase (SPS)
The activities of AI, SuSy and SPS were determined in leaves and roots of the three genotypes (). Significant genotypic difference in AI was observed under LP stress. Under normal conditions (control), ZD9 and XZ100 had the highest leaf and root AI activities, respectively. Under LP, XZ99 showed significant increase of both leaf and root AI activities; however, no significant effect and significant increase were detected in leaves and roots, respectively, of ZD9, and XZ100 had slight (leaf) and significant (root) reduction, in comparison with their controls. Both SuSy and SPS activities were significantly lower in leaves and roots of XZ99 than those of XZ100 and ZD9 under control. Nevertheless, under LP, these activities were significantly higher in XZ99 than in other two genotypes. For example, under LP treatments, shoot/root SPS and SuSy activities increased by 76.93/17.79% and 116.32/27.84%, respectively, in XZ99 compared with the ZD9.
Figure 2 Variation in response of three barley (Hordeum vulgare L. ssp. spontaneum) genotypes (XZ99, XZ100 and ZD9) for AI (acid invertase) activity, SPS (sucrose phosphate synthase) activity and SuSy (sucrose synthase) activity (µM h−1 mg−1 protein). Seedlings were grown for 20 d on complete nutrient solution with Control: CK (500 μM) and Low phosphorus: LP (10 μM). Data are mean ± standard error of four replicates. Different letters indicate significant differences (P < 0.05) among the genotypes within each P level. * indicates significant differences (P < 0.05) between the two P levels for the same genotype.
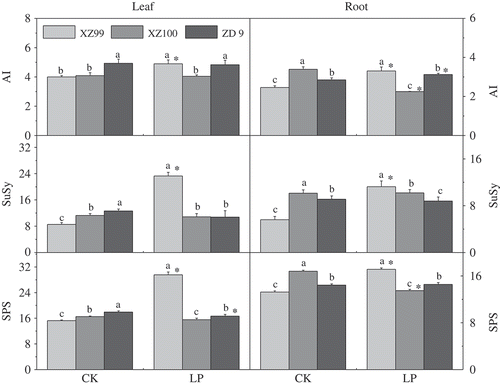
Activity of intracellular acid phosphatases (APase), plasma membrane H+, Ca2+Mg2+- and Na+K+-ATPase
The effects of P level on activities of intracellular acid phosphatases (APase), plasma membrane H+ (Hydrogen)-, Ca2+Mg2+ (Calcium-magnesium)- and Na+K+ (Sodium-potasium)-ATPase in the three barley genotypes are shown in . Leaf APase activity had less difference among genotypes under control conditions, but under LP, the difference among genotypes was significant, with XZ99 being highest and XZ100 lowest. In roots, the APase activity remained little changed under LP relative to control, although the difference among genotypes was significant. For the activities of Ca2+Mg2+-ATPase and Na+K+-ATPase, in both leaf and root of XZ99 they significantly decreased under CK and increased under LP compared to ZD9. Under CK, the Ca2+Mg2+-ATPase and Na+K+-ATPase in both leaf and root of ZD9 significantly increased compared to XZ99 and XZ100. Under LP, the leaf and root Ca2+Mg2+-ATPase significantly decreased and increased, respectively, in ZD9 compared to XZ100, and the Na+K+-ATPase in both leaf and root significantly decreased compared to XZ99. Compared to control, H+-ATPase activity in leaves significantly increased under LP for XZ99 and ZD9, whereas no significant difference was detected in XZ100. In roots, the H+-ATPase activity of all three genotypes significantly increased under LP relative to control. Among the three genotypes, the highest activities of Na+K+-, Ca++Mg++- and H+-ATPase were observed in XZ99 under LP.
Figure 3 Variation in response of three barley (Hordeum vulgare L. ssp. spontaneum) genotypes (XZ99, XZ100 and ZD9) for acid phosphatases (APase) (µM ρNP g−1 FW min−1) activity, H+-ATPase, Ca2+Mg2+-ATPase, Na+K+-ATPase activity (µM Pi mg−1 protein h−1) activity. Seedlings were grown for 12 d on complete nutrient solution with Control: CK (500 μM) and Low phosphorus: LP (10 μM). Data are mean ± standard error of four replicates. Different letters indicate significant differences (P < 0.05) among the genotypes within each P level. * indicates significant differences (P < 0.05) between the two P levels for the same genotype.
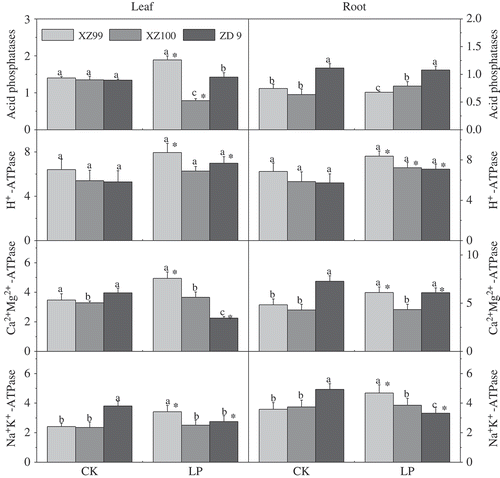
Organic acids (OAs)
Citrate, malate and succinate were measured in the root exudates of the plants at 12 d after P treatment (). Significant genotypic difference in organic acid (OA) contents was observed under LP stress. Under normal conditions, both citrate and succinate contents were significantly lower in XZ99 than in the other two genotypes. However, under LP, dramatic increase and reduction could be found in XZ99 and XZ100, respectively. For instance, citrate and succinate contents were increased by 172.21% and 199.27% in XZ99, but decreased by 65.37% and 62.10% in XZ100. However, ZD9 had relatively less change under LP, but a significant difference was observed in comparison with control. For malate, under normal conditions, XZ99 and ZD9 had the lowest and highest contents, and under LP, XZ99 and XZ100 had the highest and lowest contents, respectively, with the difference among genotypes being significant. On the whole, a greater increase in OA secretion was observed in XZ99 compared to ZD9 and XZ100 under LP relative to controls.
Figure 4 Organic acids (OAs) (μM h−1 g−1 DW root) exudation by the roots of three barley (Hordeum vulgare L. ssp. spontaneum) genotypes (XZ99, XZ100 and ZD9). Seedlings were grown for 12 d on complete nutrient solution with Control: CK (500 μM) and Low phosphorus: LP (10 μM). Data are mean ± standard error of four replicates. Different letters indicate significant differences (P < 0.05) among the genotypes within each P level. * indicates significant differences (P < 0.05) between the two P levels for the same genotype.
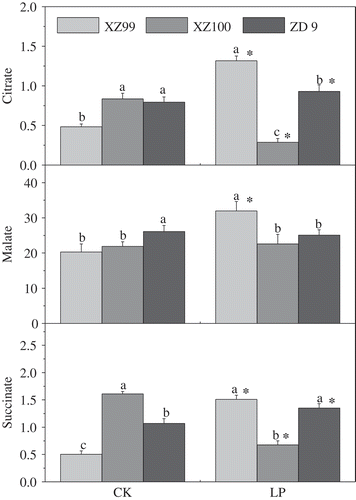
DISCUSSION
In a previous experiment, we compared the difference among 124 wild and cultivated barley genotypes in response to LP, and identified three genotypes differing greatly in LP tolerance (Nadira et al. Citation2014). This study showed that the three genotypes differed in morpho-physiological traits and biochemical adaptation mechanisms responsible for tolerance to LP stress. LP stress caused a significant reduction of plant height in comparison with the control (normal P supply). However, root growth and dry biomass were increased in response to LP stress in XZ99 (). Akhtar et al. (Citation2008) demonstrated a superior root growth under P starvation (LP) in Brassica, which helps to acquire more P. It was also observed that wheat genotypes with larger root dry weight at LP level had relatively lower reductions in shoot dry matter (Yaseen and Malhi Citation2011). In agreement with these results, we supposed that higher growth and biomass of XZ99 under LP could be attributed to its larger root system.
P concentrations and accumulation of shoots and roots were decreased when plants were exposed to LP conditions (). Under normal conditions, the shoot and root P concentrations of XZ99 were higher than those of XZ100 and ZD9. Moreover, the shoot/root P concentration ratio, which describes the partitioning of P between shoots and roots, was higher in XZ99 and ZD9 under LP. It was also reported that the efficient Brassica cultivar had a significant correlation between phosphate transport rate (PTR) and shoot P concentration and uptake (Akhtar et al. Citation2008). The cultivar with higher PTR was able to retain higher P in their shoots under P starvation. The current results show that XZ99 can accumulate more P under LP conditions than the other two genotypes. Several researchers have reported that relatively lower proportions of total P were retained in roots and stems, and higher proportions were translocated to leaves, under P deficiency in P-efficient cultivars than inefficient cultivars (Snapp and Lynch Citation1996).
Root architecture and morphology can greatly affect the ability of a plant to acquire nutrients from soil (Hammond and White 2007). In our current study, XZ99 and ZD9 increased their root surface area and number of root tips in response to LP stress. Interestingly, the average root diameter was increased more in XZ100 than XZ99 under LP. A previous study revealed that P-efficient plants reduced root diameter under LP stress, while absorptive surface area relative to root volume increased for Pi acquisition (Lynch and Brown Citation2006). Thus, it may be suggested that XZ99 has the ability to develop new roots for greater exploration for P during P starvation. Similarly, it has also been confirmed that in Brassica species, root systems with higher ratios of surface area to volume could more effectively explore for nutrients in the soil (Akhtar et al. Citation2008).
Sucrose is one kind of major end product in photosynthetic carbon fixation, and acts as a substrate for energy metabolism and the biosynthesis of complex carbohydrates (Halford et al. Citation2011). It was found that sucrose can regulate plant growth and development in response to biotic and abiotic stresses (Hammond and White 2007). For instance, SPS converts triose phosphate, the end product of photosynthesis, to sucrose (Stitt et al. Citation1987), which is the preferred form of carbon in most C3 plants. This sucrose is then cleaved in the apoplast by cell wall-bound AI, in the cytosol by SuSy or inside vacuoles by soluble AI. In our current study, the activities of AI, SuSy and SPS were all significantly increased in XZ99 under LP (). Under long-term P starvation, enormous accumulation of starch in the leaf occurs, and meanwhile the transport and synthesis of sucrose will be inhibited, resulting in inhibited plant growth and development (Wissuwa et al. Citation2005). The increased loading of sucrose to the phloem under P deficiency primarily functions to relocate carbon resources to the roots, which increases root size relative to the shoot in order to acquire P efficiently (Hermans et al. Citation2006). It may be assumed that the higher tolerance of XZ99 to LP stress is related to its greater sucrose synthesis in leaves and allocation in roots. These findings further strengthen the evidence that in response to P starvation XZ99 plants modified their root architecture.
The results of the experiments reported here show that LP stress increases intracellular APase activity in leaves of XZ99 but not in its roots (). Meanwhile, the depressive effect of LP on enzyme activity was more pronounced in roots of XZ99 than of XZ100, whereas ZD9 showed the opposite results, i.e. the enzyme was enhanced in roots and reduced in shoots. Xiao et al. (Citation2006) reported that the APase activity in roots was much higher in the Arabidopsis thaliana plants when subjected to LP condition than those under normal condition. Thus, it may be assumed that the acid phosphatase is highly associated with P use efficiency through enhancing remobilization or reutilization of existing P in the metabolically less active tissues, such as the senescent leaves.
H+-ATPase is a universal electrochemical H+ pump, which uses ATP as an energy source, being essential for plant adaptation to stress (Palmgren and Harper Citation1999). In this study, LP stress increased the leaf/root H+-ATPase activity in all genotypes, with that in XZ99 being more pronounced (). The key function of this enzyme is to generate an electrochemical proton (H+) gradient, driving ion and metabolite transport across the cell membrane via secondary transport systems (Palmgren and Harper Citation1999). It was reported that plasma membrane H+-ATPase had an important role in phosphorus acquisition (Yan et al. Citation2002). On the other hand, the alteration in activities of Ca2+ Mg2+-ATPase in chloroplasts is fundamental for plant functioning under stressful conditions. We found that XZ99 maintained a greater activity of the leaf and root Ca2+ Mg2+-ATPase in response to LP stress. It can be assumed that changes in cytosolic Ca2+ activities and their modulation in the course of the activity of specific Ca2+-ATPases play a key role in both response and adaptation of plants to Pi starvation (Raghothama Citation1999). In addition, plasma membrane Na+K+-ATPase are well-developed P-type membrane transport proteins, which combine the energy derived from ATP hydrolysis to force transport of solutes against their electrochemical gradients and are involved in transport of protons (Dattagupta et al. Citation2009). In XZ99, compared to XZ100 and ZD9, the activities of leaf/root Na+K+-ATPase were more increased in response to LP (). Our previous studies showed an increase of the Na+K+-ATPase in barley plants subjected to drought and salinity stresses (Ahmed et al. Citation2013).
The secretion of OAs from roots can be activated by multiple mechanisms in response to nutritional stresses including P limitation (Ryan et al. Citation2001). It was found that the amount and composition of OAs in the rhizosphere varied with plant species and P status of growth medium as well as the growth stage of the plant (Lambers et al. Citation2006). P-efficient plant species or cultivars secrete larger amounts of P-mobilizing OAs under LP stress, such as barley (Gahoonia et al. Citation2000), maize (Hinsinger Citation2001), rape (Brassica napus L.) (Zhang et al. Citation2011) and Arabidopsis (Narang et al. Citation2000), resulting in alleviation of P stress. This observation implied that an LP-tolerant genotype XZ99 had the lowest contents of all three OAs including citrate, succinate and malate under CK conditions, and the highest contents under LP stress. Besides, a moderately tolerant genotype ZD9 showed less increase in citrate and succinate, and showed little change in malate, under LP in comparison with the control. In XZ100, malate was the predominant organic acid exuded from the roots, and exudation of citrate and succinate was limited. These results suggest that the carboxylation of phosphoenol pyruvate to oxaloacetate by PEP carboxylase might be limited in XZ100 roots under LP conditions, so that the biosynthesis and breakdown of citrate and succinate in XZ100 was decreased. Thus it may be assumed that the higher tolerance of LP stress in XZ99 is attributed to greater secretion of citrate and succinate in roots. Further analysis of the differential regulation of the activity of the enzymes involved in organic acid metabolism is required to understand this.
In conclusion, our results showed that wild barley genotype XZ99 is more tolerant to LP stress than XZ100 and ZD9, and this tolerance is linked with more developed roots, enhanced sucrose biosynthesis and hydrolysis of carbohydrate metabolism pathway. In addition, higher activities of APase and ATPase, and more secretion of citrate and succinate in roots, are involved in adaptation and tolerance to LP stress, thus indicating that the Tibetan barley accession is valuable for barley improvement. However, further research is needed to better understand the mechanisms of plant adaptation to an LP environment.
ACKNOWLEDGMENTS
This research was supported by the National Natural Science Foundation of China (31330055, 31171544) and the China Agriculture Research System (CARS-05).
REFERENCES
- Able JA, Langridge P, Milligan AS 2007: Capturing diversity in the cereals: many options but little promiscuity. Trends Plant Sci, 12, 71–79. doi:10.1016/j.tplants.2006.12.002
- Ahmed IM, Cao FB, Zhang M, Chen X, Zhang GP, Wu FB 2013: Difference in yield and physiological features in response to drought and salinity combined stress during anthesis in Tibetan wild and cultivated barleys. PloS One. 8(10), e77869. doi:10.1371/journal.pone.0077869
- Akhtar M, Oki Y, Adachi T 2008: Genetic Variability in phosphorus acquisition and utilization efficiency from sparingly soluble P sources by brassica cultivars under P-stress environment. J. Agron. Crop Sci. 194, 380–392. doi:10.1111/j.1439-037X.2008.00326.x
- Bozzo GG, Raghothama KG, Plaxton WC 2002: Purification and characterization of two secreted purple acid phosphatase isozymes from phosphate-starved tomato (Lycopersicon esculentum) cell cultures. Euro. J. Biochem. 269, 6278–6286. doi:10.1046/j.1432-1033.2002.03347.x
- Bradford MM 1976: A rapid and sensitive method for the quantitation of microgram quantities of protein utilizing the principle of protein-dye binding. Anal. Biochem. 72, 248–254. doi:10.1016/0003-2697(76)90527-3
- Chapman HD, Pratt PF 1961: Methods of Analysis for Soils, Plants and Waters, pp. 160–170. University of California, Berkeley, CA.
- Ciereszko I, Barbachowska A 2000: Sucrose Metabolism in leaves and roots of bean (Phaseolus vulgaris L.) during phosphate deficiency. J. Plant Physiol. 156, 640–644. doi:10.1016/S0176-1617(00)80225-4
- Ciereszko I, Janonis A, Kociakowska M 2002: Growth and metabolism of cucumber in phosphate-deficient conditions. J. Plant Nutr. 25, 1115–1127. doi:10.1081/PLN-120003943
- Dai F, Nevo E, Wu DZ, Comadran J, Zhou M, Qiu L, Chen ZH, Beiles A, Chen G, Zhang GP 2012: Tibet is one of the centers of domestication of cultivated barley. Proc. Natl. Acad. Sci. U.S.A., 109, 16969–16973. doi:10.1073/pnas.1215265109
- Dattagupta S, Redding M, Luley K, Fisher C 2009: Comparison of proton-specific ATPase activities in plume and root tissues of two co-occurring hydrocarbon seep tube worm species Lamellibrachia luymesi and Seepiophila jonesi. Marine Biol. 156, 779–786. doi:10.1007/s00227-009-1132-2
- Gahoonia TS, Asmar F, Giese H, Gissel-Nielsen G, Nielsen NE 2000: Root-released organic acids and phosphorus uptake of two barley cultivars in laboratory and field experiments. Euro. J. Agron. 12, 281–289. doi:10.1016/S1161-0301(00)00052-6
- Gahoonia TS, Nielsen NE 2004: Root traits as tools for creating phosphorus efficient crop varieties. Plant Soil. 260, 47–57. doi:10.1023/B:PLSO.0000030168.53340.bc
- Halford N, Curtis T, Muttucumaru N, Postles J, Mottram D 2011: Sugars in crop plants. Ann. Appl. Biol. 158, 1–25. doi:10.1111/j.1744-7348.2010.00443.x
- Hammond JP, White PJ 2008: Sucrose transport in the phloem: integrating root responses to phosphorus starvation. J. Exp. Bot. 59, 93–109. doi:10.1093/jxb/erm221
- Hermans C, Hammond JP, White PJ, Verbruggen N 2006: How do plants respond to nutrient shortage by biomass allocation?. Trends Plant Sci. 11, 610–617. doi:10.1016/j.tplants.2006.10.007
- Hinsinger P 2001: Bioavailability of soil inorganic P in the rhizosphere as affected by root-induced chemical changes: a review. Plant Soil. 237, 173–195. doi:10.1023/A:1013351617532
- Lambers H, Shane MW, Cramer MD, Pearse SJ, Veneklaas EJ 2006: Root structure and functioning for efficient acquisition of phosphorus: matching morphological and physiological traits. Annl. Bot. 98, 693–713. doi:10.1093/aob/mcl114
- López-Bucio J, de la Vega OM, Guevara-García A, Herrera-Estrella L 2000: Enhanced phosphorus uptake in transgenic tobacco plants that overproduce citrate. Nat. Biotech. 18, 450–453. doi:10.1038/74531
- Lynch JP 2007: Roots of the second green revolution. Aus. J. Bot. 55, 493–512. doi:10.1071/BT06118
- Lynch JP, Brown KM 2006: Whole Plant Adaptations to Low Phosphorus Availability. Plant–Environment Interactions. CRC, Boca Raton, 209–242.
- Lynch JP, Brown KM 2008: Root strategies for phosphorus acquisition. In: JP Hammond, PJ White, (ed), The ecophysiology of plant–phosphorus interactions, pp. 83–116. Dordrecht: Springer.
- Nadira UA, Ahmed IM, Zhu B, Zeng J, Cai S, Wu FB, Zhang GP 2014: Difference in response to low phosphorus stress among Tibetan wild barley genotypes. J. Food Agric. Environ. 12, 408–414.
- Narang RA, Bruene A, Altmann T 2000: Analysis of phosphate acquisition efficiency in different Arabidopsis accessions. Plant Physiol. 124, 1786–1799. doi:10.1104/pp.124.4.1786
- Nolte KD, Koch KE 1993: Companion-cell specific localization of sucrose synthase in zones of phloem loading and unloading. Plant Physiol. 101, 899–905.
- Ozturk L, Eker S, Torun B, Cakmak I 2005: Variation in phosphorus efficiency among 73 bread and durum wheat genotypes grown in a phosphorus-deficient calcareous soil. Plant Soil. 269, 69–80. doi:10.1007/s11104-004-0469-z
- Palmgren MG, Harper JF 1999: Pumping with plant P-type ATPases. J. Exp. Bot. 50, 883–893. doi:10.1093/jxb/50.Special_Issue.883
- Qingren W, Jiyun L, Zhensheng L 1999: Adapted responses in the rhizosphere of P-efficient wheat genotype to stress of phosphorus deficiency. Acta Bota. Boreali-Occidentalia Sinica. 20, 1–7.
- Quick W 1996: Sucrose Metabolism in Sources and Sinks. Photoassimilate Distribution in Plants and Crops: Source-sink Relationships, pp. 115–156. CRC Press, Boca Raton, Florida.
- Raghothama K 1999: Phosphate acquisition. Annl. Rev. Plant Biol. 50, 665–693. doi:10.1146/annurev.arplant.50.1.665
- Ruan YL, Llewellyn DJ, Furbank RT 2003: Suppression of sucrose synthase gene expression represses cotton fiber cell initiation, elongation, and seed development. Plant Cell Online. 15, 952–964. doi:10.1105/tpc.010108
- Ryan P, Delhaize E, Jones D 2001: Function and mechanism of organic anion exudation from plant roots. Annl. Rev. Plant Physiol. Plant Mole. Biol. 52, 527–560. doi:10.1146/annurev.arplant.52.1.527
- Snapp SS, Lynch JP 1996: Phosphorus distribution and remobilization in bean plants as influenced by phosphorus nutrition. Crop Sci. 36, 929–935. doi:10.2135/cropsci1996.0011183X003600040019x
- Stitt M, Huber S, Kerr P 1987: Control of photosynthetic sucrose formation. In The Biochemistry of Plants, Vol. 10, Photosynthesis, Ed .MD Hatch, NK Boardman, pp. 327–409. Academic Press, San Diego, CA.
- Tanaka A, Hitsuda K, Tsuchihashi Y 1984: Tolerance to low pH and low available phosphorus of various field and forage crops. Soil Sci. Plant Nutr. 30, 39–49. doi:10.1080/00380768.1984.10434666
- Vance CP, Uhde-Stone C, Allan DL 2003: Phosphorus acquisition and use: critical adaptations by plants for securing a nonrenewable resource. New Phytol. 157, 423–447. doi:10.1046/j.1469-8137.2003.00695.x
- Wissuwa M, Gamat G, Ismail AM 2005: Is root growth under phosphorus deficiency affected by source or sink limitations?. J. Exp. Bot. 56, 1943–1950. doi:10.1093/jxb/eri189
- Wu FB, Zhang GP, Dominy P 2003: Four barley genotypes respond differently to cadmium: lipid peroxidation and activities of antioxidant capacity. Environ. Exp. Bot. 50, 67–78. doi:10.1016/S0098-8472(02)00113-2
- Xiao K, Katagi H, Harrison M, Wang ZY 2006: Improved phosphorus acquisition and biomass production in Arabidopsis by transgenic expression of a purple acid phosphatase gene from M. truncatula. Plant Sci. 170, 191–202. doi:10.1016/j.plantsci.2005.08.001
- Yan F, Zhu Y, Müller C, Zörb C, Schubert S 2002: Adaptation of H+-pumping and plasma membrane H+ ATPase activity in proteoid roots of white lupin under phosphate deficiency. Plant Physiol. 129, 50–63. doi:10.1104/pp.010869
- Yaseen M, Malhi SS 2011: Exploitation of genetic variability among wheat genotypes for tolerance to phosphorus deficiency stress. J. Plant Nutr. 34, 665–699. doi:10.1080/01904167.2011.540623
- Zhang H, Huang Y, Ye X, Xu F 2011: Genotypic variation in phosphorus acquisition from sparingly soluble P sources is related to root morphology and root exudates in Brassica napus. Sci. China Life Sci. 54, 1134–1142. doi:10.1007/s11427-011-4254-y