ABSTRACT
Nickel (Ni) is an essential cofactor for various microbial enzymes, but in higher plants, few Ni-containing enzymes (e.g., urease) have been identified. Here, we explored the use of liquid chromatography–inductively coupled plasma mass spectrometry (ICPMS) to identify novel Ni-containing proteins in Arabidopsis thaliana. Water-soluble proteins were extracted from the roots of A. thaliana, and metal-containing proteins in the extracts were separated and detected by means of a two-dimensional procedure involving size-exclusion chromatography–ICPMS and anion-exchange chromatography–ICPMS. Using this procedure, we obtained five separated Ni peaks that were present both in the wild type and a Urease-defective mutant, indicating the presence of Ni-containing proteins other than urease. One of the Ni peaks showed a significant correlation with an iron (Fe) peak, suggesting that a protein contained in this peak incorporated both Ni and Fe. To identify this protein, we used liquid chromatography–tandem mass spectrometry to perform a proteome analysis of the Ni and Fe peak. Among the 269 proteins detected, we identified acireductone dioxygenase 2; the murine and bacterial acireductone dioxygenases have two distinct metalloforms containing either Ni or Fe. Thus, acireductone dioxygenase 2 is most likely a novel Ni-containing enzyme in A. thaliana. Our findings suggest that most of the Ni-containing proteins in higher plants remain uncharacterized.
1. Introduction
Nickel (Ni) acts as a cofactor for various enzymes in biological systems; for example, urease (URE), Ni-iron (Fe) hydrogenase, and Ni superoxide dismutase require Ni for their activities (Ragsdale Citation2009). Most of the known Ni-containing enzymes have been discovered in bacteria, and Ni is an essential trace element for many bacteria (Ragsdale Citation2009). On the other hand, only a few Ni-containing enzymes have been identified in higher plants. Until very recently, the only Ni-containing enzyme that had been identified in higher plants was URE (Dixon et al. Citation1975), which catalyzes the hydrolysis of urea to generate carbon dioxide and ammonia. Because URE is essential for nitrogen metabolism in plants, Ni is an essential plant micronutrient (Eskew, Welch, and Cary Citation1983; Brown, Welch, and Cary Citation1987). Recently, Yang et al. (Citation2018) and Zheng et al. (Citation2019) have identified novel Ni-containing enzymes from Arabidopsis thaliana: the H3K27me3 demethylase JUMONJI 13 and the H3K4me3 demethylase JUMONJI 14, which are the flowering-time regulators, have been shown to require Ni2+ at the active site. A search of the Protein Data Bank Japan (https://pdbj.org/mine) reveals three other plant-derived proteins (whirly protein, hydroxyglutarate synthase, gurmarin) reported to contain Ni in their crystal structures. However, whether Ni is essential for the activity of these proteins is unknown.
Nickel, Fe, and cobalt (Co) – generally referred to as the Fe group metals – show analogous behaviors in the metal transport systems of plants. For example, in A. thaliana, iron-regulated transporter 1 (IRT1) is the primary Fe-uptake transporter in roots; it mediates the absorption of not only Fe2+ but also Ni2+ and Co2+ (Korshunova et al. Citation1999; Vert et al. Citation2002; Nishida et al. Citation2011). Another transporter, iron-regulated 2, is responsible for sequestering excess cytosolic Ni2+ into root vacuoles in A. thaliana; it also transports Fe2+ and Co2+ (Schaaf et al. Citation2006; Morrissey et al. Citation2009). In addition, the similarity between the chemical properties of Ni and Fe has been shown to be partially responsible for the phytotoxicity of Ni: specifically, excess Ni accumulation causes symptoms of Fe deficiency (e.g., chlorosis) in plants, even when the tissue Fe level is sufficient (Nishida et al. Citation2011). Such Ni-induced Fe deficiency has been observed in crops grown in Ni-rich soils and is thought to be caused by disruption of Fe homeostasis due to competition between Ni and Fe at the cellular level (Nishida et al. Citation2011; Nishida, Aisu, and Mizuno Citation2012; Lešková et al. Citation2020), although the molecular mechanism is unknown.
The behaviors of Ni at the tissue and cellular levels (e.g., absorption by roots, translocation from roots to shoots, sequestration into vacuoles) have been well studied in plants (Schaaf et al. Citation2006; Gendre et al. Citation2007; Morrissey et al. Citation2009; Nishida et al. Citation2011, Citation2020); however, information about Ni behavior in the proteome is limited. Various techniques have been developed for identifying metal-containing proteins (metalloproteins) and elucidating their behavior. The most frequently used technique is liquid chromatography coupled with inductively coupled plasma mass spectrometry (LC-ICPMS), in which metalloproteins separated by LC are directly transferred to the ICPMS system for detection and quantification (Sanz-Medel, Montes-Bayón, and Sánchez Citation2003; Suzuki, Sakai, and Furuta Citation2012; Suzuki et al. Citation2013). In this study, we explored the use of LC-ICPMS to identify novel Ni-containing proteins from plants. The proteins were isolated from the water-soluble fraction extracted from the roots of A. thaliana and were analyzed by a two-dimensional procedure involving size-exclusion chromatography (SEC)–ICPMS and anion-exchange chromatography (AEC)–ICPMS. The detected proteins were further analyzed by LC combined with tandem mass spectrometry (LC–MS/MS).
2. Materials and methods
2.1. Plant materials and growth conditions
The urease (URE)–defective mutant (ure, SALK_038002; Witte, Rosso, and Romeis Citation2005) of A. thaliana has a T-DNA insertion in URE (AT1G67550); it was obtained from the Arabidopsis Biological Resource Center (The Ohio State University, USA). The homozygous line of ure was isolated using polymerase chain reaction with primers flanking the T-DNA insertion site (5´-TGGTAGGTGATATCAAGACCTATG-3´, 5´-TGCTATAGTGCTGTAGGTGTCTCTC-3´) and the left-border primer of T-DNA (5´-TGGTTCACGTAGTGGGCCATCG-3´). The Col-0 accession (background of ure) was used as the corresponding wild type. Eighty seedlings were grown in hydroponic culture as described previously (Nishida et al. Citation2011), and 6-week-old plants were transferred to hydroponic solution supplemented with 65 nmol L−1 NiCl2 and cultivated in a growth room at 22°C under a 10-h light/14-h dark cycle. Roots of 7-week-old plants were harvested in liquid nitrogen. The hydroponic solution was renewed every week.
2.2. Protein extraction
Frozen root tissue was ground in a mortar and suspended in nine volumes of 3.5 mM Tris-HNO3 (pH 7.4). The suspension was centrifuged (2,880 g, 4°C, 20 min) twice to remove tissue debris, and the water-soluble-protein fraction was isolated by ultracentrifugation (100,000 g, 4°C, 1 h). The isolate was concentrated 100-fold by ultrafiltration (Vivaspin®-3 K, Sartorius Stedim Japan), freeze-dried, and redissolved in 50 mM Tris-HNO3 (pH 7.4). Protein concentrations were determined by the Bradford protein assay and adjusted to 10–20 mg mL−1. On average 0.3 mg protein per plant was obtained.
2.3. LC-ICPMS procedures
A scheme of the SEC/AEC-ICPMS system is shown in . SEC was conducted with a high-performance LC pump (PU-1580i, JASCO) equipped with a size-exclusion column (PROTEIN KW-803, φ8 × 300 mm, Showa Denko) and a guard column (Asahipak GS-2 G 7B, φ7.5 × 50 mm, Showa Denko). Tris-HNO3 (50 mM, pH 7.4) was used as the eluent. AEC was conducted with the same LC pump and an anion-exchange column (IEC QA-825, φ8 × 75 mm, Showa Denko). Tris-CH3COOH (20 mM, pH 8.0) and a mixture of 20 mM Tris-CH3COOH and 1 M CH3COONH4 (pH 8.0) were used as the eluents. The linear gradient of CH3COONH4 concentration was as follows: 0 M, 0–10 min; 0–0.5 M, 10–50 min; and 0.5–1 M, 50–60 min. The eluent flow rates were 0.6 mL min−1 for SEC and 0.8 mL min−1 for AEC, and the sample volume was 100 µL in both analyses. The outlet of the LC column was connected to an ICPMS instrument (7500ce, Agilent Technologies Japan). The ICPMS operating parameters were as follows: radiofrequency power, 1,550 W; plasma argon flow rate, 15 L min−1; auxiliary argon gas flow rate, 1.0 L min−1; and collision helium gas flow rate, 3.0 mL min−1. The following isotopes of 14 elements were measured: 11B, 23Na, 24Mg, 31P, 35Cl, 37Cl, 39K, 43Ca, 44Ca, 55Mn, 57Fe, 59Co, 60Ni, 63Cu, 66Zn, 95Mo. The SEC-ICPMS and AEC-ICPMS results were evaluated by analysis of metalloprotein reference standards (Figure S1).
Figure 1. A scheme of the system used for size-exclusion chromatography (SEC) and anion-exchange chromatography (AEC) coupled with inductively coupled plasma mass spectrometry (ICPMS). Eluents: 50 mM Tris-HNO3 (pH 7.4) for SEC; 20 mM Tris-CH3COOH (pH 8.0) and a mixture of 20 mM Tris-CH3COOH (pH 8.0) and 1 M CH3COONH4 (pH 8.0) for AEC. The guard column was used only for SEC
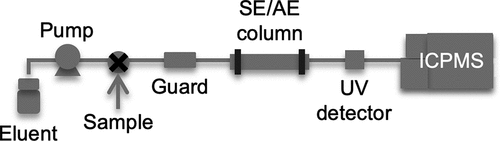
Protein (0.2–0.3 mg) in the eluent was subjected to SEC-ICPMS to obtain Ni chromatograms. Protein (2–3 mg) was subjected to SEC (without ICPMS), and the Ni-containing fractions were collected according to their retention times: 10.4–12.4 min (fraction 1), 12.4–15.3 min (fraction 2), and 15.3–18.3 min (fraction 3). To exchange the solvent, the fractions were concentrated by ultrafiltration from 1 to 2 mL to 180 µL or less, diluted with four volumes of 20 mM Tris-CH3COO3NH4, concentrated again by ultrafiltration to 150 µL, and 100 µL were used for AEC-ICPMS analysis.
2.4. Proteome analysis
2.4.1. Isolation of Ni-containing fractions
Protein (2.8 mg) was subjected to SEC; the Ni-containing fractions were collected and solvent was exchanged to 20 mM Tris-CH3COO3NH4 as described above, and 100 µL was subjected to AEC. The Ni-containing peaks were isolated according to their retention times: 16.1–19.7 min (peak III) and 30–31.4 min (peak V); the fractions were concentrated by ultrafiltration (from 1 to 3 mL to 100 µL), freeze-dried, and diluted to 20 µL with ultrapure water.
2.4.2. Trypsin digestion
Samples for LC-MS/MS were prepared as in Matsuura et al. (Citation2018) and Suzuki et al. (Citation2019) with minor modifications. Briefly, 80 µL of solution containing 7 M guanidine hydrochloride, 0.5 M Tris, and 10 mM ethylenediamine-N,N,N’,N’-tetraacetic acid (pH 8.0), and 1 µL of 0.5 M dithiothreitol were added to the Ni-containing fractions, and the samples were incubated for 30 min at 60°C to reduce proteins. Subsequently, 2 µL of 0.5 M iodoacetamide was added, the samples were incubated for 1 h at room temperature for protein alkylation, and then mixed with 8 volumes of 4:1:3 methanol/chloroform/water and centrifuged (14,000 rpm, 5 min, 4°C). The upper layers were transferred to new tubes, cooled on ice, mixed with 300 µL of methanol, and centrifuged (14,000 rpm, 5 min, 4°C). The precipitates were washed with 200 µL of 70% ethanol, dried by vacuum evaporation, redissolved in 13 µL of water, mixed with 26 µL of 50 mM ammonium bicarbonate (pH 8.0) and digested with 500 ng of Trypsin Gold (Promega) overnight at 37°C. The digestion was terminated with trifluoroacetic acid (0.1%), and the samples were concentrated to 10–15 µL by vacuum evaporation.
2.4.3. LC-MS/MS
Tryptic digests were analyzed by LC-MS/MS (Matsuura et al. Citation2018) on an LTQ-Orbitrap Velos mass spectrometer (Thermo Fisher Scientific) equipped with a Zaplous Advance nano UHPLC HTS-PAL xt System (AMR). Mixtures of (A) 0.1% aqueous formic acid and (B) 100% acetonitrile were used as the mobile phase. The samples were loaded onto a trap column (0.3 mm × 5 mm, 5 µm particle diameter, L-column; CERI) that was directly connected to a Zaplous α Pep-C18 packed column (0.1 mm × 150 mm, 3 µm particle diameter; AMR). The flow rate was 500 nL min−1, and peptides were eluted with a linear gradient of 5–45% mobile phase B over 20 min. Data were analyzed with Proteome Discoverer software (version 2.1, Thermo Fisher Scientific) with the reference genome sequence data of A. thaliana (TAIR10) downloaded from the TAIR10 FTP server (ftp://ftp.arabidopsis.org/home/tair/). Information about the cofactor in each detected protein was retrieved from the UniProt database (https://www.uniprot.org).
3. Results
Water-soluble proteins were extracted from the roots of wild-type A. thaliana (Col-0) and were analyzed by SEC-ICPMS. Three Ni fractions eluted within the retention time range of 10–18 min (fractions 1–3; , upper panel). According to a regression line for the relationship between protein molecular weight (MW) and retention time, the MW ranges of the proteins corresponding to these three fractions were expected to be 514–700, 241–514, and <241 kDa, respectively (Figure S1). The same three Ni fractions were detected in samples from the ure mutant (, lower panel), confirming that these were not derived from URE. The Ni fractions that eluted after 20 min showed poor reproducibility across experiments (data not shown) and were attributed to nonprotein molecules on the basis of their retention times.
Figure 2. Analysis of Ni-containing proteins by size-exclusion chromatography–inductively coupled plasma mass spectrometry (SEC-ICPMS). Water-soluble-proteins extracted from the roots of Arabidopsis thaliana grown in hydroponic culture were fractionated and analyzed by SEC-ICPMS. Data for the wild type (Col-0) and the urease-defective mutant (ure) are shown in the upper and lower panels, respectively. The retention times for protein standards are indicated by arrows (urease, 480 kDa; catalase, 245 kDa; superoxide dismutase 1, 32.5 kDa). Analyses were repeated three times for Col-0 and twice for ure, and representative chromatograms are shown
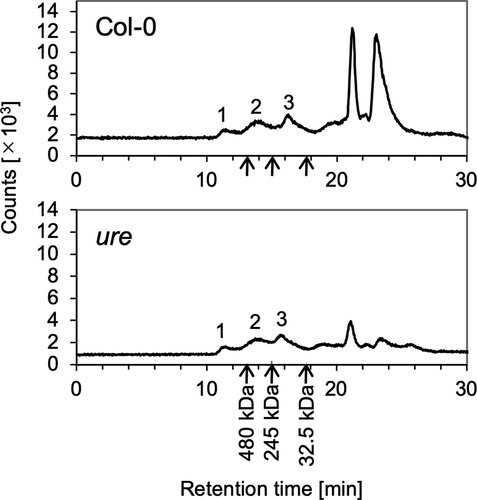
Each Ni fraction was collected and analyzed by AEC-ICPMS, with simultaneous monitoring of the 14 elements contained in the hydroponic solution. Unique chromatograms were obtained for each element, indicating the presence of various proteins containing each element (Figure S2). The concentrations of magnesium (Mg) and calcium (Ca) are well correlated in the plant ionome (Baxter et al. Citation2012), and correlation between Mg and Ca at the proteome level was confirmed by our experiments (Figure S2). In the Ni chromatograms, five peaks (I–V) and unbound proteins were detected in both the wild type ()) and ure (Figure S3A); fraction 1 yielded no separate peak, fraction 2 yielded peaks I–III, and fraction 3 yielded peaks IV and V. The unbound peaks were attributed to alkaline proteins that did not bind to the anion-exchange column at the pH used for the analysis. Pearson’s correlation coefficients for the relationships between Ni and each of the other elements were calculated for each of the five Ni peaks in both Col-0 and ure () and S3B). Significant correlations (coefficients ≥0.7) were obtained for Ni with Mg and Co in peak III and with Fe in peak V in both the wild type ()) and the mutant (Figure S3B, C). Thus, Ni-containing proteins in peak III also contained Co and Mg and those in peak V also contained Fe. No reproducible significant correlations between Ni and any other elements were observed for the other Ni peaks, suggesting that Ni was incorporated specifically by the proteins in these peaks.
Figure 3. Analysis of Ni-containing proteins by anion-exchange chromatography–inductively coupled plasma mass spectrometry (AEC-ICPMS). Fractions with Ni-containing proteins detected by size-exclusion chromatography–ICPMS (fractions 1–3) were collected and analyzed by AEC-ICPMS. Representative results of three replicates for Col-0 are shown (results for ure are shown in Figure S3). (a) Ni detection. Reproducible peaks are indicated by arrowheads, and peaks corresponding to unbound proteins are indicated by dotted arrows. Signal intensities are expressed in counts per million (CPM). (b) Heatmap showing Pearson’s correlation coefficients for the relationship between Ni and other elements in peaks I–V. Pairs showing highly positive correlation (indicated by a coefficient of ≥0.7) for both Col-0 and ure are indicated in white type. (C) Chromatograms of Ni and other metals for peak III (left) and peaks IV and V (right)
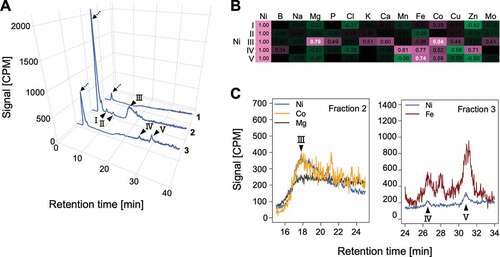
To identify the Ni-containing proteins in peaks III and V, we used LC-MS/MS to conduct a proteome analysis. No candidate proteins were identified in peak III (data not shown). In contrast, analysis of peak V identified 269 potentially Ni-containing A. thaliana proteins (Data S1). No proteins annotated as containing Ni cofactor in the UniProt database were found among these 269 candidates (Data S1). Of these proteins, 35 were annotated as metalloproteins, and 7 were known or predicted to be Fe-containing proteins (Figure S4, ). Of these seven proteins, acireductone dioxygenase (ARD) 2 was annotated as having a metal-binding motif with the potential to bind both Fe2+ and Ni2+. ARD is ubiquitous in bacteria, mammals, and plants and plays an essential role in the methionine recycling pathway (described in detail in the discussion section) (Sauter et al. Citation2005; Pochapsky et al. Citation2006; Xu et al. Citation2006; Bürstenbinder et al. Citation2007). The structural and biochemical properties of ARD from the bacterium Klebsiella oxytoca and the mouse are well characterized. There are two metalloforms of ARD for the same polypeptide, one containing Fe (Fe-ARD) and one containing Ni (Ni-ARD), and they have been shown to have different activities toward their common substrate (Pochapsky et al. Citation2006; Xu et al. Citation2006; Deshpande et al. Citation2016). The Subcellular Localization Database for Arabidopsis Proteins predicts, with 100% probability, that the A. thaliana ARD2 localizes in the cytosol (data are available at https://suba.live), as do ARD orthologs. According to the Arabidopsis eFP Browser, ARD2 is expressed in all plant tissues (including roots, which were used in this study) (data are available at http://www.bar.utoronto.ca/). On the basis of all these data, we assumed ARD2 to be the most likely Ni-containing protein in peak V.
Table 1. Fe-containing proteins identified by liquid chromatography–tandem mass spectrometry in peak V.†.
4. Discussion
In this study, we detected multiple Ni-containing proteins in the water-soluble fraction of A. thaliana roots by two-dimensional LC-ICPMS. This result indicates that there are multiple proteins with the potential to bind Ni in A. thaliana roots. Ni-containing proteins in plants might remain largely uncharacterized. AEC-ICPMS analysis of a URE standard indicated that URE would be expected to appear at a retention time of approximately 32.8 min in fraction 1 (, , S1, and S3). However, no peak for URE was detected, whereas multiple Ni peaks were detected in fractions 2 and 3, indicating that Ni-containing proteins that were more abundant than URE were present. In peak V, Ni was significantly correlated with Fe, and we, therefore, expected that the Ni-containing protein in peak V contained Fe as well. Using LC-MS/MS analysis, we detected 269 proteins in peak V; only 7 of these, including ARD2, were annotated as being Fe-containing proteins. This result indicates that analyzing correlations between metals may be an efficient strategy for narrowing down candidates when exploring proteins having more than one metal cofactor or having multiple forms with different metal cofactors.
ARD plays an essential role in the methionine salvage pathway, which is ubiquitous in eukaryotic and prokaryotic organisms; in this cyclic pathway, 5´-methylthioadenosine – a by-product of ethylene synthesis, polyamine synthesis, and nicotianamine synthesis – is transformed into methionine (Sauter et al. Citation2005; Bürstenbinder et al. Citation2007). ARD exhibits different chemical properties depending on the metal species in the active site: Fe-ARD catalyzes ‘on-pathway’ cleavage of 1,2-dihydoxy-3-keto-5-(thiomethyl)pent-1-ene to generate formate and 2-keto-4-(thiomethyl)butyrate, which is the precursor of methionine. Although the Ni-ARD uses the same substrate, this metalloform catalyzes an ‘off-pathway’ reaction that produces methylthiopropionate, formate, and carbon monoxide (CO). Free methionine concentration tends to be higher in Ni-deficient foliage than in Ni-sufficient foliage in the tree plant Carya illinoinensis (Bai, Reilly, and Wood Citation2006), implying the increased abundance of Fe-ARD relative to that of Ni-ARD in the absence of Ni. The biological function of the off-pathway reaction is unclear, but it may be involved in pathology in mammals (Deshpande, Pochapsky, and Ringe Citation2017). In plants, CO is known to induce the Fe-uptake systems of roots and to alleviate Fe deficiency (Kong et al. Citation2010; Yang et al. Citation2016). Fe deficiency may decrease the amount of Fe-ARD. If the abundance of Ni-ARD increased relative to that of Fe-ARD under Fe deficiency and the off-pathway reaction was activated, the resulting elevated CO levels would induce Fe uptake by the roots and would thus contribute to the restoration of Fe-ARD activity. Consistent with this hypothesis, IRT1 is higher expressed in the presence of Ni than in the absence of Ni under Fe deficiency (Nishida et al. Citation2011). Further investigation of the ARD metalloforms of A. thaliana would elucidate the function and essentiality of Ni in plants.
Peak III was the largest of the peaks in the AEC-ICPMS chromatograms (excluding the peaks of unbound proteins), and we expected this peak to contain a novel protein with the potential to bind both Ni and Co. However, LC-MS/MS failed to reveal any proteins in this peak (data not shown). One possible explanation for this is that plant-derived polymeric contaminants, such as polysaccharides, may have prevented detection of the proteins by MS/MS. Optimization of the solvent gradient or additional purification might allow for separation of the target protein from such contaminants. Another possibility is interference due to presence of a protein that was insufficiently digested by trypsin owing to modifications such as methylation and acetylation. Using a protease with different specificity (e.g., chymotrypsin) might solve this problem. Large Ni peaks in the unbound-protein fractions probably contained complex mixtures of proteins. At pH 8 used in this study, those proteins were neutral or positively charged, but it might be possible to separate them at a higher pH or by cation-exchange chromatography, which can be used for the separation of positively charged proteins. Furthermore, additional as-yet-unknown Ni-containing proteins may be present in the shoots or other parts of the plants, and isolation of these proteins might shed light on tissue-specific functions of Ni.
Acknowledgments
We thank the ABRC Stock Center for providing seeds of the T-DNA insertion line.
Disclosure statement
The authors have no conflicts of interest.
Additional information
Funding
References
- Bai, C., C. C. Reilly, and B. W. Wood. 2006. “Nickel Deficiency Disrupts Metabolism of Ureides, Amino Acids, and Organic Acids of Young Pecan Foliage.” Plant Physiology 140 (2): 433–443. doi:10.1104/pp.105.072983.
- Baxter, I., C. Hermans, B. Lahner, E. Yakubova, M. Tikhonova, N. Verbruggen, D.-Y. Chao, and D. E. Salt. 2012. “Biodiversity of Mineral Nutrient and Trace Element Accumulation in Arabidopsis Thaliana.” PLoS One 7: 4. doi:10.1371/journal.pone.0035121.
- Brown, P. H., R. M. Welch, and E. E. Cary. 1987. “Nickel: A Micronutrient Essential for Higher Plants.” Plant Physiology 85 (3): 801–803. doi:10.1104/pp.85.3.801.
- Bürstenbinder, K., G. Rzewuski, M. Wirtz, R. Hell, and M. Sauter. 2007. “The Role of Methionine Recycling for Ethylene Synthesis in Arabidopsis.” The Plant Journal 49 (2): 238–249. doi:10.1111/j.1365-313X.2006.02942.x.
- Deshpande, A. R., T. C. Pochapsky, and D. Ringe. 2017. “The Metal Drives the Chemistry: Dual Functions of Acireductone Dioxygenase.” Chemical Reviews 117 (15): 10474–10501. doi:10.1021/acs.chemrev.7b00117.
- Deshpande, A. R., K. Wagenpfeil, T. C. Pochapsky, G. A. Petsko, and D. Ringe. 2016. “Metal-dependent Function of a Mammalian Acireductone Dioxygenase.” Biochemistry 55 (9): 1398–1407. doi:10.1021/acs.biochem.5b01319.
- Dixon, N. E., C. Gazzola, R. L. Blakeley, and B. Zerner. 1975. “Jack Bean Urease (EC 3.5. 1.5). Metalloenzyme. Simple Biological Role for Nickel.” Journal of the American Chemical Society 97 (14): 4131–4133. doi:10.1021/ja00847a045.
- Eskew, D. L., R. M. Welch, and E. E. Cary. 1983. “Nickel: An Essential Micronutrient for Legumes and Possibly All Higher Plants.” Science 222 (4624): 621–623. doi:10.1126/science.222.4624.621.
- Gendre, D., P. Czernic, G. Conéjéro, K. Pianelli, J. F. Briat, M. Lebrun, and S. Mari. 2007. “TcYSL3, a Member of the YSL Gene Family from the Hyper‐accumulator Thlaspi Caerulescens, Encodes a nicotianamine‐Ni/Fe Transporter.” The Plant Journal 49 (1): 1–15. doi:10.1111/j.1365-313X.2006.02937.x.
- Kong, W. W., L. P. Zhang, K. Guo, Z. P. Liu, and Z. M. Yang. 2010. “Carbon Monoxide Improves Adaptation of Arabidopsis to Iron Deficiency.” Plant Biotechnology Journal 8 (1): 88–99. doi:10.1111/j.1467-7652.2009.00469.x.
- Korshunova, Y. O., D. Eide, W. G. Clark, M. L. Guerinot, and H. B. Pakrasi. 1999. “The IRT1 Protein from Arabidopsis Thaliana Is a Metal Transporter with a Broad Substrate Range.” Plant Molecular Biology 40 (1): 37–44. doi:10.1023/a:1026438615520.
- Lešková, A., M. Zvarík, T. Araya, and R. F. Giehl. 2020. “Nickel Toxicity Targets Cell Wall-related Processes and PIN2-mediated Auxin Transport to Inhibit Root Elongation and Gravitropic Responses in Arabidopsis.” Plant & Cell Physiology 61 (3): 519–535. doi:10.1093/pcp/pcz217.
- Matsuura, A., K. Yoshimura, H. Kintsu, T. Atsumi, Y. Tsuchihashi, T. Takeuchi, N. Satoh, et al. 2018. “Structural and Functional Analyses of Calcium Ion Response Factors in the Mantle of Pinctada Fucata.” Journal of Structural Biology 204 (2): 240–249. doi:10.1016/j.jsb.2018.08.014.
- Morrissey, J., I. R. Baxter, J. Lee, L. Li, B. Lahner, N. Grotz, J. Kaplan, D. E. Salt, and M. L. Guerinot. 2009. “The Ferroportin Metal Efflux Proteins Function in Iron and Cobalt Homeostasis in Arabidopsis.” The Plant Cell 21 (10): 3326–3338. doi:10.1105/tpc.109.069401.
- Nishida, S., A. Aisu, and T. Mizuno. 2012. “Induction of IRT1 by the Nickel-induced Iron-deficient Response in Arabidopsis.” Plant Signaling & Behavior 7 (3): 329–331. doi:10.4161/psb.19263.
- Nishida, S., R. Tanikawa, S. Ishida, J. Yoshida, T. Mizuno, H. Nakanishi, and N. Furuta. 2020. “Elevated Expression of Vacuolar Nickel Transporter Gene IREG2 Is Associated with Reduced Root-to-shoot Nickel Translocation in Noccaea Japonica.” Frontiers in Plant Science 11: 610. doi:10.3389/fpls.2020.00610.
- Nishida, S., C. Tsuzuki, A. Kato, A. Aisu, J. Yoshida, and T. Mizuno. 2011. “AtIRT1, the Primary Iron Uptake Transporter in the Root, Mediates Excess Nickel Accumulation in Arabidopsis Thaliana.” Plant & Cell Physiology 52 (8): 1433–1442. doi:10.1093/pcp/pcr089.
- Pochapsky, T. C., S. S. Pochapsky, T. Ju, C. Hoefler, and J. Liang. 2006. “A Refined Model for the Structure of Acireductone Dioxygenase from Klebsiella ATCC 8724 Incorporating Residual Dipolar Couplings.” Journal of Biomolecular NMR 34 (2): 117–127. doi:10.1007/s10858-005-5735-8.
- Ragsdale, S. W. 2009. “Nickel-based Enzyme Systems.” Journal of Biological Chemistry 284 (28): 18571–18575. doi:10.1074/jbc.R900020200.
- Sanz-Medel, A., M. Montes-Bayón, and M. L. F. Sánchez. 2003. “Trace Element Speciation by ICP-MS in Large Biomolecules and Its Potential for Proteomics.” Analytical and Bioanalytical Chemistry 377 (2): 236–247. doi:10.1007/s00216-003-2082-z.
- Sauter, M., R. Lorbiecke, B. Ouyang, T. C. Pochapsky, and G. Rzewuski. 2005. “The Immediate-early Ethylene Response Gene OsARD1 Encodes an Acireductone Dioxygenase Involved in Recycling of the Ethylene Precursor S-adenosylmethionine.” The Plant Journal 44 (5): 718–729. doi:10.1111/j.1365-313X.2005.02564.x.
- Schaaf, G., A. Honsbein, A. R. Meda, S. Kirchner, D. Wipf, and N. von Wirén. 2006. “AtIREG2 Encodes a Tonoplast Transport Protein Involved in Iron-dependent Nickel Detoxification in Arabidopsis Thaliana Roots.” Journal of Biological Chemistry 281 (35): 25532–25540. doi:10.1074/jbc.M601062200.
- Suzuki, M., K. Kubota, R. Nishimura, L. Negishi, K. Komatsu, H. Kagi, K. Rehav, S. Cohen, and S. Weiner. 2019. “A Unique Methionine-rich Protein-aragonite Crystal Complex: Structure and Mechanical Functions of the Pinctada Fucata Bivalve Hinge Ligament.” Acta biomaterialia 100: 1–9. doi:10.1016/j.actbio.2019.10.008.
- Suzuki, Y., Y. Hashiura, T. Sakai, T. Yamamoto, T. Matsukawa, A. Shinohara, and N. Furuta. 2013. “Selenium Metabolism and Excretion in Mice after Injection of 82Se-enriched Selenomethionine.” Metallomics 5 (5): 445–452. doi:10.1039/c3mt20267d.
- Suzuki, Y., T. Sakai, and N. Furuta. 2012. “Isolation of selenoprotein-P and Determination of Se Concentration Incorporated in Proteins in Human and Mouse Plasma by Tandem Heparin Affinity and Size-exclusion Column HPLC-ICPMS.” Analytical Sciences 28 (3): 221. doi:10.2116/analsci.28.221.
- Vert, G., N. Grotz, F. Dédaldéchamp, F. Gaymard, M. L. Guerinot, J. F. Briat, and C. Curie. 2002. “IRT1, an Arabidopsis Transporter Essential for Iron Uptake from the Soil and for Plant Growth.” The Plant Cell 14 (6): 1223–1233. doi:10.1105/tpc.001388.
- Witte, C. P., M. G. Rosso, and T. Romeis. 2005. “Identification of Three Urease Accessory Proteins that are Required for Urease Activation in Arabidopsis.” Plant Physiology 139 (3): 1155–1162. doi:10.1104/pp.105.070292.
- Xu, Q., R. Schwarzenbacher, S. S. Krishna, D. McMullan, S. Agarwalla, K. Quijano, P. Abdubek, et al. 2006. “Crystal Structure of Acireductone Dioxygenase (ARD) from Mus Musculus at 2.06 Å Resolution.” Proteins: Structure, Function, and Bioinformatics 64 (3): 808–813. doi:10.1002/prot.20947.
- Yang, L., J. Ji, H. Wang, K. R. Harris-Shultz, E. F. Abd Allah, Y. Luo, Y. Guan, and X. Hu. 2016. “Carbon Monoxide Interacts with Auxin and Nitric Oxide to Cope with Iron Deficiency in Arabidopsis.” Frontiers in Plant Science 7: 112. doi:10.3389/fpls.2016.00112.
- Yang, Z., Q. Qiu, W. Chen, B. Jia, X. Chen, H. Hu, K. He, et al. 2018. “Structure of the Arabidopsis JMJ14-H3K4me3 Complex Provides Insight into the Substrate Specificity of KDM5 Subfamily Histone Demethylases.” The Plant Cell 30 (1): 167–177. doi:10.1105/tpc.17.00666.
- Zheng, S., H. Hu, H. Ren, Z. Yang, Q. Qiu, W. Qi, X. Liu, et al. 2019. “The Arabidopsis H3K27me3 Demethylase JUMONJI 13 Is a Temperature and Photoperiod Dependent Flowering Repressor.” Nature Communications 10 (1): 1303. doi:10.1038/s41467-019-09310-x.