ABSTRACT
Excessive input of nitrogen fertilizer causes nitrogen pollution in aquatic environments. Utilizing microbial inoculants seems to be effective in decreasing the extent of nitrogen fertilizer application. Genome sequencing analysis of Azoarcus sp. strain KH32C, which was isolated from a paddy-upland rotation paddy field in Japan, showed that the strain may interact with plants. In this study, we examined the effect of inoculation of rice seeds with strain KH32C as a bacterial inoculant in paddy fields without nitrogen fertilization. Rice of three cultivars inoculated with strain KH32C were cultivated in paddy fields with different soil carbon and nitrogen levels. We investigated the growth rate of rice plants and the elemental composition of brown rice. In addition, the bacterial community structures in the rice root-associated soil were examined using molecular genetic analysis. KH32C inoculation resulted in an increase in the rice plant growth rate in the early growth phase with cultivar Nipponbare. Elemental composition analysis showed that the zinc concentration in brown rice of Nipponbare was increased by KH32C inoculation. KH32C inoculation affected the bacterial community in Nipponbare root-associated soil. The community of potential plant growth-promoting bacteria, the majority of which were in the class Alphaproteobacteria, had relatively high abundance in the early growth stage after KH32C inoculation. Our results indicated that strain KH32C can be utilized as a bacterial inoculant with the effect of promoting rice growth and enhancing the zinc content of rice grains in low nitrogen input paddy fields.
1. Introduction
In modernized agriculture, the excess application of nitrogen (N) fertilizer, which is used for increasing crop yields to supply food to the increasing human population, has caused N pollution such as a nitrate pollution of groundwater and eutrophication of surface water (Carpenter et al. Citation1998; Tilman et al. Citation2002; Vitousek et al. Citation1997). To prevent N pollution in aquatic environments, it is essential to decrease the extent of N fertilizer application (Carpenter et al. Citation1998); however, crop productivity will decrease without N fertilizer.
The production of N chemical fertilizer needs considerable energy consumption and releases massive amount of carbon dioxide (CO2) into the atmosphere as part of the industrial process. Increasing atmospheric CO2 concentration may affect the crop quality. Myers et al. (Citation2014) and Zhu et al. (Citation2018) reported that increasing atmospheric CO2 concentration lowered protein, iron (Fe), and zinc (Zn) concentrations of rice. Zhu et al. (Citation2018) also reported that mineral (Fe + Zn) deficits will become more serious in the highest rice-consuming countries with the lowest gross domestic product through changes of the rice grain quality.
Utilization of plant growth-promoting bacteria (PGPB) or rhizobacteria (PGPR) may be effective in overcoming the decrease in crop productivity as a result of limiting fertilizers and decrease the extent of N fertilizer use. One of the advantages of using microbial inoculants in agriculture is the reduction in environmental damage in comparison with chemical fertilizer use (Berg and Smalla Citation2009). PGPB or PGPR promote plant growth by various mechanisms (Berg and Smalla Citation2009; Lugtenberg and Kamilova Citation2009). Azoarcus sp. strain BH72, which was isolated from a root of kallar grass in Pakistan, has been reported as a nitrogen-fixing root endophyte of rice (Hurek and Reinhold-Hurek Citation2003). Recently, strain BH72 was classified into Azoarcus olearius whose type strain, DQS-4T, was isolated from oil-contaminated soil (Faoro et al. Citation2017). Bacillus pumilus strain TUAT-1, which was isolated from an agricultural field in Japan, was reported as a rice growth-promoting bacterium (Win et al. Citation2018). Denitrifying bacterium Azoarcus sp. strain KH32C, which strongly denitrifies extracellular nitrous oxide, was obtained from paddy-upland rotation paddy soil in Japan (Tago et al. Citation2011). Genome sequence analysis of strain KH32C revealed that gene clusters related to denitrification, nitrogen fixation, aromatic-compound degradation pathway, and capsular polysaccharide biosynthesis were found in the chromosome (Nishizawa et al. Citation2012), which indicated that strain KH32C interacts with rice plants. In the case of strain BH72, endoglucanase produced by strain BH72 is involved in the endophytic colonization of rice roots (Reinhold-Hurek et al. Citation2006). Meanwhile, strain KH32C does not have an endoglucanase gene (Nishizawa et al. Citation2012), and thus seems to have different features from strain BH72 in terms of its plant–microbe association.
Our objective in the present study was to elucidate the effect of the inoculation of rice with strain KH32C. Strain KH32C was inoculated onto rice seeds, and KH32C-inoculated rice was cultivated in paddy fields without any fertilizer. We investigated the effect of KH32C inoculation on rice growth, rice elemental composition, and the bacterial community in rice root-associated soil with molecular genetic analysis.
2. Materials and methods
2.1. Bacterium cultivation and inoculation of rice seeds for field experiments
Azoarcus sp. stain KH32C was cultivated on DNB-NS agar plates (0.08 g nutrient broth, 3.0 mM sodium nitrate, 4.4 mM sodium succinate, and 15 g agar per a liter; Tago et al. Citation2011) at 27–30°C under anaerobic conditions. Germinated rice seeds were immersed in a strain KH32C cell suspension diluted with water, which was adjusted the cell concentration to about 2.0 × 105 cells/mL using spectrophotometer (GeneQuant pro, Amersham Biosciences, Buckinghamshire, England), for 5–10 min. In a control experiment, bacterium inoculation was not done for germinated rice seeds. Three rice (Oryza sativa L.) cultivars, Nipponbare, Takanari, and IR64, were used in this experiment. Nipponbare is a japonica rice that was the first rice cultivar analyzed by genome sequencing (International Rice Genome Sequencing Project Citation2005). Takanari and IR64 are indica-type cultivars. Takanari is a high-yielding cultivar that was bred in Japan. IR64 was cultivated in tropical Asia, especially in Indonesia in the 1990s (Mackill and Khush Citation2018).
2.2. Rice cultivation in low nitrogen input paddy fields and sampling of rice plants and root-associated soil
Rice cultivation was performed at Center for International Field Agriculture Research and Education, College of Agriculture, Ibaraki University. Adjacent paddies, paddy field No. 4 (PF4) and paddy field No. 2 (PF2) (36°01ʹ N, 140°12ʹ E), were used as nitrogen-limited paddy fields in 2015 and 2017, respectively. The soil at the sites was Humic Allophane soil (Haludands, Haplic Andosols; Okada et al. Citation2011). Strain KH32C-inoculated and non-inoculated (control) rice seedlings were sown in different seedling trays, and the seedlings were raised in a greenhouse. PF4 had not been fertilized since the rice cultivation period in 2014. In PF4, rice transplantation was conducted on 29 May 2015 after raising seedlings for 20 days. In PF2, chemical fertilizer had been applied until the rice cultivation period in 2016 (4.5 g N m−2, 4.5 g phosphorus m−2, 4.5 g potassium m−2, 0.9 g magnesium m−2). Manure was applied as a soil conditioner before submergence of the field in 2017. Rice transplantation was conducted on 27 May 2017 after raising seedlings for 21 days. Transplanted rice seedlings of KH32C inoculation and control were cultivated in each paddy field without fertilizer. The plots for KH32C inoculation and control were adjacent, and each plot was surrounded by corrugated sheets. The planting density was 22.2 hills m−2 with one plant per hill. Five or six rice seedlings in each treatment were sampled from seedling trays when they were transplanted. Two hills were sampled at an early growth stage and at panicle formation stage. For investigation of rice yield, rice grains from a hill were sampled at the harvesting stage in PF4. In PF2, ear numbers from eight hills were investigated, and the grains from two hills which had intermediate ears numbers were sampled. For elemental composition analysis, rice grains from a hill were sampled at the harvesting stage. After digging up a rice plant, rice root-associated soil was sampled using a spatula from near the rice roots at early growth, panicle formation, full heading, and harvesting stages, except for the harvesting stage of PF4. Soil sampling at the full heading stage was conducted at only in PF2. Root-associated soil from two hills was mixed in a plastic bag. In the harvesting stage of PF4, soil samples were collected near rice plants at a depth of between 0 and 10 cm using sterile instruments. The soil samples were stored at 4°C and analyzed as soon as possible. Sampling dates were decided by the observation of the rice growth in each stage (Table S1). All samplings except for seedlings were performed in triplicate.
2.3. Investigation of rice plant growth
Rice plant samples were dried at 80°C, and dry weight (DW) was measured. Based on DW, the growth rate was calculated. The formula for growth rate was (sample DW per a rice plant – prior sample DW per a rice plant)/time between sampling days. Grain yield of rice was estimated using sampled grains, which were dried at 80°C.
2.4. Elemental composition analysis of rice
Harvested rice grains were hulled and milled. Milled rice was dried at 80°C overnight, and the total carbon (TC) and total N (TN) contents were analyzed using CN Corder (JM3000CN, J Science Lab, Kyoto, Japan). Milled rice samples were dried at 105°C for 12 h to measure DW. Next, for ashing of the milled rice, 1.0–1.5 g samples were heated 105°C for 24 h. Then, the temperature was increased at a rate of 50°C/hour. The temperature increase was stopped at 350°C and maintained for 5 h. Then, the temperature increase was resumed and finally reached 550°C, which was maintained for 10 h (MMF-1; As One, Osaka, Japan). Semi-quantitative analysis of inorganic elements was performed using the ashed-sample sealed in a powder sample container (Micro C-cell) by X-ray fluorescence in vacuum conditions using an energy dispersive X-ray fluorescence spectrometer (EDX-700HS; Shimazu, Kyoto, Japan). Lower energy below 4.4 keV and higher energy above 4.4 keV were measured at applied voltages of 15 kV or 50 kV, respectively, and an integration time of 100 sec. The X-ray beam was collimated in 0.3 mm. Semi-quantitative analysis was done using the fundamental parameter method supplied with the instrumental program. Semi-quantitative values were calculated by multiplying the peak intensity of each element (kV×cps/µA), with sensitivity coefficients set in advance, and adjusted summation of all identified elemental values to be 100%. The composition of each element in dried rice was calculated.
2.5. Soil total carbon and nitrogen
After the soil samples were dried at 105°C overnight, TC and TN were analyzed using CN Corder (JM3000CN, J Science Lab).
2.6. DNA extraction from soil samples
DNA from root-associated soil samples was extracted using ISOIL for Beads Beating (Nippon Gene, Toyama, Japan) using a standard protocol with some modifications (Nishizawa et al. Citation2008). DNA samples extracted from triplicate root-associated soil were pooled as one sample.
2.7. Terminal-restriction fragment length polymorphism (T-RFLP) profiling
PCR amplification of the bacterial 16S rRNA gene was performed. The total volume of the PCR mixture was 30 µL, which contained 1 µL of template DNA, 0.5 µL of 10 µM forward primer (Q-10F, 5´-CAGTTTGATCCTGGCTCAG-3´; J-Bio21, Kisarazu, Japan), 0.5 µL of 10 µM reverse primer (926r, 5´-CCGTCAATTCCTTTRAGTTT-3´) (Sato et al. Citation2009), 3 µL of 10× Ex Taq buffer (20 mM Mg2+ plus), 3 µL of dNTP Mixture (2.5 mM each), and TaKaRa Ex Taq polymerase (Takara Bio, Otsu, Japan). The Q-10F primer labeled with a quenching fluorescence was purchased from J-Bio21. PCR conditions were initially 95°C (2 min), then cycles of 95°C (30 sec), 54°C (45 sec), and 72°C (1.5 min). In the case of samples from PF4, the PCR cycle number was 25 cycles. In samples from PF2, the cycle number for each sample was decided using real-time quantitative-PCR in order to reflect the bacterial community composition (Nishizawa et al. Citation2008). PCR reactions were carried out in a TaKaRa PCR Thermal Cycler Dice® Touch (Takara Bio). The PCR products were purified using a QIAquick PCR Purification Kit (Qiagen, Hilden, Germany) in accordance with the manufacturer’s protocol. HaeIII, HhaI, and MspI (Takara Bio) were used for restriction enzyme digestion, and the fragments were purified using a QIAquick PCR Purification Kit (Qiagen). Two µL of terminal-restriction fragments (T-RFs) was mixed with 12 µL of Hi-DiTM Formamide Genetic-analysis grade (Applied Biosystems, Foster City, USA) and 0.1 µL of GeneScanTM 500 Liz® Size Standard. T-RFs were denatured at 96°C for 2 min and quenched thereafter. The length of T-RFs was determined using an ABI PRISM 3130xl Genetic Analyzer (Applied Biosystems) in GeneMapper mode. Analysis for the determination of T-RF lengths was performed by using GeneMapper® Software version 3.7 (Applied Biosystems). T-RFs data were developed by using T-REX software (Culman et al. Citation2009). T-RFs whose sizes were under 20 bases were omitted from this analysis. Based on the T-RFs profile, distance matrix of the bacterial communities was calculated using Jaccard’s index, and cluster analysis was performed with Ward’s method using the vegan package of R program (ver. 3.2.5, R Development Core Team Citation2008).
2.8. Meta-amplicon sequencing analysis
To assess the bacterial community composition of root-associated soil with Nipponbare, Miseq Illumina sequencing of the V3-V4 region of bacterial 16S rRNA was carried out. For PCR amplification, the primer set of S-D-Bact-0341-b-S-17 (5´-CCTACGGGNGGCWGCAG-3´) and S-D-Bact-0785-a-A-21 (5´-GACTACHVGGGTATCTAATCC-3´) which added overhang adopter sequences were used (Klindworth et al. Citation2013). PCR conditions were initially 95°C (3 min), then 25 cycles of 95°C (30 sec), 55°C (30 sec), and 72°C (30 sec), and finally 72°C (5 min). A Miseq Reagent Kit (Illumina, San Diego, CA, USA) was used for sequencing. Nucleotide sequence data were optimized using mothur (ver. 1.36.1, Schloss et al. Citation2009) in accordance with the Miseq standard operating procedure (Kozich et al. Citation2013). Briefly, paired-end sequences were assembled, then the allowed quality score of a mismatched base in the overlap was less than 10. Alignment using the align.seqs command was done using SILVA-based bacterial reference alignment. Filtering by read length and long homopolymer removing denoise were done using the screen.seqs command, and chimera removal was done using the chimera.unchim command. Taxonomy classification was done using the classify.seqs command with mothur-modified RDP reference file ver. 14 (trainset14_032015, Cole et al. Citation2009), and non-bacteria sequences were removed using the remove.liniage command. Optimized sequencing data were classified as operational taxonomic units (OTUs) at 97% similarity using the cluster.split command. All further analyses were conducted based on the OTU. Calculation of alpha-diversity and the rarefaction curve, non-metric multidimensional scaling (NMDS) analysis, and estimation of the bacterial taxa were conducted using mothur. Heatmap analysis of bacterial community dynamics based on Z-score was analyzed using the gplots package of R program (R Development Core Team Citation2008). Representative sequences of each OTU were extracted using the get.oturep command in mothur; later, relative abundance of potential plant growth-promoting bacteria (PGPB) was estimated by comparing representative sequence of each OTU with the PGPB database (Chao et al. Citation2016) using BLAST+ (ver. 2.4.0). Each OTU sequence was considered as a potential PGPB when the E-value was ≤1 × 10−60, sequence identity was ≥95%, and query coverage was ≥99% (Chao et al. Citation2016).
2.9. Statistical analysis
To assess the effect of strain KH32C, paired t-tests were carried out between KH32C inoculation and control for rice growth rate, rice yield, and elemental composition of brown rice.
2.10. Accession number
Raw sequence data obtained in this study have been submitted to DDBJ under accession number DRA007632.
3. Results
3.1. Total carbon and nitrogen of paddy field soil
No remarkable effects on TC and TN were observed after KH32C inoculation in rice root-associated soil. TC in PF4 was lower than PF2; the ranges of TC in PF4 and PF2 were 51.44–62.38 g/kg and 65.35–68.17 g/kg, respectively. TN showed a similar trend to TC; TN ranges of PF4 and PF2 were 4.54–5.63 g/kg and 5.25–5.67 g/kg, respectively ().
Table 1. Total carbon and total nitrogen in paddy field soil. Eg, early growth stage; Pf, panicle formation stage; Fh, full heading stage; Hv, harvesting stage
3.2. Rice plant growth
Rice cultivation on N-deficient agar plates was performed before the field experiment (Text S1). Inoculation of Nipponbare seeds with strain KH32C significantly promoted the growth of plant biomass on N-deficient agar plates; the fresh weight of rice seedlings with KH32C inoculation after 12 days’ cultivation was 1.6 times that after inoculation with Escherichia coli (Figure S1). Strain KH32C was detected by PCR from the roots of KH32C-inoculated rice (Figure S2). We also performed experiments using pots in low-N conditions. KH32C inoculation promoted the growth of Nipponbare; the plant biomass at heading stage was 1.1 times higher that after E. coli inoculation (data not shown). Thus, we performed field experiments.
shows rice plant growth on two paddy fields. Strain KH32C was inoculated onto rice seeds before raising seedling. In the case of Nipponbare, the growth rate increased significantly after KH32C inoculation compared with the control, corresponding to a 1.9-fold increase in the early growth stage and 1.7-fold in the panicle formation stage in PF4. In PF2, the growth rate in the early growth stage was increased 1.3-fold after KH32C inoculation, but the increase was not significant. In the case of Takanari, the growth rate in the early growth stage was decreased after KH32C inoculation, 0.8 times compared with the control in PF4; however, in PF2, the growth rate in both the early growth stage and panicle formation stage was similar with KH32C inoculation and the control. Inoculation with strain KH32C did not show any remarkable effect on the growth of IR64 in PF4, whereas a negative effect on growth at the panicle formation stage was observed in PF2. KH32C inoculation had no significant effects on rice yields (Table S2).
3.3. Elemental composition of brown rice
Elemental composition of brown rice is shown in . Although most elements were not different between the KH32C inoculation and control samples, N levels in Nipponbare, Takanari, and IR64 harvested from PF2 were lower than those from PF4. The contents of silicon, Zn, Fe, and copper (Cu) in Nipponbare, Takanari, and IR64 were different between PF4 and PF2. In PF4, calcium in IR64, Zn of Nipponbare and Takanari, manganese in IR64 were significantly increased with KH32C inoculation, but potassium (K) and rubidium (Rb) levels in Takanari were decreased. In PF2, KH32C inoculation increased the contents of Fe in Takanari and Cu and Rb in Nipponbare, whereas N in Takanari and K in IR64 decreased. The contents of Zn and Cu in Nipponbare tended to increase after KH32C inoculation in both PF4 and PF2. KH32C inoculation resulted in a significant increase in the Zn content of Nipponbare (32.7%) and Takanari (27.5%) in PF4, 12.4% increase in Nipponbare in PF2. Meanwhile, the Cu content of Nipponbare was significantly increased by 10.0% after KH32C inoculation in PF2, and the Cu content of Nipponbare increased 13.6% in PF4 although the increase was not statistically significant. No elements that were increased or decreased significantly by KH32C inoculation were in common in PF4 and PF2 among all three cultivars.
Table 2. Elemental composition in dried brown rice
3.4. Soil bacterial community analysis
The bacterial community in rice root-associated soil was assessed by T-RFLP profiling. In PF4, cluster analysis was carried out based on the T-RFs profile, indicating that the bacterial community structures were similar within each rice growth stage. Of note, in the early growth stage, the bacterial community structures of all cultivars inoculated with strain KH32C were in the same cluster (). In PF2, the bacterial community structures of three cultivars in the early growth stage were not similar within KH32C inoculation (data not shown), but bacterial communities in KH32C-inoculated Nipponbare tended to be similar throughout the cultivation period (Figure S3a). In the case of Takanari and IR64, the bacterial communities were not similar according to KH32C inoculation but tended to be similar within each rice growth stage (Figure S3b and S3c).
Figure 2. Cluster analysis of the bacterial community in rice root-associated soil based on terminal restriction fragments profile in PF4. Eg, early growth stage; Pf, panicle formation stage; Hv, harvesting stage. Underlines indicate KH32C inoculation
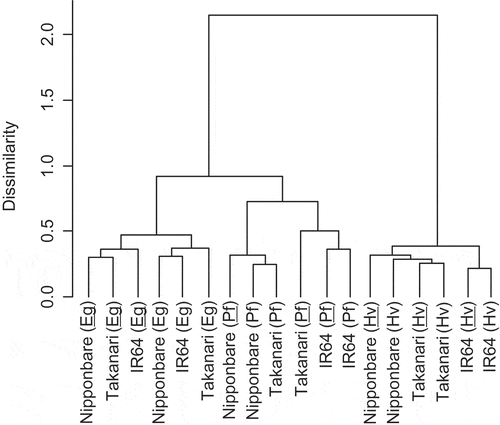
Figure 3. Heatmap analysis based on the relative abundance of Alphaproteobacteria and Deltaproteobacteria at the family level in root-associated soil of Nipponbare plants. Color means Z-score. Eg, early growth stage; Pf, panicle formation stage; Hv, harvesting stage
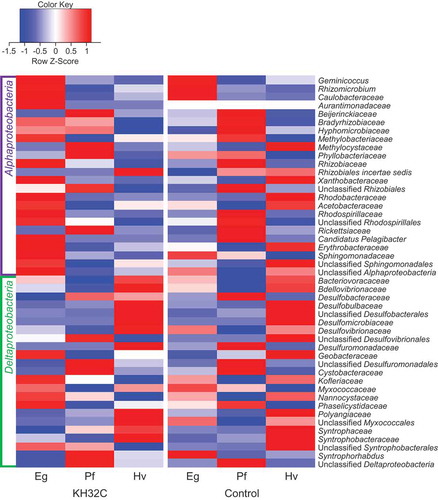
Figure 4. Relative abundance of potential plant growth-promoting bacteria at the phylum level in root-associated soil of Nipponbare in PF4. Proteobacteria is shown at a class level. Eg, early growth stage; Pf, panicle formation stage; Hv, harvesting stage; ACC deaminase, 1-aminocyclopropane-1-carboxic acid deaminase; IAA, indole-3-acetic acid; PGP, plant growth-promoting
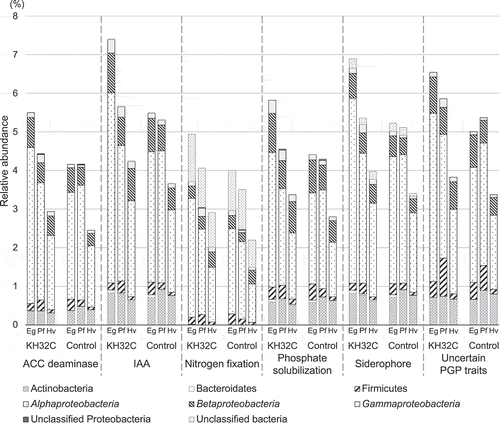
To reveal the bacterial community composition, meta-amplicon sequencing analysis was carried out using Nipponbare root-associated soil of PF4. In meta-amplicon sequencing analysis, 91,511 total high-quality sequences and 14,064 total OTUs were obtained (Table S3). The diversity of the bacterial community depended on the rice cultivation period as well (Figure S4). The Shannon index of KH32C inoculation samples changed from 7.42, 7.36 to 7.62, the Shannon index of control samples changed from 7.48, 7.32 to 7.67 in the stages of early growth, panicle formation, and harvesting, respectively (Table S4). NMDS analysis showed that the bacterial community was affected by both rice growth stage and KH32C inoculation (Figure S5). The dominant phyla were Proteobacteria and Acidobacteria (Figure S6). Class Betaproteobacteria accounted for 9.7% to 11.4% of the whole bacterial amplicon-sequences in each sample. Among Betaproteobacteria, the order Rhodocyclales was 0.27% to 0.67% of the whole bacterial amplicon-sequences. Azoarcus, which belong to Rhodocyclales, was detected in only one amplicon-sequence (0.003%) with KH32C inoculation at the harvesting stage. The bacterial community structure of the class Alphaproteobacteria was different between the KH32C inoculation and the control. Most of the detected families in Alphaproteobacteria had relatively high abundance in the early growth stage with KH32C inoculation; however, in the control, families of Alphaproteobacteria were abundant in the panicle formation stage. On the other hand, Deltaproteobacteria showed similar patterns between KH32C inoculation and the control (). The PGPB database constructed by Chao et al. (Citation2016) contains 16S rRNA genes of PGPB with six plant growth promoting (PGP) properties, nitrogen fixation, phosphate solubilization, production of 1-aminocyclopropane-1-carboxic acid deaminase, indole-3-acetic acid, or siderophores, and uncertain PGP traits, according to 1,322 previous papers related to PGPB. In the present study, relative abundances of PGPB of all six PGP traits were found in high proportions in KH32C inoculation, particularly in the early growth stage. In all PGP traits, the majority was class Alphaproteobacteria ().
4. Discussion
In this study, we examined the effect of KH32C inoculation onto rice seeds in paddy fields without N fertilizer. KH32C inoculation promoted early growth of Nipponbare. In the case of Takanari and IR64, strain KH32C had a negative effect on plant growth (). It was suggested that the growth promotion effect depended on differences among the rice cultivars. The kind of plant species is an important factor in plant–microbe interactions, and plant cultivars are also important. Chanway, Nelson, and Holl (Citation1988) reported that the growth-promoting effect of Bacillus spp. with spring wheat was different among cultivars. In addition, nodulation by some rhizobia strains was cultivar specific (Heron and Pueppke Citation1984). Differences among cultivars also affect N use. N absorption or N use efficiency differs among cultivars (Broadbent, De Datta, and Laureles Citation1987; Fageria and Baligar Citation2003; Le Gouis et al. Citation2000; van Sanford and MacKown Citation1986). In addition, N use efficiency in rice and wheat is decreased with an increasing extent of N fertilizer application (Fageria and Baligar Citation2001; Gauer et al. Citation1992). The growth-promoting effect of strain KH32C in Nipponbare was exerted especially in PF4. Soil TC and TN levels were lower in PF4 than in PF2 (). These results suggested that KH32C inoculation onto rice seeds was highly effective for promoting rice growth in lower carbon (C) and N soils.
KH32C inoculation increased the Zn content in Nipponbare brown rice in both PF4 and PF2, although not to a significant extent in PF2 (). An increase in atmospheric CO2 concentration decreased the Fe and Zn contents in rice (Myers et al. Citation2014; Zhu et al. Citation2018). Zn deficiency is a serious problem in human health, and the estimated prevalence of Zn deficiency is 31% around the world (Caulfield and Black Citation2004). Zn deficiency is more severe in developing countries (WHO Citation2002) because people depend on cereals whose Zn content is low (Gibson Citation1994; Shahzad, Rouached, and Rakha Citation2014). Biofortification of rice may be effective for alleviating Zn malnutrition, and considerable effort to increase the Zn concentration in rice had been made (Shahzad, Rouached, and Rakha Citation2014; Swamy et al. Citation2016). For example, Masuda et al. (Citation2009) used a transgenic technique, and consequently, Zn concentration in polished rice and brown rice increased. Suitable N fertilizer could promote Zn accumulation in brown rice (Hao et al. Citation2007), indicating that N fertilizer as well as Zn fertilizer participate in the Zn content in rice. As with the growth-promoting effect, the Zn-enhancing effect with strain KH32C was strongly exerted in PF4 soil. Strain KH32C is expected to be utilized as bacterial inoculant to enhance the Zn content of rice grain in low-N input paddy field.
In soil including the rhizosphere, the bacterial community is affected by various factors, such as plant species and cultivars (Berg and Smalla Citation2009; Philippot et al. Citation2013; van Overbeek and van Elsas Citation2008), plant growth stage (Philippot et al. Citation2013; van Overbeek and van Elsas Citation2008), and fertilizer (Fierer et al. Citation2012; Wessén, Hallin, and Philippot Citation2010). In the present study, the bacterial communities of rice root-associated soil were affected by the rice growth stage ( and S3). KH32C inoculation affected the bacterial community of rice root-associated soil in the early growth stage in PF4 (). In the case of Nipponbare in PF2, the effect of KH32C inoculation on the bacterial community of root-associated soil was stronger than the effect of rice growth stage (Figure S3a), although the bacterial community with Takanari and IR64 were mainly affected by the rice growth stage (Figure S3b and S3c). Meta-amplicon sequencing analysis also showed that KH32C inoculation affected the bacterial community of Nipponbare root-associated soil ( and , and S5). To our knowledge, this is the first study to reveal that inoculation of the betaproteobacteria Azoarcus strongly affected the bacterial community of Nipponbare in the early growth stage. KH32C inoculation promoted the growth of Nipponbare alone (), implying that the effect on the bacterial community structure depended on whether strain KH32C interacted with plant or not. Quality and quantity of rhizodeposits both affected the soil bacterial community. For example, flavonoids included in root exudates induce symbiosis with rhizobia and mycorrhizal fungi, whereas another flavonoids inhibit root pathogenic bacteria and fungi (Hassan and Mathesius Citation2012). KH32C inoculation promoted rice plant growth, and perhaps affected the quality or quantity of rhizodeposits at the same time directly or indirectly. Change in the component of rhizodeposits may cause changes in the microbial community in Nipponbare root-associated soil. In the case of Nipponbare, the relative abundance of Alphaproteobacteria was increased at the family level by KH32C inoculation ( and ), which consequently resulted in an increase in potential PGPB ().
The mechanism of the plant growth-promoting effect by strain KH32C is still unknown. The complete genome sequence of strain KH32C suggested that strain KH32C does not have an endoglucanase gene (Nishizawa et al. Citation2012). Thus, strain KH32C seems to be unable to penetrate into the interior of rice root cells, which means strain KH32C is not an endophyte like strain BH72. Moreover, a gene related to the indole-3-acetic acid synthesis pathway was not found in the KH32C genome (Nishizawa et al. Citation2012). Therefore, strain KH32C maybe have a distinctive mechanism to promote plant growth.
Gao et al. (Citation2017) reported that inoculation of strain KH32C in soil could mitigate the emission of nitrous oxide, which is a greenhouse gas, from agricultural soil, suggesting that strain KH32C can be utilized as a bacterial inoculant to mitigate the greenhouse gas emission. In this study, we revealed that strain KH32C promoted the early growth of rice and enhanced Zn content in brown rice, which were cultivar (Nipponbare) specific, in low-N input paddy fields. Our results indicated that strain KH32C can be utilized as a biofertilizer to regulate rice growth and rice grain quality. Moreover, KH32C inoculation onto rice seeds affected the bacterial community in root-associated soil, which possibly participates in the growth promotion effect of strain KH32C. Further study will be needed to elucidate the mechanism of the growth-promoting effect of strain KH32C. Our results in this study will contribute to improvements in the environment, food, and human health.
Correction Statement
This article has been republished with minor changes. These changes do not impact the academic content of the article.
sup_Sakoda_revision_final.docx
Download MS Word (417.7 KB)Acknowledgments
We thank Drs. Shimpei Uraguchi and Tsuneo Hakoyama for help with laboratory experiments. We also thank Dr. Takahiro Takimoto and the technical staff of Center for International Field Agriculture Research and Education, College of Agriculture, Ibaraki University for their assistance with the field experiments. This work was partially supported by Social Implementation Program on Climate Change Adaptation Technology (SI-CAT) from the Ministry of Education, Culture, Sports, Science and Technology and also by a grant of Takano Life Science Research Foundation.
Disclosure statement
No potential conflict of interest was reported by the authors.
Supplemental Material
Supplemental data for this article can be accessed here.
References
- Berg, G., and K. Smalla. 2009. “Plant Species and Soil Type Cooperatively Shape the Structure and Function of Microbial Communities in the Rhizosphere.” FEMS Microbiology Ecology 68: 1–13. doi:10.1111/j.1574-6941.2009.00654.x.
- Broadbent, F. E., S. K. De Datta, and E. V. Laureles. 1987. “Measurement of Nitrogen Utilization Efficiency in Rice Genotypes.” Agronomy Journal 79: 786–791. doi:10.2134/agronj1987.00021962007900050006x.
- Carpenter, S. R., N. F. Caraco, D. L. Correll, R. W. Howarth, A. N. Sharpley, and V. H. Smith. 1998. “Nonpoint Pollution of Surface Waters with Phosphorus and Nitrogen.” Ecological Applications 8: 559–568. doi:10.1890/1051-0761(1998)008[0559:NPOSWW]2.0.CO;2.
- Caulfield, L. E., and R. E. Black. 2004. “Zinc Deficiency.” Chap. 5 in Comparative Quantification of Health Risks: Global and Regional Burden of Disease Attribution to Selected Major Risk Factors, edited by M. Ezzati, A. D. Lopez, A. Rodgers, and C. J. L. Murray. Geneva, Switzerland: World Health Organization. http://www.who.int/healthinfo/global_burden_disease/cra/en/
- Chanway, C. P., L. M. Nelson, and F. B. Holl. 1988. “Cultivar-Specific Growth Promotion of Spring Wheat (Triticum aestivum L.) by Coexistent Bacillus Species.” Canadian Journal of Microbiology 34: 925–929. doi:10.1139/m88-164.
- Chao, Y., W. Liu, Y. Chen, W. Chen, L. Zhao, Q. Ding, S. Wang, Y.-T. Tang, T. Zhang, and R.-L. Qiu. 2016. “Structure, Variation, and Co-occurrence of Soil Microbial Communities in Abandoned Sites of a Rare Earth Elements Mine.” Environmental Science & Technology 50: 11481–11490. doi:10.1021/acs.est.6b02284.
- Cole, J. R., Q. Wang, E. Cardenas, J. Fish, B. Chai, R. J. Farris, A. S. Kulam-Syed-Mohideen, et al. 2009. “The Ribosomal Database Project: Improved Alignment and New Tools for rRNA Analysis.” Nucleic Acids Research 37 :D141–145. doi:10.1093/nar/gkn879.
- Culman, S. W., R. Bukowski, H. G. Gauch, H. Cadillo-Quiroz, and D. H. Buckley. 2009. “T-REX: Software for the Processing and Analysis of T-RFLP Data.” BMC Bioinformatics 10. doi:10.1186/1471-2105-10-171.
- Fageria, N. K., and V. C. Baligar. 2001. “Lowland Rice Response to Nitrogen Fertilization.” Communications in Soil Science and Plant Analysis 32: 1405–1429. doi:10.1081/CSS-100104202.
- Fageria, N. K., and V. C. Baligar. 2003. “Methodology for Evaluation of Lowland Rice Genotypes for Nitrogen Use Efficiency.” Journal of Plant Nutrition 26: 1315–1333. doi:10.1081/PLN-120020373.
- Faoro, H., R. Rene Menegazzo, F. Battistoni, P. Gyaneshwar, F. P. Do Amaral, C. Taulé, S. Rausch, et al. 2017. “The Oil-contaminated Soil Diazotroph Azoarcus olearius DQS-4T is Genetically and Phenotypically Similar to the Model Grass Endophyte Azoarcus sp. BH72.” Environmental Microbiology Reports 9: 223–238. doi:10.1111/1758-2229.12502.
- Fierer, N., C. L. Lauber, K. S. Ramirez, J. Zaneveld, M. A. Bradford, and R. Knight. 2012. “Comparative Metagenomic, Phylogenetic and Physiological Analysis of Soil Microbial Communities across Nitrogen Gradients.” ISME Journal 6: 1007–1017. doi:10.1038/ismej.2011.159.
- Gao, N., W. Shen, E. Camargo, Y. Shiratori, T. Nishizawa, K. Isobe, X. He, and K. Senoo. 2017. “Nitrous Oxide (N2O)-reducing Denitrifier-inoculated Organic Fertilizer Mitigates N2O Emissions from Agricultural Soils.” Biology and Fertility of Soils 53: 885–898. doi:10.1007/s00374-017-1231-z.
- Gauer, L. E., C. A. Grant, D. T. Gehl, and L. D. Bailey. 1992. “Effects of Nitrogen Fertilization on Grain Protein Content, Nitrogen Uptake, and Nitrogen Use Efficiency of Six Spring Wheat (Triticum aestivum L.) Cultivars, in Relation to Estimated Moisture Supply.” Canadian Journal of Plant Science 72: 235–241. doi:10.4141/cjps92-026.
- Gibson, R. S. 1994. “Zinc Nutrition in Developing Countries.” Nutrition Research Reviews 7: 151–173. doi:10.1079/NRR19940010.
- Hao, H.-L., Y.-Z. Wei, X.-E. Yang, Y. Feng, and C.-Y. Wu. 2007. “Effects of Different Nitrogen Fertilizer Levels on Fe, Mn, Cu and Zn Concentrations in Shoot and Grain Quality in Rice (Oryza sativa).” Rice Science 14: 289–294. doi:10.1016/S1672-6308(08)60007-4.
- Hassan, S., and U. Mathesius. 2012. “The Role of Flavonoids in Root-Rhizosphere Signalling: Opportunities and Challenges for Improving Plant-microbe Interactions.” Journal of Experimental Botany 63: 3429–3444. doi:10.1093/jxb/err430.
- Heron, D. S., and S. G. Pueppke. 1984. “Mode of Infection, Modulation Specificity, and Indigenous Plasmids of 11 Fast-growing Rhizobium Japonicum Strains.” Journal of Bacteriology 160: 1061–1066.
- Hurek, T., and B. Reinhold-Hurek. 2003. “Azoarcus sp. Strain BH72 as a Model for Nitrogen-fixing Grass Endophytes.” Journal of Biotechnology 106: 169–178. doi:10.1016/j.jbiotec.2003.07.010.
- International Rice Genome Sequencing Project. 2005. “The Map-based Sequence of the Rice Genome.” Nature 436: 793–800. doi:10.1038/nature03895.
- Klindworth, A., E. Pruesse, T. Schweer, J. Peplies, C. Quast, M. Horn, and F. O. Glöckner. 2013. “Eavluation of General 16S Ribosomal RNA Gene PCR Primers for Classical and Next-generation Sequencing-based Diversity Studies.” Nucleic Acids Research 41: e1. doi:10.1093/nar/gks808.
- Kozich, J. J., S. L. Westcott, N. T. Baxter, S. K. Highlander, and P. D. Schloss. 2013. “Development of a Dual-index Sequencing Strategy and Curation Pipeline for Analyzing Amplicon Sequence Data on the Miseq Illumina Sequencing Platform.” Applied Environmental Microbiology, 79: 5112–5120. doi:10.1128/AEM.01043-13.
- Le Gouis, J., D. Béghin, E. Heumez, and P. Pluchard. 2000. “Genetic Differences for Nitrogen Uptake and Nitrogen Utilisation Efficiencies in Winter Wheat.” European Journal of Agronomy 12: 163–173. doi:10.1016/S1161-0301(00)00045-9.
- Lugtenberg, B., and F. Kamilova. 2009. “Plant-Growth-Promoting Rhizobacteria.” Annual Review of Microbiology 63: 541–556. doi:10.1146/annurev.micro.62.081307.162918.
- Mackill, D. J., and G. S. Khush. 2018. “IR64: A High-quality and High-yielding Mega Variety.” Rice 11: 18. doi:10.1186/s12284-018-0208-3.
- Masuda, H., K. Usuda, T. Kobayashi, Y. Ishimaru, Y. Kakei, M. Takahashi, K. Higuchi, H. Nakanishi, S. Mori, and N. K. Nishizawa. 2009. “Overexpression of the Barley Nicotianamine Synthase Gene HvNAS1 Increases Iron and Zinc Concentrations in Rice Grains.” Rice 2: 155–166. doi:10.1007/s12284-009-9031-1.
- Myers, S. S., A. Zanobetti, I. Kloog, P. Huybers, A. D. B. Leakey, A. J. Bloom, E. Carlisle, et al. 2014. “Increasing CO2 Threatens Human Nutrition.” Nature 510: 139–142. doi:10.1038/nature13179.
- Nishizawa, T., K. Tago, K. Oshima, M. Hattori, S. Ishii, S. Otsuka, and K. Senoo. 2012. “Complete Genome Sequence of the Denitrifying and N2O-reducing Bacterium Azoarcus sp. Strain KH32C.” Journal of Bacteriology 194: 1255. doi:10.1128/JB.06618-11.
- Nishizawa, T., M. Komatsuzaki, N. Kaneko, and H. Ohta. 2008. “Archaeal Diversity of Upland Rice Field Soils Assessed by the Terminal Restriction Fragment Length Polymorphism Method Combined with Real Time Quantitative-PCR and a Clone Library Analysis.” Microbes and Environments 23: 237–243. doi:10.1264/jsme2.23.237.
- Okada, H., S. Niwa, S. Takemoto, M. Komatsuzaki, and M. Hiroki. 2011. “How Different or Similar are Nematode Communities between a Paddy and an Upland Rice Fields across a Flodding–Drainage Cycles?” Soil Biology & Biochemistry 43: 2142–2151. doi:10.1016/j.soilbio.2011.06.018.
- Philippot, L., J. M. Raaijmakers, P. Lemanceau, and W. H. van der Putten. 2013. “Going Back to the Roots: The Microbial Ecology of the Rhizosphere.” Nature Reviews Microbiology 11: 789–799. doi:10.1038/nrmicro3109.
- R Development Core Team 2008: “R: A Language and Environment for Statistical Computing”. R Foundation for Statistical Computing, Vienna, Austria. ISBN 3-900051-07-0. http://www.R-project.org
- Reinhold-Hurek, B., T. Maes, S. Gemmer, M. van Montagu, and T. Hurek. 2006. “An Endoglucanase is Involved in Infection of Rice Roots by the Not-cellulose-metabolizing Endophyte Azoarcus sp. Strain BH72.” Molecular Plant-microbe Interactions : MPMI 19: 181–188. doi:10.1094/MPMI-19-0181.
- Sato, Y., K. Hosokawa, R. Fujimura, T. Nishizawa, T. Kamijo, and H. Ohta. 2009. “Nitrogenase Activity (Acetylene Reduction) of an Iron-oxidizing Leptospirillum Strain Cultured as a Pioneer Microbe from a Recent Volcanic Deposit on Miyake-jima, Japan.” Microbes and Environments 24: 291–296. doi:10.1264/jsme2.ME09139.
- Schloss, P. D., S. L. Westcott, T. Ryabin, J. R. Hall, M. Hartmann, E. B. Hollister, R. A. Lesniewski et al. 2009. “Introducing Mothur: Open-source, Platform-independent, Community-supported Software for Describing and Comparing Microbial Communities.” Applied Environmental Microbiology 75:7537–7541. doi:10.1128/AEM.01541-09.
- Shahzad, Z., H. Rouached, and A. Rakha. 2014. “Combating Mineral Malnutrition through Iron and Zinc Biofortification of Cereals.” Comprehensive Reviews in Food Science and Food Safety 13: 329–346. doi:10.1111/1541-4337.12063.
- Swamy, B. P. M., M. A. Rahman, M. A. Inabangan-Asilo, A. Amparado, C. Manito, P. Chadha-Mohanty, R. Reinke, and I. H. Slamet-Loedin. 2016. “Advances in Breeding for High Grain Zinc in Rice.” Rice 9. doi:10.1186/s12284-016-0122-5.
- Tago, K., S. Ishii, T. Nishizawa, S. Otsuka, and K. Senoo. 2011. “Phylogenetic and Functional Diversity of Denitrifying Bacteria Isolated from Various Rice Paddy and Rice-soybean Rotation Fields.” Microbes and Environments 26: 30–35. doi:10.1264/jsme2.ME10167.
- Tilman, D., K. G. Cassman, P. A. Matson, R. Naylor, and S. Polasky. 2002. “Agricultural Sustainability and Intensive Production Practices.” Nature 418: 671–677. doi:10.1038/nature01014.
- van Overbeek, L., and J. D. van Elsas. 2008. “Effects of Plant Genotype and Growth Stage on the Structure of Bacterial Communities Associated with Potato (Solanum tuberosum L.).” FEMS Microbiology Ecology 64: 283–296. doi:10.1111/j.1574-6941.2008.00469.x.
- van Sanford, D. A., and C. T. MacKown. 1986. “Variation in Nitrogen Use Efficiency among Soft Red Winter Wheat Genotypes.” Theoretical and Applied Genetics 72: 158–163. doi:10.1007/BF00266987.
- Vitousek, P. M., H. A. Mooney, J. Lubchenco, and J. M. Melillo. 1997. “Human Domination of Earth’s Ecosystems.” Science 277: 494–499. doi:10.1126/science.277.5325.494.
- Wessén, E., S. Hallin, and L. Philippot. 2010. “Differential Responses of Bacterial and Archaeal Groups at High Taxonomical Ranks to Soil Management.” Soil Biology and Biochemistry 42: 1759–1765. doi:10.1016/j.soilbio.2010.06.013.
- Win, K. T., A. Z. Oo, N. Ohkama-Ohtsu, and T. Yokoyama. 2018. “Bacillus pumilus Strain TUAT-1 and Nitrogen Application in Nursey Phase Promote Growth of Rice Plants under Field Conditions.” Agronomy 8: 216. doi:10.3390/agronomy8100216.
- World Health Organization. 2002. The World Health Report 2002 - Reducing Risks, Promoting Healthy Life. World Health Organization. doi:10.1080/1357628031000116808.
- Zhu, C., K. Kobayashi, I. Loladze, J. Zhu, Q. Jiang, X. Xu, G. Liu, et al. 2018. “Carbon Dioxide (CO2) Levels This Century Will Alter the Protein, Micronutrients and Vitamin Content of Rice Grains with Potential Health Consequences for the Poorest Rice-dependent Countries.” Science Advances 4: eaaq1012. doi:10.1126/sciadv.aaq1012.