ABSTRACT
The Codex Alimentarius Commission has recently adopted maximum levels for inorganic arsenic (As; in 2014) and total cadmium (Cd; in 2006) in polished rice grains to maintain food safety and to decrease the risk to human health. As rice is a staple crop in Japan and monsoon Asian countries, reducing concentrations of As and Cd in rice is an urgent matter. In flooded conditions, Cd concentration in soil solution decreases whereas As concentration increases. Therefore, we aimed to evaluate the efficiency of iron-bearing materials to decrease As concentration in soil solution and rice (Oryza sativa L.) grain under submerged cultivation, while also considering Cd concentration. In experiments conducted in paddy fields in six regions, As concentrations in the soil solution during the cultivation period decreased in the following order: control (REF) > steel converter furnace slag (SCS) > non-crystalline iron hydroxide (FH) > zero-valent iron (ZVI). The concentrations of As in brown rice were in the same order, with ZVI achieving particularly strong reduction. Cadmium concentrations were low, probably owing to submerged cultivation conditions. Application of iron-bearing materials slightly and insignificantly reduced the yields of brown rice and straw. Application of these materials did not have a significant negative impact on the quality of rice. Our data indicate that the application of iron-bearing materials effectively reduces As concentrations in soil solution and rice grains without negative effects on yield and quality, with a particularly powerful effect of ZVI which is possibly explained by arsenic sulfide formation.
KEY WORDS:
1. Introduction
Fast industrialization in Japan during the 1960s caused serious soil pollution by heavy metals and metalloids, such as cadmium (Cd) and arsenic (As). This pollution prompted the establishment of the Act to Prevent Soil Contamination on Agricultural Land in 1970 to control contamination of farms by hazardous materials including As and Cd. The Codex Alimentarius Commission adopted maximum levels for Cd (0.4 mg kg‒1) and inorganic As (0.2 mg kg‒1) in polished rice (Codex Alimentarius Commission Citation2006, Citation2014). According to surveillance of rice (Oryza sativa L.) produced in Japan in 2014, the mean concentration of inorganic arsenic (iAs) in polished rice was 0.12 mg kg‒1 with a maximum value of 0.26 mg kg‒1 (MAFF Citation2014). The intake of iAs causes a significant risk of cancer for people, as As is a carcinogen (IARC Citation2004). Given that 34–50% of Cd intake and 62% of iAs intake by Japanese people comes from rice (Kawada and Suzuki Citation1998; Oguri et al. Citation2014), reducing the concentrations of Cd and As in rice grains is a high priority.
Water management is a popular and cost-effective practice for alleviating rice Cd contamination in Japan (Bolan et al. Citation2013). Cadmium absorption by rice was effectively decreased by continuous submergence of the paddy field for 3 weeks before and 3 weeks after heading (Arao et al. Citation2009). This can be explained by the formation of Cd sulfide (De Livera et al. Citation2011) and/or Cd carbonate (Khaokaew et al. Citation2011a; Kosolsaksakul et al. Citation2014) under reduced soil conditions; these compounds are hardly soluble, which reduces the availability of soil Cd to rice plants. In contrast, continuous submergence and therefore reduced soil conditions cause the reduction of As from arsenate (As(V)) to arsenite (As(III)) in soil solids (Ohtsuka et al. Citation2013), leading to a release of As into the soil solution due to lower adsorption of As(III) than of As(V).
As(V) forms an inner-sphere complex with iron (Fe) oxides (Waychunas et al. Citation1993; Fendorf et al. Citation1997; Sherman and Randall Citation2003), whereas As(III) forms an outer-sphere complex (Goldberg and Johnston Citation2001) and multiple inner-sphere complexes (Ona-Nguema et al. Citation2005). Reductive dissolution of iron (hydr)oxides facilitated by the activity of Fe-reducing bacteria also promotes As dissolution, because iron (hydr)oxides adsorb As in soils (Nickson et al. Citation2000).
Water-saving cultivation to produce aerobic conditions is one of the promising methods to decrease As concentration in soil solution (Nakamura and Katou Citation2012) and rice grains (Arao et al. Citation2009; Hu et al. Citation2013). Thus, there is a tradeoff relation between the bioavailability of As and that of Cd, which limits the opportunities to solve the problem by optimizing water management alone, although a recent study (Honma et al. Citation2016) suggested that intermittent irrigation with an appropriate irrigation interval could simultaneously reduce the accumulation of As and Cd in rice grain. An alternative, promising strategy is a combination of water management (either submerged cultivation to reduce Cd absorption or water-saving cultivation to reduce As uptake) with soil amendment to reduce absorption of As or Cd, respectively.
A variety of materials to treat water contaminated with As have been reported, including various oxides (Hua et al. Citation2012), treated slags, carbons derived from agricultural waste (char carbons and coconut husk carbons), biosorbents (immobilized biomass, orange juice residue), goethite, and some commercial adsorbents, which include resins, gels, silica and treated silica (Mohan and Pittman Citation2007).
Iron-bearing materials have high As adsorption capacity (Suda et al. Citation2015). Due to the high affinity of As for Fe oxides, such amendments have been extensively studied for remediation of As-contaminated soils (Komárek et al. Citation2013). Ferrihydrite, a short-range ordered iron hydroxide common in soils, has a high specific surface area and high adsorption capacity (Komárek et al. Citation2013). Zero-valent iron (ZVI) also effectively removes As(III) and As(V) from water (Bang et al. Citation2005; Sun et al. Citation2006), and decreases As in soil water and in plant shoots (Kumpiene et al. Citation2006a).
Our previous report revealed that application of Fe materials, including ZVI, non-crystalline iron hydroxide (FH) and steel converter furnace slag (SCS), to paddy fields resulted in a significant decrease of the As concentration in rice grain and straw (Matsumoto et al. Citation2015a). In this study, we applied these three iron materials to paddy fields with submerged cultivation and examined the possibilities for simultaneous decrease in As and Cd in rice grains. The effects were analyzed in relation to As concentration in soil solution, and yield and quality of brown rice.
2. Materials and methods
2.1. Soils
Six paddy fields (sites A–F) in Japan were used for field cultivation experiments (). The soil types of the fields are diverse and include Gley Lowland soils, a Gray Lowland soil, a Gray Upland soil, and an Andosol (Cultivated Soil Classification Committee Citation1995).
Table 1. Soil properties.
Soils were sampled from the plowed layer of these fields. All samples were air-dried at room temperature and passed through a 2-mm mesh sieve before use. Soils were suspended at a 1:5 ratio and the suspension pH (soil pH) was measured with a pH meter with a glass electrode. Carbon and nitrogen contents were analyzed by the dry combustion method (Makino et al. Citation2006). Cation exchange capacity (CEC) was measured by the continuous leaching technique with 1 mol L−1 ammonium acetate buffer (pH 7) (Sumner and Miller Citation1996). Available phosphorus and phosphate adsorption coefficient to soil (PAC) were determined according to the methods of Truog (Citation1930) and Araki et al. (Citation1986), respectively. Two selective Fe dissolution experiments were carried out, which used either acid ammonium oxalate (for non-crystalline iron) or dithionite citrate (for free iron oxide) (Blakemore et al. Citation1981). Available silicate was leached in an aqueous solution with an incubation test (Nonaka and Takahashi Citation1988) and exchangeable Mn (Gambrell Citation1996) was extracted with 1 mol L−1 ammonium acetate solution, respectively, with a minor modification. The extracts were analyzed with inductively coupled plasma-optical emission spectrometry (ICP-OES) (Vista-Pro, Varian, Palo Alto, CA, USA). Acid-extractable As and Cd were extracted at a soil-to-solution ratio of 1:10 by 1 mol L−1 and 0.1 mol L−1 hydrochloric acid (HCl) for As and Cd, respectively. The concentrations of As and Cd in the extracts were measured by ICP-OES at wavelengths of 188.980 nm (As) and 214.439 nm (Cd).
2.2. Cultivation design
The cultivation design is summarized in . Rice plants were cultivated in four of the six fields in 2013 and in the remaining two fields in 2014 (). Each field was separated into 12 small plots for four treatments in triplicate. Treatments included three kinds of iron-bearing materials (see below) and a reference (REF: no material) with a randomized block design.
Table 2. Cultivation design.
2.3. Iron-bearing materials
We used three types of iron-bearing materials: ZVI (steel powder 53NJ; Kobe Steel, Ltd., Tokyo, Japan), FH (Fix-All FB, Ishihara Sangyo Kaisha, Ltd., Osaka, Japan), and SCS (FM gorudo, Yoneda Industry Co., Okayama, Japan). ZVI differed from the one used in our previous study (metal Fe powder), whereas the other two materials were the same (Matsumoto et al. Citation2015b). The crystalline structure of FH is similar to that of ferrihydrite (5Fe2O3·9H2O), according to X-ray diffraction analysis (data not shown). The iron contents were as follows: ZVI, 98%; FH, 64%; SCS, 20%. Because the iron content and price of SCS are substantially lower than those of ZVI and FH, we applied 1 kg m−2 each of ZVI and FH, and 2 kg m−2 of SCS.
2.4. Soil redox potential
Soil redox potential (Eh) was measured at 5- and 15-cm depths in duplicate. Platinum electrodes (Hirose Rika, Ltd.) were used in two of the three replicate plots and a silver (Ag)/silver chloride (AgCl) reference electrode (4400, DKK-TOA Co.) was used in each plot. All electrodes were connected to a data logger (CR1000; Campbell Sci., Inc.) with a multiplexer (AM16/32; Campbell Sci., Inc.); the data were collected hourly. The potential against the reference electrode was converted to the corresponding potential for a hydrogen electrode. The results of soil redox potential are presented in the supporting information (S1-1–S1-5).
2.5. Sampling and analysis of soil solution
The soil solution sampler was composed of a 10-cm porous part (OD 2.5 mm) and a connected polyethylene/polyvinyl chloride tube (10 Rhizon MOM 10 cm; Rhizosphere Research Products, Wageningen, The Netherlands); a polyvinyl chloride extension tube (PF; TOP Co., Ltd., Tokyo, Japan); and an evacuated plastic tube (BD Vacutainer Serum; Becton, Dickinson and Company, NJ, USA in 2013 and Venoject II, TERUMO Co., Japan, in 2014). The fibers were set parallel to the ground surface at the center of the intersection between inter-row and intra-row spacing, at a soil depth of 5 cm, in every plot except site D. For first sampling, vacant evacuated tubes were connected to the extension tubes, soil solution was collected by suction, and the tubes were removed. This first sampling is for determination of soil solution pH. Then, a second sample of soil solution was collected again into a tube with nitric acid (used to prevent the precipitation of iron (hydr)oxide during sampling). Before the second sampling, nitric acid (0.66 mL, 10 wt %) was added to pre-weighed evacuated plastic tubes using a plastic syringe with a stainless needle. Net weights of the tubes were calculated by subtracting the initial weights of those tubes.
Because the fibers work as a micro-membrane (pore size < 0.2 μm) and would retain particles, membrane filtration was not conducted. Soil solution was sampled five times: (1) just before midseason drainage; (2) after midseason drainage; (3) 2 weeks before heading; (4) at heading; and (5) 2–3 weeks after heading.
Concentrations of As and Cd in soil solution were measured by flow-injection inductively coupled plasma mass spectrometry (FI-ICPMS) (Flexar HPLC System; Perkin Elmer NexION, 300XX ICP-MS; PerkinElmer, Inc. MA, USA) with 1 wt % nitric acid as a mobile phase at a flow rate of 1 mL min−1 with O2 gas in DRC mode. Indium was used as an internal standard.
2.6. Rice yield and grain quality
Mature rice was harvested from each plot. Shoots were cut by hand (at the bottom of the stem), air-dried and weighed. Husked grains (brown rice) from the air-dried shoots were sieved through a 1.8- or 1.9-mm mesh to remove small grains and weighed to evaluate grain yield. Brown rice yield was expressed at a standard moisture content of 150 g kg−1. Perfect grain percentages (a commonly used index for rice grain quality) were measured with a rice inspector (RGQI10B; Satake Co. Higashihiroshima, Japan). This index refers to whole rice grains left after removing cracked, broken, dead and immature rice grains, and grains of other species.
2.7. Elemental analysis of rice grains
Grains were placed in a 50-mL plastic tube with a 20-mm stainless steel ball and finely ground using a shaking mill (Shake Master; Bio Medical Science Inc., Tokyo, Japan). To decompose the rice grain, 0.2 g of the powder was mixed with 5 mL of nitric acid in a 50-mL polypropylene tube and incubated overnight at room temperature The temperature was then raised to 105°C within 1 h and kept for 2 h using a Hot Block Acid Digestion System (DigiPREP MS; SCP Sciences, Inc., Canada) After the sample cooled, 1 mL of 30% hydrogen peroxide solution was added, and the mixture was heated again for 1 h at 105°C. After the dilution with water, concentrations of As and Cd were measured as described above for soil solution.
2.8. Statistical analysis
Statistical analysis were conducted with an add-in program for MS Excel (Ekuseru-Toukei 2012; Social Survey Research Information Co., Ltd., Japan). Two-way repeated-measures analysis of variance (two-way ANOVA) with sites and treatments as factors was used to analyze As concentration in soil solution and rice grains, Cd concentration in rice grains, rice grain yield and perfect grain percentage. The Tukey–Kramer multiple comparison test was used to detect significant differences.
3. Results
3.1. Soil properties
Soil pH (H2O) ranged from 5.62 (slightly acidic) to 6.79 (neutral) (). The contents of total carbon, CEC, PAC, free iron oxide and non-crystalline iron (the latter is related to the soil adsorption capacity) were considerably higher in soils A, D and F than in soils B, C and E. The values were particularly high for soil F (except for free iron oxide), probably because this soil (Andosol) has unique properties such as high amounts of organic matter and non-crystalline minerals, and low bulk density. The typical clay minerals in Andosols, allophane and ferrihydrite, have high anion adsorption capacity. In Japan, the PAC value ≥ 15 g diphosphorus pentoxide (P2O5) kg−1 is one of the criteria for Andosol. Available Si was the highest in soil D (and nearly the same in soil E), followed by C, F, A and B. The difference between the highest and the lowest values was almost 3-fold.
3.2. Changes of Eh during cultivation period
Arsenic and Cd are effectively taken up by rice plants from soil solution and are distributed to grains during the 3 weeks before and after heading (Arao et al. Citation2009). Over this 6-week period, soil Eh at all sites was mostly low and stable (−200 mV) during the 3 weeks before and after heading (S1), suggesting high availability for As and low availability for Cd.
3.3. Overall trends among sites and treatments analyzed by two-way ANOVA
The results of two-way ANOVA with sites and treatments as factors, and Tukey–Kramer multiple comparison tests, are summarized in –. Site had significant effects on all of the parameters analyzed: As and Fe in soil solution, As and Cd in rice grains, brown rice yield, stem yield and perfect grain percentage. This indicates that our experimental sites were sufficiently different to evaluate the effects of application of the iron-bearing materials. Significant effects of material were observed on As in soil solution and in rice grain, and on perfect grain percentage, but not on rice Cd or grain yield. With the exception of Fe and Si in soil solution (), the effects of the interaction between the site and material (or the absence of significance) were detected (–). The data at each site of every treatment are shown in the supplemental figures (S2–3 and S5-9), with the results of statistical treatment by multiple range tests for the comparison of any pair of the data (S4 and S10), if the interaction between “Site and Material” has been detected.
Table 3. Two-way analysis of variance (ANOVA) of arsenic (As), iron (Fe) and silicon (Si) concentration and pH of soil solution during cultivation period in each site and material.
Table 4. Two-way analysis of variance (ANOVA) of arsenic (As) and cadmium (Cd) concentration in rice (Oryza Sativa L.) grain and rice stem.
Table 5. Two-way analysis of variance (ANOVA) of yield and whole grain ratio.
3.4. Concentrations of arsenic, iron and cadmium in soil solution
The mean As concentration in soil solution (As-sol) during the whole cultivation period, five sampling times, at each site decreased in the following order: A > C ≈ B > E > F, with significant differences between A and C, and between B and E according to the Tukey–Kramer multiple comparison test (). Iron-bearing materials reduced the mean As-sol values in the following order: REF > SCS > FH >> ZVI, with no significant difference between REF and SCS (). ZVI was the most efficient in reducing As-sol.
High correlations were found between As-sol and Fe concentrations in soil solution (Fe-sol) in all treatments and sites, except ZVI at site A and SCS at site E (). These results are consistent with the reported high correlation between As-sol and Fe in paddy soils without material addition (Yamaguchi et al. Citation2011).
Figure 1. (a) Relationship between iron (Fe) and arsenic (As) concentrations in soil solution in (a) Site A, (b) Site B, (c) Site C, (d) Site E and (e) Site F (soil depth: 5 cm). REF: reference, no treatment; FH: non-crystalline iron hydroxide; SCS: steel converter furnace slag; ZVI: zero-valent iron.
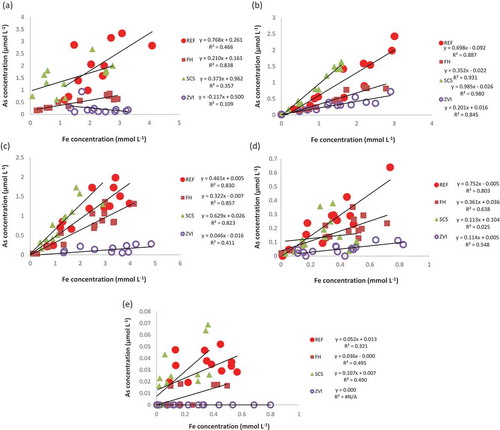
The slopes of the linear regression lines () show the ratio of the increase of As-sol to that of Fe-sol (ΔAs/ΔFe), which tended to decrease in the following order: REF ≈ SCS > FH >> ZVI. Two-way ANOVA showed no significant differences among treatments (except SCS) in their effects on Fe-sol ().
The concentrations of Cd-sol were too low to analyze under submerged conditions except at site B, where Cd concentration was lower in the SCS treatment than in other treatments, suggesting that the high pH of the materials caused by SCS suppresses Cd solubility from the soil ( and ).
Figure 2. Effect of iron materials on cadmium (Cd) concentration in soil solution at site B during the cultivation period (soil depth: 5 cm). Error bars indicate standard deviation. The numbers on the x-axis indicate sampling times, which are: 1, just before midseason drainage; 2, after midseason drainage; 3, 2 weeks before heading; 4, at heading; and 5, 2–3 weeks after heading. REF: reference, no treatment; FH: non-crystalline iron hydroxide; SCS: steel converter furnace slag; ZVI: zero-valent iron.
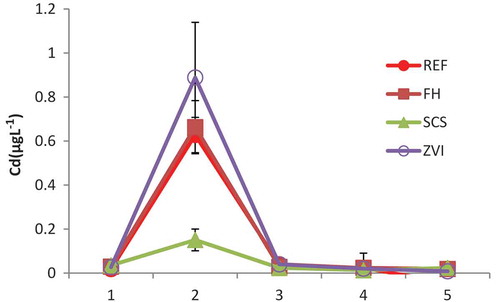
3.5. Arsenic and cadmium concentration in brown rice
The mean iAs concentration in brown rice was the highest at site C followed by sites D, B, A, E and F, with significant differences except between C, D and B (). Application of iron-bearing materials significantly decreased the mean As concentration in brown rice and rice stems in the following order: REF > SCS ≈ FH > ZVI, with significant differences between REF and SCS and between FH and ZVI ().
There was a significant correlation between the average of As-sol (the average amount of As-sol though the three samplings of soil solution around rice heading) and As in brown rice (). The relative decrease of As in rice grain by iron-bearing materials declined with the decrease in As concentration in brown rice (). When the As concentration in brown rice was lower than 0.1 mg kg−1 (as at site F; wet Andosol), the relative decreases of FH and SCS were negative, indicating that As concentrations in brown rice in these treatments were higher than those in REF. The concentration of Cd in brown rice was very low in all samples, reflecting its low concentration in soil solution because of reducing conditions in submerged soil.
3.6. Yield and quality of brown rice
Brown rice yields were highest at site C, followed by B, F, D and A (). The yields were comparable to the average rice yield in Japan (5.36 t ha−1 in 2014; MAFF Citation2014). Site E showed the lowest yield, probably due to low available nitrogen and low sunshine because of its topographical features. The highest yield was obtained in the presence of SCS, but no significant differences were observed among the materials. The perfect grain percentage varied from 48.8 to 86.1% and was significantly different between all sites.
4. Discussion
Ions in soil solution are the most labile and easily available fraction for plants to take up. Therefore, we first focused on the effect of applied iron-bearing materials on ion concentrations in soil solution. Because soil Eh was mostly low and stable (−200 mV) during the 3 weeks before and after heading (S1), the predominant forms of As and Fe would be As(III) and Fe(II), respectively, according to the known relationship between pH and Eh. Reductive dissolution of ferric (hydr)oxide, owing to the activity of Fe-reducing bacteria, resulted in the concomitant dissolution of As adsorbed on ferric (hydr)oxides, which are ubiquitous in soils. Arsenic adsorbed onto ferric (hydr)oxide is concomitantly released into solution due to the loss of the adsorption phase. Under field-relevant conditions, the reduction of As(V) is thermodynamically favored over Fe(III) reduction (Kocar and Fendorf Citation2009). A close correlation between the release of Fe and that of As has been interpreted as evidence of coupled dissolution of As and Fe from the same minerals (Fakih et al. Citation2009; Weber et al. Citation2010). Our data generally showed a significant correlation between As and Fe release from soil (), except ZVI at site A and SCS at site E. The mean As-sol values decreased in the following order, REF > SCS > FH >> ZVI, with no significant difference between REF and SCS (), whereas the mean Fe-sol values did not differ significantly among treatments except for SCS, which was significantly lower (). The mean ratios of the increments in As-sol to those of Fe-sol (ΔAs/ΔFe) tended to decrease in the following order: REF > SCS > FH >> ZVI ().
These data indicate that iron-bearing materials suppress the dissolution of soil iron minerals, followed by a decrease in As dissolution. Reductive dissolution of iron minerals needs an electron donor from some soil constituents; easily reducible organic matter probably plays the primary role. The total amount of easily reducible organic matter in soils is the same before and after application of iron-bearing materials; therefore, the total amount of electron donors would also remain the same. The decrease of electron transfer to soil iron hydroxides due to the application of iron-bearing materials is likely to suppress the dissolution of soil iron minerals on which As is adsorbed. These phenomena may explain the mechanism of the inhibitory effect of iron-bearing materials on As dissolution from soil. To determine the stoichiometry of electron transfer between organic matter and iron (hydr)oxides, the ratio of reduced iron originating from soil iron oxides to that originating from iron-bearing materials should be clarified. The amount of dissolved iron, however, is just a part of reduced iron. Thus, a new method to determine the origin of Fe-sol (from soil vs. from iron-bearing material) is needed to investigate the relation between Fe-sol and As-sol. SCS and FH, which contain iron(III) (hydr)oxides, can support the reaction mechanism mentioned above.
Another possible explanation for As-sol mitigation by iron-bearing materials is that they can re-adsorb the released As, probably through the coordination exchange bond between their surface hydroxyl groups and As.
On the other hand, release of As(III) during reductive dissolution of ferrihydrite is suppressed by the subsequent formation of the secondary Fe mineral (Tufano and Fendorf Citation2008). Because As(III) is adsorbed on siderite (Jönsson and Sherman Citation2008), secondary formation of siderite may prevent Fe and As(III) dissolution, thereby reducing the release of As(III) from soil to solution. With the development of anaerobic condition in a soil incubation test under water-submerged conditions, extended X-ray absorption fine structure (EXAFS) reveled Fe(II) remained in the soil solid phase as the secondary mineral siderite (Yamaguchi et al. Citation2011). The degree of siderite formation is vary depend on soil properties. Transformation to a secondary mineral phase is limited in organic-rich soil horizons (Fakih et al. Citation2009). The precipitation of siderite may have been limited in soils A and F possibly due to the high carbon content (). The low correlation coefficients in , site A and site F, might be attributed to the influence of the high carbon content.
Zero valent iron showed a much lower As-sol concentration than the other treatments did ( and ). Compared to the surfaces of FH and SCS, the surface of ZVI is strongly reduced, and thus ZVI is not expected to decrease soil iron dissolution. Zero valent iron, however, effectively removed As(III) and As(V) from water in batch experiments (Bang et al. Citation2005). It showed that the As(III) removal rate was higher than that for As(V) when iron materials were mixed with arsenic solutions purged with nitrogen gas at pH 4–7. X-ray photoelectron spectroscopy data on the reacted iron compounds showed the reduction of As(III) to As(0) (Bang et al. Citation2005). Batch experiments showed that both As(V) and As(III) could be removed efficiently from simulated groundwater by ZVI under aerobic and relatively anaerobic conditions (Sun et al. Citation2006). Application of ZVI to chromated copper arsenate-contaminated soil decreased As and Cr concentrations in leachates, in soil pore water and in plant shoots (Kumpiene et al. Citation2006b). Zero valent iron is oxidized to ferrous iron under aerobic conditions, resulting in the consumption of dissolved oxygen in water and eventually leading to anoxic conditions. Further iron oxidation may be accompanied by the reduction of dissolved sulfate and As in water and possible formation of the insoluble, stable arsenopyrite (FeAsS) precipitate as mentioned below with minor modification (Ramaswami et al. Citation2001).
Aerobic iron oxidation: 2 Fe° + 2 H2O + O2 → 2 Fe2+ + 4 OH−
Anoxic iron oxidation: Fe° + 2 H2O → Fe2+ + H2 + 2 OH−
Arsenic and sulfate reduction: 14 Fe2+ + SO42− + H3AsO3 + 11 H+ → FeAsS + 13Fe3+ + 7 H2O + 2 e−
Also, ZVI enhanced Cadmium sulfide (CdS) formations and reduced Cd in the exchangeable fraction and in soil solution (Hashimoto and Yamaguchi Citation2013).
These studies and our results suggest that, upon ZVI application, one of the mechanisms to decrease As-sol might be the formation of a hardly soluble arsenic sulfide, probably arsenopyrite. The precise mechanism should be clarified by X-ray absorption near-edge structure spectroscopy, which appears to be a promising method for this purpose. Another possible reaction mechanism is As adsorption through surface iron hydroxyl groups which would be generated by the partial oxidation of the surface iron on ZVI.
The concentration of Cd-sol was too low to analyze, except at site B, indicating that submerged conditions suppressed Cd-sol accumulation. The concentrations of both Fe-sol and Cd in SCS plots were significantly decreased, probably because the pH of soil solution was significantly increased (), which would also suppress the dissolution of soil iron oxides.
It is most likely that a considerable decrease in Cd absorption by rice under submerged conditions is due to a decrease in Cd solubility because of the formation of carbonates (Khaokaew et al. Citation2011b) and/or CdS (De Livera et al. Citation2011; Hashimoto and Yamaguchi Citation2013) as depicted in Eq. 2 and 3 from Lindsay (Citation1979).
(4) CdCO3 (otavite) + 2 H+ = Cd2+ + CO2 (gas) + H2O log K0 = 6.16
(5) CdS (greenockite) = Cd2+ + S2− log K0 = − 27.07
As can be expected from these equations, the formation of Cadmium carbonate (CdCO3) and CdS is favored at high pH and under reducing conditions, respectively. Because of the formation of these sparingly soluble Cd compounds, Cd could not be detected in soil except at site B, where Cd-sol concentration was lowest in SCS treatment ().
4.1. Arsenic and cadmium concentrations in rice plants
The application of iron materials significantly decreased the mean As concentrations in brown rice and rice stems in the following order: REF > SCS ≈ FH > ZVI (), with significant differences between REF and SCS and between FH and ZVI (). These data clearly indicate that iron-bearing materials decrease As concentration in rice plants. Because there was a significant correlation between the average of As-sol and As in brown rice (), the decrease in As-sol may be primarily responsible for mitigation of As accumulation in rice plants. On the other hand, As-sol in SCS tended to be higher than in FH, whereas rice As was similar in SCS and FH ( and ). This difference may be partially attributed to the difference in Si concentrations in soil solution, which was significantly higher in SCS than in the other treatments (). Silicic acid is known to compete with As in terms of plant uptake due to a similar chemical form of silicate and arsenate. Arsenite transport in rice roots shares the same highly efficient pathway as Si; the transporters are Lsi1 and Lsi2 (Ma et al. Citation2008). Silicic acid fertilization decreased the total As concentration in straw and grain, even though Si addition increased As concentration in the soil solution (Li et al. Citation2009). They also reported that Si decreased the iAs concentration in grain. A similar tendency was observed in our results: the proportions of rice iAs to rice As of REF, SCS, FH and ZVI are 79.0, 73.7, 78.5 and 86.5%, respectively. SCS contains a large amount of silicates, which could dissolve into soil solution and suppress As uptake by rice plants.
There was a negative relationship between the decrease ratio of As by iron-bearing materials and As concentration in brown rice (). The same trends were observed in iAs concentration in brown rice (S6) and As concentration in stem (S7). These results suggest that iron-bearing materials are more effective to reduce As uptake by rice plants along with the increase of As availability of soils. Site F (Wet Andosol) had high amounts of non-crystalline iron oxide and carbon, suggesting high As adsorption capacity, which might have masked the effect of iron-bearing materials.
The total iron input was 0.4 kg m−2 for SCS, 0.64 kg m−2 for FH, and 0.98 kg m−2 for ZVI. This order of iron content agrees with that of the decrease ratio of As in rice grains. These results suggest that the iron content could be an important factor for suppression of As dissolution from soils regardless of the chemical form of iron in the materials.
4.2. Rice yield and grain quality
Whereas stem yield significantly decreased in ZVI, there was no negative effect of iron-bearing materials on grain yield or perfect grain percentage (). The significantly higher perfect grain percentage in FH and ZVI than in REF might indicate a positive effect of iron-bearing materials on rice quality. It is well documented that slag-based silicon fertilizers have beneficial effects on the growth and disease resistance of rice (Ning et al. Citation2014). SCS, which is steel converter furnace slag, is expected to provide a positive effect on rice growth and yield with successive applications.
Supplementary Materials (Tables)
Download PDF (64.1 KB)Supplementary Materials (Figures)
Download PDF (270.3 KB)Acknowledgments
This work was supported by a grant from MAFF of Japan (Research project for improving food safety and animal health As-240). We thank E. Amano, F. Ochida, M, Watanabe, H. Ikesugi and Y, Murasaki (NIAES) for their field and laboratory assistance.
Supplemental material
Supplemental data for this article can be accessed here.
References
- Araki S, Hirai H, Kyuma K 1986: Phosphate absorption of red and/or yellow colored soil materials in relation to the characteristics of free oxides. Soil Sci. Plant Nutr., 32, 609–616. http://www.tandfonline.com/doi/abs/10.1080/00380768.1986.10557542.
- Arao T, Kawasaki A, Baba K, Mori S, Matsumoto S 2009: Effects of water management on cadmium and arsenic accumulation and dimethylarsinic acid concentrations in Japanese rice. Environ. Sci. Technol., 43, 9361–9367. http://www.ncbi.nlm.nih.gov/pubmed/20000530.
- Bang S, Johnson MD, Korfiatis GP, Meng X 2005: Chemical reactions between arsenic and zero-valent iron in water. Water Res., 39, 763–770. doi:10.1016/j.watres.2004.12.022
- Blakemore L, Searle P, Daly B 1981: Methods for chemical analysis of soils. New Zealnd Soil Bureau Scientific Report 10A. Methods for chemical analysis of soils. New Zealnd Soil Bureau Scientific Report 10A. New Zealand.
- Bolan N, Makino T, Kunhikrishnan A, Kim P-J, Ishikawa S, Murakami M, Naidu R, Kirkham M 2013: Cadmium contamination and its risk management in rice ecosystems. In Advances in Agronomy Volume 119, Ed. Sparks DL, pp. 183–273. San Diego, CA, Elsevier.
- Codex Alimentarius Commission 2006: Report of the 29th session of the CODEX Alimentarius Commission. Report of the 29th session of the CODEX Alimentarius Commission.
- Codex Alimentarius Commission 2014: Joint FAO/WHO food standards program, codex alimentarius commission, Thirty–seventh Session, CICG, Geneva, Switzerland 14–18 July 2014.
- COMMITTE CSC 1995: Classification of cultivated soils in Japan third approximation. Misc. Publ. Natl. Inst. Agro-Environ. Sci., 17, 1–79.
- De Livera J, McLaughlin MJ, Hettiarachchi GM, Kirby JK, Beak DG 2011: Cadmium solubility in paddy soils: effects of soil oxidation, metal sulfides and competitive ions. Sci. Total Environ., 409, 1489–1497. http://www.sciencedirect.com/science/article/pii/S0048969710013598 (October, 2015).
- Fakih M, Davranche M, Dia A, Nowack B, Morin G, Petitjean P, Châtellier X, Gruau G 2009: Environmental impact of As(V)–Fe oxyhydroxide reductive dissolution: an experimental insight. Chem. Geol., 259, 290–303. http://linkinghub.elsevier.com/retrieve/pii/S0009254108005354 (December, 2014).
- Fendorf S, Eick MJ, Grossl P, Sparks DL 1997: Arsenate and chromate retention mechanisms on goethite. 1. Surface Structure. Environ. Sci. Technol., 31, 315–320. http://pubs.acs.org/doi/abs/10.1021/es950653t.
- Gambrell RP 1996: Manganese. In Methods of Soil Analysis Part3. Chemical methods, Ed. Sparks DL, pp. 665–682. Soil Science Society of America, Inc., Madison, WI.
- Goldberg S, Johnston CT 2001: Mechanisms of arsenic adsorption on amorphous oxides evaluated using macroscopic measurements, vibrational spectroscopy, and surface complexation modeling. J. Colloid Interface Sci., 234, 204–216. http://www.sciencedirect.com/science/article/pii/S0021979700972953 (November, 2015).
- Hashimoto Y, Yamaguchi N 2013: Chemical speciation of cadmium and sulfur K-edge XANES spectroscopy in flooded paddy soils amended with Zerovalent iron. Soil Sci. Soc. Am. J., 77, 1189–1198. <Go to ISI>://WOS:000322083200008. doi:10.2136/sssaj2013.01.0038
- Honma T, Ohba H, Kaneko-Kadokura A, Makino T, Nakamura K, Katou H 2016: Optimal soil Eh, pH, and water management for simultaneously minimizing arsenic and cadmium concentrations in rice grains. Environ. Sci. Technol., 50, 4178–4185. http://dx.doi.org/10.1021/acs.est.5b05424 (March, 2016).
- Hu P, Huang J, Ouyang Y et al. 2013: Water management affects arsenic and cadmium accumulation in different rice cultivars. Environ. Geochem. Health, 35, 767–778. http://link.springer.com/10.1007/s10653-013-9533-z.
- Hua M, Zhang S, Pan B, Zhang W, Lv L, Zhang Q 2012: Heavy metal removal from water/wastewater by nanosized metal oxides: A review. J. Hazard. Mater., 211-212, 317–331. http://linkinghub.elsevier.com/retrieve/pii/S0304389411012453.
- International Agency for Research on Cancer (IARC) Working Group on the Evaluation of Carcinogenic Risks to Humans 2004: Some Drinking-water Disinfectants and ontaminants,388 Including Arsenic. IARC Monographs on the Evaluation of Carcinogenic Risks to Humans, vol. 84. World Health Organization (WHO), Lyon. http://monographs.iarc.fr/ENG/Monographs/vol84/mono84.pdf.
- Jönsson J, Sherman DM 2008: Sorption of As(III) and As(V) to siderite, green rust (fougerite) and magnetite: implications for arsenic release in anoxic groundwaters. Chem. Geol., 255, 173–181. doi:10.1016/j.chemgeo.2008.06.036
- Kawada T, Suzuki S 1998: A review on the cadmium content of rice, daily cadmium intake, and accumulation in the kidneys. J. Occup. Health, 40, 264–269. doi:10.1539/joh.40.264
- Khaokaew S, Chaney RL, Landrot G, Ginder-Vogel M, Sparks DL 2011a: Speciation and release kinetics of cadmium in an alkaline paddy soil under various flooding periods and draining conditions. Environ. Sci. Technol., 45, 4249–4255. doi:10.1021/es103971y
- Khaokaew S, Chaney RL, Landrot G, Ginder-Vogel M, Sparks DL 2011b: Speciation and release kinetics of cadmium in an alkaline paddy soil under various flooding periods and draining conditions. Environ. Sci. Technol., 45, 4249–4255. http://www.ncbi.nlm.nih.gov/pubmed/21513267.
- Kocar BD, Fendorf S 2009: Thermodynamic constraints on reductive reactions influencing the biogeochemistry of arsenic in soils and sediments. Environ. Sci. Technol., 43, 4871–4877. http://www.ncbi.nlm.nih.gov/pubmed/19673278 (December, 2015).
- Komárek M, Vaněk A, Ettler V 2013: Chemical stabilization of metals and arsenic in contaminated soils using oxides - A review. Environ. Pollut., 172, 9–22. http://www.ncbi.nlm.nih.gov/pubmed/22982549 (September, 2014).
- Kosolsaksakul P, Farmer JG, Oliver IW, Graham MC 2014: Geochemical associations and availability of cadmium (Cd) in a paddy field system, northwestern Thailand. Environ. Pollut., 187, 153–161. http://dx.doi.org/10.1016/j.envpol.2014.01.006.
- Kumpiene J, Ore S, Renella G, Mench M, Lagerkvist A, Maurice C 2006a: Assessment of zerovalent iron for stabilization of chromium, copper, and arsenic in soil. Environ. Pollut., 144, 62–69. http://www.sciencedirect.com/science/article/pii/S0269749106000376 (November, 2015).
- Kumpiene J, Ore S, Renella G, Mench M, Lagerkvist A, Maurice C 2006b: Assessment of zerovalent iron for stabilization of chromium, copper, and arsenic in soil. Environ. Pollut., 144, 62–69. http://www.ncbi.nlm.nih.gov/pubmed/16517035 (December, 2014).
- Li RY, Stroud JL, Ma JF, McGrath SP, Zhao FJ 2009: Mitigation of arsenic accumulation in rice with water management and silicon fertilization. Environ. Sci. Technol., 43, 3778–3783. http://pubs.acs.org/doi/abs/10.1021/es803643v.
- Lindsay WL 1979: Cadmium. In Chemical equilibria in soils:. Ed. Lindsay, WL, pp. 315–327. New York, Wiley-Interscience.
- Ma JF, Yamaji N, Mitani N, Xu X-Y, Su Y-H, McGrath SP, Zhao F-J 2008: Transporters of arsenite in rice and their role in arsenic accumulation in rice grain. Proc. Natl. Acad. Sci. U. S. A., 105, 9931–9935. http://www.pubmedcentral.nih.gov/articlerender.fcgi?artid=2481375&tool=pmcentrez&rendertype=abstract (March, 2016).
- MAFF 2014: Survey results of arsenic concentration in polished and unpolished rice grain in Japan. Press release of Ministry of Agriculture, Forestry and Fisheries http://www.maff.go.jp/j/press/syouan/nouan/pdf/140221–01.pdf (in Japanese).
- Makino T, Sugahara K, Sakurai Y, Takano H, Kamiya T, Sasaki K, Itou T, Sekiya N 2006: Remediation of cadmium contamination in paddy soils by washing with chemicals: selection of washing chemicals. Environ. Pollut., 144, 2–10. http://www.sciencedirect.com/science/article/pii/S0269749106000443 (October, 2015).
- Matsumoto S, Kasuga J, Taiki N, Makino T, Arao T 2015a: Inhibition of arsenic accumulation in Japanese rice by the application of iron and silicate materials. Catena, 135, 328–335. doi:10.1016/j.catena.2015.07.004
- Matsumoto S, Kasuga J, Taiki N, Makino T, Arao T 2015b: Reduction of the risk of arsenic accumulation in rice by the water management and material application in relation to phosphate status. J. Plant Interact., 10, 65–74. http://www.tandfonline.com/doi/full/10.1080/17429145.2015.1016129.
- Mohan D, Pittman CUJ 2007: Arsenic removal from water/wastewater using adsorbents–A critical review. J. Hazard. Mater., 142, 1–53. doi:10.1016/j.jhazmat.2007.01.006
- Nakamura K, Katou H 2012: Arsenic and Cadmium solubilization and immobilization in paddy soils in response to alternate submergence and drainage. In Competitive Sorption and Transport of Heavy Metals in Soils and Geological Media, Ed. Selim H, pp. 379–404. CRC Press, Boca Raton, FL.
- Nickson RT, Mcarthur JM, Ravenscroft P, Burgess WG, Ahmed KM 2000: Mechanism of arsenic release to groundwater, Bangladesh and West Bengal. Appl. Geochem., 15, 403–413. doi:10.1016/S0883-2927(99)00086-4
- Ning D, Song A, Fan F, Li Z, Liang Y 2014: Effects of slag-based silicon fertilizer on rice growth and brown-spot resistance. PLoS One, 9, e102681. http://www.pubmedcentral.nih.gov/articlerender.fcgi?artid=4103847&tool=pmcentrez&rendertype=abstract (March, 2016).
- Nonaka K, Takahashi K 1988: A method of measuring available silicates in paddy soils. JARQ, 22, 91–95.
- Oguri T, Yoshinaga J, Tao H, Nakazato T 2014: Inorganic arsenic in the Japanese diet: daily intake and source. Arch. Environ. Contam. Toxicol., 66, 100–112. doi:10.1007/s00244-013-9947-8
- Ohtsuka T, Yamaguchi N, Makino T, Sakurai K, Kimura K, Kudo K, Homma E, Dong DT, Amachi S 2013: Arsenic dissolution from Japanese paddy soil by a dissimilatory arsenate-reducing bacterium geobacter sp. OR-1. Environ. Sci. Technol., 47, 6263–6271.
- Ona-Nguema G, Morin G, Juillot F, Calas G, Brown GE 2005: EXAFS Analysis of Arsenite Adsorption onto Two-Line Ferrihydrite, Hematite, Goethite, and Lepidocrocite. Environ. Sci. Technol., 39, 9147–9155. http://pubs.acs.org/doi/abs/10.1021/es050889p.
- Ramaswami A, Tawachsupa S, Isleyen M 2001: Batch-mixed iron treatment of high arsenic waters. Water Res., 35, 4474–4479. doi:10.1016/S0043-1354(01)00168-3
- Sherman DM, Randall SR 2003: Surface complexation of arsenic(V) to iron(III) (hydr)oxides: structural mechanism from ab initio molecular geometries and EXAFS spectroscopy. Geochim. Cosmochim. Acta, 67, 4223–4230. http://linkinghub.elsevier.com/retrieve/pii/S0016703703002370 (December, 2014).
- Suda A, Baba K, Yamaguchi N, Akahane I, Makino T 2015: The effects of soil amendments on arsenic concentrations in soil solutions after long-term flooded incubation. Soil Sci. Plant Nutr., 1–11. http://www.tandfonline.com/doi/abs/10.1080/00380768.2015.1006119#.VNVxGUK5NX8.
- Sumner ME, Miller WP 1996: Cation exchange capacity and exchange coefficients. In Methods of Soil Analysis Part3. Chemical Methods, Ed. Sparks DL, pp. 1201–1229. Soil Science Society of America, Inc., Madison, WI.
- Sun H, Wang L, Zhang R, Sui J, Xu G 2006: Treatment of groundwater polluted by arsenic compounds by zero valent iron. J. Hazard. Mater., 129, 297–303. doi:10.1016/j.jhazmat.2005.08.026
- Truog E 1930: The determination of the readily available phosphorus in soils. J. Am. Soc. Agron., 22, 874–882. doi:10.2134/agronj1930.00021962002200100008x
- Tufano KJ, Fendorf S 2008: Confounding Impacts of Iron Reduction on Arsenic Retention. Environ. Sci. Technol., 42, 4777–4783. http://dx.doi.org/10.1021/es702625e (December, 2015).
- Waychunas GA, Rea BA, Fulller CC, Davis JA 1993: Surface chemistry of ferrihydrite Part 1. EXAFS studies of the geometry of coprecipitated and adsorbed arsenate. Geochim. Cosmochim. Acta, 57, 2251–2269. http://www.ncbi.nlm.nih.gov/pubmed/15003161.
- Weber F-A, Hofacker AF, Voegelin A, Kretzschmar R 2010: Temperature dependence and coupling of iron and arsenic reduction and release during flooding of a contaminated soil. Environ. Sci. Technol., 44, 116–122. http://www.ncbi.nlm.nih.gov/pubmed/20039741.
- Yamaguchi N, Nakamura T, Dong D, Takahashi Y, Amachi S, Makino T 2011: Arsenic release from flooded paddy soils is influenced by speciation, Eh, pH, and iron dissolution. Chemosphere, 83, 925–932. http://www.ncbi.nlm.nih.gov/pubmed/21420713 (October, 2014).