ABSTRACT
Increasing carbon storage in soil is a potential measure against global warming, but the mechanisms of carbon accumulation in soil are not well understood. Clarifying the mechanism would help in the development of new methods for soil carbon storage, such as in agricultural systems. We grew high-biomass forage rice cultivars for 11 years under conditions of potassium and silicic acid deficiency. Rice cultivars were healthy, without signs of potassium or silicic acid deficiency. The quantities of potassium and silicic acid absorbed by the rice plants were greater than those of available forms in the soil, fertilizer, and irrigation water, indicating that mineral weathering promoted the release of potassium, silicic acid, and aluminum from primary minerals. Furthermore, the carbon bound to active aluminum increased by 69.4 kg C ha−1 year−1 during 11 years of paddy rice cultivation. Although organic acids secreted by rice roots have been expected to be a factor in the strong mineral weathering effect in the rhizosphere, an only extremely small amount of organic acids were detected from rice roots. Therefore, organic acids are not a factor in promoting mineral weathering. On the other hand, leaching of potassium and silicic acid is observed in the contact reaction between the mineral powder and the cell wall prepared from rice root. These findings show that the primary minerals could be reacted with chelating sites on the cell wall of the rice root surface to dissolve potassium, aluminum, and silicic acid. The rice plants absorb potassium and a large amount of silicic acid under potassium and silicic acid deficient conditions. Then, the highly active aluminum remained in the rhizosphere, where it bound to organic matter, producing persistent soil carbon. Plants that can absorb silicic acid vigorously have a role of increasing active aluminum through mineral weathering, resulting contribute to carbon accumulation in soil.
1. Introduction
In volcanic regions, high concentrations of soil organic matter are observed in both tropical and temperate zones (Kato Citation1978; Brady and Weil Citation2008). Pyroclastic sediments that contain fine materials are easily weathered. In some volcanic regions (for example, Japan, Indonesia, and the Philippines), soil carbon contents are greater than 6% (Takahashi and Shoji Citation2002). These carbon-rich soils (classified as Andosols) contain highly stable organic matter bound to Al and Fe that is highly resistant to degradation by soil microorganisms.
Silicon (Si) is present in large quantities in many soil types. In general, plants contain no more than 10 g SiO2 kg−1 dry matter. However, poaceous plants such as rice (Oryza sativa L.) have a high silicic acid (SiO2) content, about 100 g SiO2 kg−1 dry matter (Takahashi Citation1974). Thus, the rice plants producing an average grain yield (5000 kg ha−1 in Japan) must absorb as much as 1000 to 1200 kg SiO2 ha−1 every year. To investigate the effects of soil fertility on rice cultivation, field experiments focused on the three major soil nutrients (nitrogen, N; phosphate, P; and potassium, K) have been conducted over 70–80 years at several agricultural experiment stations in Japan (Hyogo, 35°0′N, 135°1′E; Aichi, 35°0′N, 140°1′E; and Fukushima, 38°0′N, 141°1′E). In the field experiments, the rice yield fertilized with NP is almost identical to those with NPK (Shiota, Sano, and Okimura Citation1984; Ogawa, Kuwana, and Ushio Citation2004), indicating that rice plants have strong ability for the absorption of K and SiO2, and may absorb them from an unknown source in the soil.
Sugiyama and Ae (Citation2000) and Akagi et al. (Citation2006) have reported that upland rice plants absorb K and SiO2 from biotite, muscovite, and orthoclase. However, the absorption mechanism has not been identified. In addition, when a rice mutant (Lsi-1; Ma et al. Citation2002) defective in Si uptake was cultivated, less active aluminum (Al) remained in the rhizosphere than when wild-type rice was cultivated (Hobara et al. Citation2016). Thus, rice plants, which have high mineral weathering and strong SiO2 absorption abilities, increase the active Al content in the rhizosphere. Toxic and active Al also remains in the rhizosphere if primary minerals are weathered by plants. The persistent organic matter in soil (i.e., stored carbon or humic substances) is stabilized through the formation of complexes with metal elements such as Al and Fe (Gulde et al. Citation2008; Wei et al. Citation2017), and Al, in particular, contributes greatly to carbon accumulation in paddy soil through this mechanism. Thus, the ability of rice plants to absorb K and SiO2 from minerals strongly contributes to the stabilization of soil organic matter via the release of Al from minerals.
In recent years, forage rice cultivars (FRCs) with excellent dry biomass production have been developed as alternatives to imported feed in Japan. FRCs show higher K and Si uptake than conventional edible rice cultivars (ERCs). We hypothesized that cultivating FRCs, with their high Si uptake, would increase the quantity of active Al in the soil and thus also increase the accumulation of soil carbon. To test this hypothesis, we cultivated FRCs for 11 years in paddy fields under Si and K deficient conditions and monitored changes in the K and Si balances and persistent soil carbon accumulation. We also examined whether rice roots promote the weathering of soil minerals such as orthoclase and volcanic glass (obsidian). One way that the roots of some plants can promote mineral weathering is by exuding organic acids (i.e., citric acid, oxalic acid) (Ae et al. Citation1990; Badri and Vivanco Citation2009). However, other mechanisms are possible. For example, groundnuts can grow in soil with low phosphorous fertility, but they exude only very small amounts of organic acids (Otani, Ae, and Tanaka Citation1996). Ae, Shen, and Otani (Citation2001) suggested that surfaces of groundnut roots can chelate Al and Fe, and termed the interaction between the root surface and Al/Fe compounds (minerals) a ‘contact reaction’. Because rice roots exude only very small quantities of organic acids (Otani, Ae, and Tanaka Citation1996), contact reaction may occur on rice roots surface similar to groundnut roots. In addition, in paddy environments active substances (such as organic acids) exuded by roots and by rhizosphere microorganisms are diluted with large amounts of irrigation water, decreasing their weathering effect until it is negligible. Thus, we hypothesized that rice breaks down primary minerals containing K and Si via a contact reaction between the root surface and the mineral surface, and the consequent production of active Al promotes carbon accumulation.
2. Materials and methods
2.1. Cultivation
From 2005 to 2015, we cultivated four FRCs in paddy fields (36°0′N, 140°1′E) with fine gray lowland soil (Fluvisols) in Tsukuba-mirai, Ibaraki, Japan. The four FRCs were ‘Hoshiaoba’, ‘Leafstar’, ‘Hokuriku 193ʹ, and ‘Tachisuzuka’ (). The FRC fields received 80–120 kg N ha−1, 35 kg P ha−1, and 50 kg K ha−1 in each year from 2008 to 2015. From 2012 to 2018, the ERC ‘Koshihikari’ was cultivated in an adjacent paddy field, with fertilizer supplied at 60–80 kg N ha−1, 2–2.6 kg P ha−1, and 70 kg K ha−1 each year. From 2012 to 2018, an ERC was cultivated in an adjacent paddy field under the same management conditions as the FRCs except for the fertilization (Wakabayashi et al. Citation2016).
Table 1. Yield, potassium (K) and silicon (Si) uptake and balance (annual and average) in forage rice cultivars
2.2. Chemical analysis of soil and irrigation water
After the rice was harvested, soil samples which were 0–0.1 m depth from the ground surface were collected from the field sites for the measurement exchangeable K (1 M ammonium acetate extraction method), K extracted by hot 1 M nitric acid (Prat Citation1965), and available SiO2 (pH 6.2 phosphate buffer extraction method; Kato et al. Citation2002). Non-exchangeable K was calculated by subtracting the exchangeable K from K extracted with hot nitric acid (Surapaneni et al. Citation2002) (Appendix 1, 2).
We analyzed the chemical form of Al in soil by using a sequential extraction method based on adsorption strength between Al and organic matter (Dai et al. Citation2011). The Al fractions were extracted according to a 5-step sequential extraction (1 M KCl, 0.5 M CuCl2, 0.1 M sodium pyrophosphate, 0.2 M ammonium oxalate, and 0.5 M NaOH solutions) method. The concentrations of Al in their respective extracts (Appendix 3, 4) were determined by inductively coupled plasma atomic emission spectroscopy (ICP-AES; Optima 4300DV, Perkin Elmer, Waltham, MA, USA). Dissolved organic carbon contents of the sequentially extracted fractions were measured by using a dissolved organic carbon analyzer (TOC-L, Shimadzu, Kyoto, Japan).
To calculate the SiO2 and K balances, the available soil depth of the fields and the bulk density of the soil were assumed to be 0.1 m and 0.95 g cm−3, respectively. The K and Si concentrations in the irrigation water, which was collected several times from 2010 to 2015, were measured by ICP-AES. The annual volume of irrigation water was 15,000 Mg ha−1 (Tanji Citation1998). After the harvest, dried rice plants were ground and the resulting their powders were digested by mixing with nitrate and hydrogen peroxide (Oda Citation2004). K and Al in the digested solutions were measured by ICP-AES. The SiO2 contents of rice samples were measured by the dry ash method (Hokkaido Research Organization Citation2012). The uptake amounts of K, SiO2, and Al were determined from both the element content and dry matter weight (Plant Nutrition Experiment Method Editorial Committee Citation1990).
2.3. Measurement of organic acids exuded from plant roots
After wheat (Triticum L. ‘Haruyokoi’), maize (Zea mays L. ‘Goldrush’), rice (Oryza sativa L. ‘Kirara397ʹ), clover (Trifolium repens L. ‘Shirotsumekusa’), and tomato (Solanum lycopersicum L. ‘Sugar lump’) were cultivated in quartz sand for 4 weeks, they were transferred to 500 mL plastic containers (3 replicates) containing distilled water. They were hydroponically cultivated for another 2 weeks. Then, the roots were washed and soaked in 500 mL of distilled water for 3 days to collect the root exudates (Otani, Ae, and Tanaka Citation1996). The soaking solutions (20 mL) were collected and passed through a 0.2 μm cellulose acetate membrane filter. We used a strong cation exchange column (Bond Elut SCX, Varian Co. Ltd., Palo Alto, CA, USA) to separate the acidic fraction. The acidic fraction was eluted by 2.5 mL of 0.1 M hydrochloric acid to obtain a concentrated solution. The concentrated solution was dried by vacuum freeze-drying and dissolved in 50 μL of acetonitrile; then 50 μL of N, O-bis (trimethylsilyl)-trifluoroacetamide was added to the solution. The reaction was allowed to proceed for 30 minutes at 60°C to prepare trimethylsilyl derivatives. The organic acids in this reaction solution were quantified with a gas chromatography mass spectrometry system (GC2010 PARVUM2, Shimadzu Co. Ltd., Kyoto, Japan) equipped with a DB-5 column (length 30 cm, diameter 0.25 mm, Agilent, USA).
2.4. Contact reaction experiments
The FRC variety ‘Hokuriku 193ʹ was cultivated in the sand, and a sample root of cell walls was prepared according to Ae et al. (Citation1996). The roots which were cut into 5 mm long pieces were suspended in 0.1% sodium deoxycholate for 1 hour to remove intercellular components and plasma membranes. The collected root cell wall was washed several times with water and resuspended 0.5 M HCl for 0.5 hours. The suspension was placed on filter paper (No.3, Kiriyama Glass Works Co.) and washed with water, and rinsed with ethanol, and dried at 50 °C. Root cell walls of tomato variety ‘Aiko’ were also prepared by the same method. Then, 30 mg of root cell wall samples (5 mm in length) and 30 mg of powdered mineral or soil were added to a plastic test tube containing 5 mL of 10 mM sodium acetate buffer solution (pH 5.6). The test tube was shaken gently for 2 hours, and then the solution was filtered through a 0.22 µm mixed cellulose ester filter. The K, Si, and Al concentrations in the filtrate were measured by ICP-AES (iCap7400 Duo, Thermo Scientific, Waltham, MA, USA). Orthoclase, obsidian, kaolinite, and two types of gray lowland soil powder were used as test materials. In addition, root cell wall samples were soaked in a 0.1 M AlCl3 solution for 1 hour and then washed with water and rinsed with ethanol (‘Al-treated’). The weathering activity of Al-treated roots was also examined. The following minerals and soils were used for the experiment. Orthoclase (KCM Corporation, Nagoya, Japan) was powdered and sieved to the fine grain fraction (<64 μm). Obsidian collected from Wada Pass (36°8′N, 138°8′E), Nagano Pref., Japan, was powdered and sieved to the fine grain fraction (< 64 μm). Kaolinite powder (CP kaolin, Sigma-Aldrich Japan, Tokyo, Japan) was used for the contact reaction experiment. Two types of gray lowland soil (volcanic-origin and granitic-origin) were collected from the Tsukuba FRC test field and the Otsu FRC cropping field (35°0′N, 136°0′E), respectively. They were powdered and sieved to the fine grain fraction (<64 μm).
2.5. Statistical analysis
Student’s t-test was used to compare continuous Al and carbon contents in soil (). P-values less than 0.1 (P < 0.1) were considered statistically significant. All statistical analyses were performed using Kaleida Graph version 4.5 (HURINKS Inc., Tokyo, Japan).
Figure 1. Temporal changes in aluminum and organic carbon contents of extracts from soils by a 5-step sequential procedure. (a) Al contents extracted by 0.5 M CuCl2 solution (AlCu) and 0.1 M Na-pyrophosphate solution (Alp). Alp in FRC-soil (r = 0.73, P = 0.011, Increase rate (Δ) = 57 mg Al kg−1 year-1), AlCu in FRC-soil (r = 0.83 P = 0.002, Δ = 16 mg Al kg−1 year−1), and AlCu in ERC-soil (r = 0.88, P = 0.001, Δ = 13 mg Al kg-1 year-1) increased linearly with time. (b) Organic carbon content extracted by 0.1 M Na-pyrophosphate solution (Cp). Cp in FRC-soil (r = 0.65 P = 0.029, Δ = 73 mg C kg-1 year-1) increased linearly with time
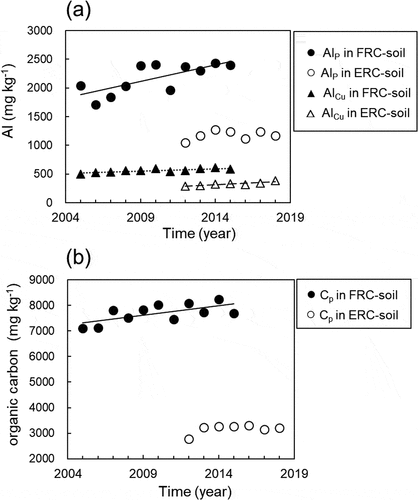
3. Results and discussion
3.1. K and Si balances in rice cultivation and Al and carbon accumulation in soil
Cultivation of FRCs in paddy fields from 2005 to 2015 produced average dry matter yields of 18,115 kg ha−1 (), higher than those produced by the ERC variety ‘Koshihikari’ (yield 12,600 kg ha−1, ). Potassium fertilizer application (36 kg K ha−1 year−1, ) to FRC was less than K uptake (208 kg K ha−1 year−1, ). The average amount of K absorbed by the FRCs and the ERC was 208 and 151 kg K ha−1 year−1, respectively (), and the average Si uptake of the FRCs and the ERC was 1531 and 1308 kg SiO2 ha−1 year−1, respectively. Thus, K and SiO2 absorption by FRCs is about 1.2 to 1.4 times the amounts absorbed by ERC.
Table 2. Yield, potassium (K) and silicon (Si) uptake and balance (annual and average) in edible rice cultivars
The potassium balances in the FRC paddy fields over the 11 years are shown in . Three sources of K were available to the rice plants, and the total supply over the 11 years was as follows: K fertilizer (400 kg K ha−1), irrigation water (440 kg K ha−1), and soil (770 kg K ha−1, exchangeable K). The total K supply was 1610 kg K ha−1, whereas K uptake by FRCs was 2284 kg K ha−1. The K balance in this field, therefore, was negative (−674 kg K ha−1, ). Thus, FRCs grew under apparently K-deficient conditions. Although K uptake by FRCs in the paddy field showed some annual variation (174–256 kg K ha−1year−1), it did not decrease with time, and the dry weight did not decrease during the 11 years of cultivation (16,093–22,097 kg ha−1). In the FRC paddy field, the concentration of exchangeable K and non-exchangeable K decreased from 109 to 55 mg kg−1 and from 138 to 117 mg kg−1 over the 11 years, respectively (Appendix 1). These results suggest that the rice was able to absorb K from the non-exchangeable K fraction in the soil.
The total SiO2 uptake by FRCs over the 11 years was 16,836 kg SiO2 ha−1, which was much greater than the total supply (5945 kg SiO2 ha−1) from the irrigation water (2563 kg SiO2 ha−1) and available SiO2 (3382 kg SiO2 ha−1) of the soil, resulting in a significantly negative SiO2 balance (−10,891 kg SiO2 ha−1, ). This negative SiO2 balance indicates that the rice needed more SiO2 than that of available form in the soil, similar to the K balance.
On the other hand, the soil in the ERC field was richer in available K than that of the FRC field (), and severe SiO2 deficiency was not observed in ERC fields compared with that in FRC fields. In the ERC paddy field, the non-exchangeable K content did not decrease over 7 years (Appendix 2).
Aluminum is the third most abundant element, following O and Si, in primary minerals. Aluminum uptake by the FRC and ERC rice plants was 2.9 and 3.4 kg Al ha−1 year−1, respectively, much less than the SiO2 uptake (1531 kg SiO2 ha−1 year−1, 1308 kg SiO2 ha−1 year−1). In general, the Al content of silicate minerals is high; for example, orthoclase (KAlSi3O8) contains 29 wt% Si, 14 wt% K, and 10 wt% Al. Therefore, when the Si and K derived from silicate minerals are taken up by rice plants, a large quantity of Al remains in the soil, especially in the rhizosphere. The Al released from minerals binds with organic matter such as plant and microbial debris in the rhizosphere. This form of soil carbon (called humic substances) is resistant to microbial degradation (Scheel, Dorfler, and Kalbitz Citation2007). In the FRC paddy field, the exchangeable Al extracted with a 1 M KCl solution (AlK) was 40 mg Al ha−1 year−1 or less, and no secular change was observed during the 11 years (Appendix 3). The aluminum extracted with 0.5 M CuCl2 solution (AlCu), corresponding to Al weakly bonded to the soil organic matter, increased year by year (16 mg Al kg−1 year−1, r = 0.83, P = 0.002; )). The Al extracted with 0.1 M Na–pyrophosphate solution (AlP), corresponding to Al strongly bonded to the soil organic matter (Dai et al. Citation2011), also increased significantly (r = 0.73, P = 0.011), at the rate of 57 mg Al kg−1year−1, in the FRC field over the 11 years ()). The organic carbon extracted with Na-pyrophosphate (Cp) from the FRC field increased (r = 0.65, P = 0.029) at 73 mg C kg−1 year−1 ()). The Cp of FRC soils increased with Alp, indicating carbon accumulation of 763 kg C ha−1 (i.e., 69.4 kg C ha−1 year−1) over the 11 years. Furthermore, we did not observe a significant increase in Alp in the ERC soils over the course of the experiment ()), although a slight increase in AlCu (r = 0.88, P = 0.001) was detected. In ERC soil with sufficient K, there was a slight accumulation of Al binding to the soil organic matter due to mineral weathering. By contrast, mineral weathering was strongly promoted by the absorption of K by the rice, along with large quantities of SiO2 in FRC paddy soil, the active Al remaining in the rhizosphere became bound to organic matter such as plant cells and soil microorganism debris. A schematic diagram of the processes associated with mineral weathering followed by silicate and K absorption by the rice plants is shown in ).
Figure 2. Schematic chemical reaction of weathering process of primary minerals by root surface cell wall (CW) of plants and nutritional K and Si (a), or only K (b) are taken up by the plants. Solubilized active Al reacts with organic compounds to form persistent soil storage carbon
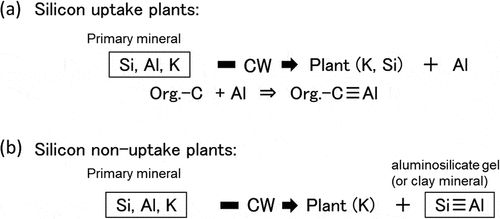
Soils with high carbon content (classified as Andosol) are widely distributed in volcanic regions. Soil carbon quantity is highly correlated with the abundance of plant opal, and many plants that can accumulate Si are members of the Poaceae family, including Miscanthus and Sasa in Japan, and Saccharum in Indonesia and the Philippines (Kato Citation1970; Kondo Citation1988; Cornelis et al. Citation2011). Soil carbon content can be increased by growing plants with the ability to absorb Si and form plant opal (phytoliths). Members of the Poaceae family are pioneer plants in volcanic ejecta. Therefore, the Si-absorbing plants may play a key role in the formation of Andosols because they can accumulate Al binding to the carbon of the plant residual itself. For aluminum to be active through its ability to promote mineral weathering by cell walls or organic acids, it is important that silicic acid is absorbed by the plant. The distribution situation of Andosol in the world is consistent with our proposed idea of soil carbon accumulation ()).
3.2. Mineral weathering by organic acids exuded by rice roots
Organic acids exuded from roots are known to act as chelating agents that promote mineral weathering (Ae et al. Citation1990; Badri and Vivanco Citation2009). Relative to other plants, however, rice roots exude a very small amount of organic acids (Otani, Ae, and Tanaka Citation1996; Ae, Shen, and Otani Citation2001). We examined organic acids exuded by five crops: wheat, maize, rice, clover, and tomato (). Oxalic acid, malonic acid, citric acid, malic acid, and tartaric acid were detected in the tomato root exudates. Of the five crops, the rice roots exuded the smallest quantity of organic acids. Groundnut roots also exude only small quantities of organic acids (Ae et al. Citation1996). However, groundnuts can grow in soil with low P content because their root cell walls have the ability to dissolve Fe- and Al-bound phosphate (phosphate minerals) through contact reactions (Ae et al. Citation1996). Thus, the ability of rice to absorb non-exchangeable K under conditions of K and Si deficiency could indicate that they have the ability to cause silicate mineral weathering through a contact reaction at the root surface.
Table 3. Organic acids exuded from the roots of 5 plant species
3.3. Mechanism of the release of K, Si, and Al from primary minerals
The soil pH (H2O) of the FRC field was 5.6 in the first year of the experiment and decreased to 5.4 after two years, it had kept at 5.3–5.4 for the next nine years. Thus, strong acidification of the soil was not observed. The decrease in pH in the rice rhizosphere is reported to be about 0.5 (Zeng et al. Citation2020) and the pH of irrigation water is 6.5–8.0 (Usui and Kasubuchi Citation2013), the contribution of water acidity to mineral dissolution is also small. In general, the weathering of primary minerals by water (based on mainly H+) takes a long time. For example, the Si dissolution rate of orthoclase is 1.67 × 10–12 mol m−2 s−1 (25°C, pH 5), thus, a sphere of orthoclase with a diameter of 1 mm would take 520,000 years to dissolve (Lasaga Citation1984). On the other hand, an increase in active Al (AlCu and Alp) was detected in field soils in which paddy rice had been cultivated for 11 years under low K and Si conditions (). Therefore, a change in dissolution rate due to a decrease in pH is not enough to explain the increase in the active Al content in the soils.
To investigate the role of rice root cell walls in the weathering of primary minerals, the FRC ‘Hokuriku 193ʹ was used for contact reaction experiments (). Cell walls of the roots of the tomato were prepared for comparison because tomato roots exuded the highest quantity of organic acids (). Powdered orthoclase, obsidian, kaolinite, and two types of gray lowland soil collected from the Tsukuba FRC cropping field (volcanic origin) and the Otsu FRC cropping field (granitic origin), were used for the contact reaction experiments. In addition, isolated root cell walls were soaked in AlCl3 solution (Al-treated), and their weathering activity was examined.
Table 4. Elements released (K, Si, Al) by “contact reactions” between plant roots and soils or minerals (orthoclase, obsidian, kaolinite)
shows the results of the reaction between minerals and tomato root cell walls, rice root cell walls, or Al-treated rice root cell walls. Tomato root cell walls did not dissolve K from the minerals or the two soils, but the rice root cell walls released 206 μg K g−1 from orthoclase, 283 μg K g−1 from obsidian, and 60 and 89 μg K g−1 from the volcanic-origin and granitic-origin paddy soils, respectively. However, the Al-treated rice root cell walls did not release K from orthoclase or obsidian, and they released a smaller quantity of K from the two soil powders than rice roots not treated with Al (). This inhibition of K release by Al-treated roots implies that strong chelation sites are present on the surface of rice root cell walls. Rice root cell walls contain pectin-derived carboxyl groups (-COOH), phenolic hydroxyl groups (-OH) such as ferulic acid, and alcoholic (-OH) groups. Arranged three-dimensionally on the surface of rice root cell walls, these functional groups can chelate ions with a small ionic radius and a 5–6 coordination structure such as Al and Fe. The weathering of silicate minerals by chelation compounds such as oxalic acid usually starts with Al leaching (Blum and Stillings Citation1995), and chelation sites on the cell wall behave similarly to oxalate. SiO4 tetrahedra with a high proportion of Si4+ ions are substituted by Al3+ in orthoclase and volcanic glass (including obsidian), allowing K+ to enter the lattice, resulting in a perfect local electrostatic charge balance. Since feldspar weathering occurs by the selective attack on the tetrahedral Al and the hydrolysis of the Al-O-Si (Blum Citation1994), it could be promoted by chelation of functional groups on the root surface. Consequently, K+ and Si tetrahedrons near Al in a silicate network can also be released from the mineral such as orthoclase or obsidian as K+ and H4SiO4 monomers (silicic acid).
Here, we observed the release of K from minerals and soils. Silicic acid, however, was only partially dissolved, because it could not exit from the silicate network or recombine with Al (as described later in this section). On the other hand, Si dissolution was not observed in the reaction between kaolinite and roots or Al-treated roots (). Since kaolinite had few Al isomorphous substitutions in the Si tetrahedral layer, Si-releasing from kaolinite could not be observed. The kaolinite did not decompose in the experiment, although the weathering of kaolinite could occur from the only edge surface Al (Cama and Ganor Citation2006). The slight leaching of K observed in the reaction between kaolinite and roots was considered to be an impurity, because the authigenic kaolinite does not contain K. These results suggest that the weathering of orthoclase and obsidian was efficiently promoted by a contact reaction between the mineral surfaces containing tetrahedral Al and the root cell wall surfaces.
The combination of mineral weathering by chelation and the high Si uptake leads to enrichment of the active Al in the rhizosphere. Most of the active Al was precipitated as amorphous hydroxyl Al in the paddy fields (pH 5–7). The amorphous hydroxyl Al is then combined with organic matter in the rhizosphere ()). Although minerals contained large amounts of Si and Al, only small amounts of Si and Al were detected relative to the amount of K released in the experiment. Because of using dead rice roots (with no capacity for Si uptake) in this experiment, the released Si and Al could have recombined and precipitated as aluminosilicate gel. If the plants could not absorb the Si released via the chelating ability of their roots, aluminosilicate gel or minerals would form in the rhizosphere ()). Hydroxyl Al can adsorb more organic matter than clay minerals because of its greater surface area (Brady and Weil Citation2008).
Assuming that K dissolved by root cell walls originates only from orthoclase (composition; K = 14 wt% and Al = 10 wt%) in granitic soil or volcanic glass (Wada Pass – obsidian composition; K = 4.7 wt% and Al = 6.5 wt%) in volcanic soil, the amount of Al supplied to the soil would be 0.7–1.4 times the quantity of solubilized K. Hence, 448–944 kg Al ha−1 (426–897 mg kg−1) must have been added to the FRC paddy fields by mineral weathering because of the negative K balance (674kg K ha−1) in the field (). This estimated value shows good agreement with the quantity of Alp (627mg kg−1; 57mg kg−1 × 11 years, ) obtained by sequential extraction.
After the death of rice root cells, Al on the root surface would be precipitated as hydroxyl Al bound to surrounding organic carbon derived from plants and microbial debris. The natural weathering rate of feldspar is reported to be 10 to 104 times faster than that of experimental water-induced rock weathering (Swobodacolberg and Drever Citation1993). This difference in weathering rates is reasonably explained by contact reactions between plant roots and mineral surfaces. Since the atomic structure of obsidian is disordered, obsidian is more easily weathered than orthoclase by the chelation ability of plant roots. Granitic and volcanic rocks contain also mica minerals (biotite, muscovite) that contain tetrahedral and octahedral Al, and K. Absorption of K from biotite and muscovite by upland rice has been confirmed (Sugiyama and Ae Citation2000; Akagi et al. Citation2006). Further investigation of chelating ability and chemical structure of rice root cell wall is needed.
4. Conclusions
We confirmed that stable soil organic carbon (Cp) combined with Al increased year by year in rice paddy soil under FRC cultivation. Under conditions of K and Si deficiency, FRC cultivation promoted weathering of primary silicate minerals including K, Si, and Al. However, the weathering of primary mineral and its relationship to K (or Si) deficiency of remains unclear. Although K deficiency eventually promotes Si accumulation in rice plants (Chen et al. Citation2016), it remains unknown how the deficiency affects the functions of root cell walls in rice plants, and further research is warranted. When K and Si were absorbed by FRCs, active Al remaining in the rhizosphere became bound to organic matter, forming stable soil carbon. Mineral weathering could begin with chelation at the surface of root cell walls. Chelation sites on the root cell wall might react with tetrahedral Al in primary minerals such as orthoclase and/or volcanic glass (obsidian). Soil carbon content can be increased by growing plants with the ability to absorb Si. These Si-absorbing plants may play a key role in the carbon accumulation of soils. Although the carbon accumulation rate (69.4 kg C ha−1 year−1) via mineral weathering by rice in paddy fields is about one-tenth that of coral (800 kg C ha−1 year−1, Yamano et al. Citation2002), the cultivation area of rice (167 × 106 ha, FAO Citation2017) is more than five times the area of coral reefs (28 × 106 ha, Burke et al. Citation2011). Thus, the carbon accumulation ability of rice is comparable to that of coral. Rice cultivation in paddy soil has huge potential to play an important role as a countermeasure against global warming based on carbon sequestration.
Acknowledgments
We thank Misses K. Ozawa, S. Matsuzono, M. Ogai, and Messrs. Y. Yoshida, and R. Monma for their help with sample preparation. We thank Drs. T. Ishikawa, S. Yada, and S. Wakabayashi for their assistance in the field.
Disclosure statement
No potential conflict of interest was reported by the authors.
Additional information
Funding
References
- Ae, N., J. Arihara, K. Okada, T. Yoshihara, and C. Johansen. 1990. “Phosphorus Uptake by Pigeon Pea and Its Role in Cropping Systems of the Indian Subcontinent.” Science 248 (4954): 477–480. doi:10.1126/science.248.4954.477.
- Ae, N., T. Otani, T. Makino, and J. Tazawa. 1996. “Role of Cell Wall of Groundnut Roots in Solubilizing Sparingly Soluble Phosphorus in Soil.” Plant and Soil 186 (2): 197–204. doi:10.1007/bf02415514.
- Ae, N., R. Shen, and T. Otani. 2001. “The Significance of the Root Cell Wall in Phosphorus Uptake.” In Plant Nutrient Acquisition, New Perspectives, edited by N. Ae, J. Arihara, K. Okada, and A. Srinivasan, 251–275. Tokyo: Springer.
- Akagi, T., S. Saito, S. Watanabe, M. Sugiyama, and N. Ae. 2006. “Selective Intake of Potassium from K-bearing Silicate Minerals by Sunflower and Upland Rice Inferred from Eu Anomaly: Implication for Weathering as a Direct Consequence of Plant Physiology.” Chikyukagaku (Geochemistry) 40: 1–12.
- Badri, D. V., and J. M. Vivanco. 2009. “Regulation and Function of Root Exudates.” Plant, Cell & Environment 32 (6): 666–681. doi:10.1111/j.1365-3040.2009.01926.x.
- Blum, A. E. 1994. “Feldspars in Weathering.” In Feldspars and Their Reactions, edited by I. Parsons, 595–630. Dordrecht: Springer.
- Blum, A. E., and L. L. Stillings. 1995. “Feldspar Dissolution Kinetics.” Chemical Weathering Rates of Silicate Minerals 31: 291–351.
- Brady, N. C., and R. R. Weil. 2008. The Nature and Properties of Soils, 94–313. 14th ed. New Jersey and Ohio: Pearson Prentice Hall.
- Burke, L., K. Reytar, M. Spalding., and A. Perry. 2011. “Reefs at Risk Revisited.” World Resources Institute. 116.
- Cama, J., and J. Ganor. 2006. “The Effects of Organic Acids on the Dissolution of Silicate Minerals: A Case Study of Oxalate Catalysis of Kaolinite Dissolution.” Geochimica Et Cosmochimica Acta 70 (9): 2191–2209. doi:10.1016/j.gca.2006.01.028.
- Chen, D. Q., B. B. Cao, S. W. Wang, P. Liu, X. P. Deng, L. N. Yin, and S. Q. Zhang. 2016. “Silicon Moderated the K Deficiency by Improving the Plant-water Status in Sorghum.” Scientific Reports 6. doi:10.1038/srep22882.
- Cornelis, J. T., B. Delvaux, R. B. Georg, Y. Lucas, J. Ranger, and S. Opfergelt. 2011. “Tracing the Origin of Dissolved Silicon Transferred from Various Soil-plant Systems Towards Rivers: A Review.” Biogeosciences 8 (1): 89–112. doi:10.5194/bg-8-89-2011.
- Dai, Q. X., N. Ae, T. Suzuki, M. Rajkumar, S. Fukunaga, and N. Fujitake. 2011. “Assessment of Potentially Reactive Pools of Aluminum in Andisols Using a Five-step Sequential Extraction Procedure.” Soil Sci. Plant Nut. 57 (4): 500–507. doi:10.1080/00380768.2011.598445.
- Food and Agriculture Organization of the United Nations (FAO), 2017. FAOSTAT: Crops, http://www.fao.org/faostat/en/#data/QC
- Gulde, S., H. Chung, W. Amelung, C. Chang, and J. Six. 2008. “Soil Carbon Saturation Controls Labile and Stable Carbon Pool Dynamics.” Soil Science Society of America Journal 72 (3): 605–612. doi:10.2136/sssaj2007.0251.
- Hobara, S., S. Fukunaga-Yoshida, T. Suzuki, S. Matsumoto, T. Matoh, and N. Ae. 2016. “Plant Silicon Uptake Increases Active Aluminum Minerals in Root-zone Soil: Implications for Plant Influence on Soil Carbon.” Geoderma 279: 45–52. doi:10.1016/j.geoderma.2016.05.024.
- Hokkaido Research Organization, 2012. “Plant Nutrition. Analytical Methods for Soil and Crop Nutrition Diagnosis.” 117–152.
- Kato, N., K. Kumagai, F. Nakagawa, and H. Sumida. 2002. “Comparison of Three Methods for Evaluation of Available Silicon in Paddy Soils.” Proceedings of the Second Silicon in Agriculture Conference, Tsuruoka, Japan, August. 22-26.
- Kato, Y. 1970. “Soil Distribution, Cross-sectional Morphology and Parent Material of Andosol in Tokai Region.” The Journal of Soil Science and Plan 41 (3): 89–94. in Japanese.
- Kato, Y. 1978. “Generation and Classification of Andosol.” The Japanese Society of Irrigation, Drainage and Reclamation Engineering 46 (12): 869–876. in Japanese.
- Kondo, R. 1988. “Opal Phytolith Analysis of Andisols with Regard to Interpretation of Paleo Vegetation.” Proceeding 9th International Soil Classification Workshop, Properties, Classification, and Utilization of Andisols and Paddy Soils, Japan, 520–534.
- Lasaga, A. C. 1984. “Chemical Kinetics of Water‐rock Interactions.” Journal of Geophysical Research: Solid Earth 89 (B6): 4009–4025. doi:10.1029/JB089iB06p04009.
- Ma, J. F., K. Tamai, M. Ichii, and G. F. Wu. 2002. “A Rice Mutant Defective in Si Uptake.” Plant Physiology 130 (4): 2111–2117. doi:10.1104/pp.010348.
- Oda, H. 2004. “Uptake and Transport of Cd Supplied at Different Growth Stages in Hydroponically Cultured Soybean Plants.” Biomedical Research on Trace Elements 15 (3): 289–291.
- Ogawa, K., T. Kuwana, and A. Ushio. 2004. “Effect of Long-term (50 Years) Application of Rice Straw Compost and Three Fertilizer Elements (NPK) on the Yield of Rice and Wheat. (In Japanese).” Kinki Chugoku Shikoku Agricultural Research 5: 3–9.
- Otani, T., N. Ae, and H. Tanaka. 1996. “Phosphorus (P) Uptake Mechanisms of Crops Grown in Soils with Low P Status .2. Significance of Organic Acids in Root Exudates of Pigeon Pea.” Soil Sci. Plant Nutr. 42 (3): 553–560. doi:10.1080/00380768.1996.10416324.
- Plant Nutrition Experiment Method Editorial Committee. 1990. “Inorganic Component Analysis.” In Plant Nutrition Experiment Method, 114–162. Japan, Tokyo: Hakuhodo.
- Prat, P. F. 1965. “Digestion with Hydrofluoric and Perchloric Acid for Total Potassium and Sodium.” Methods of Soil Analysis, Agronomy 9: 1019–1021.
- Scheel, T., C. Dorfler, and K. Kalbitz. 2007. “Precipitation of Dissolved Organic Matter by Aluminum Stabilizes Carbon in Acidic Forest Soils.” Soil Science Society of America Journal 71 (1): 64–74. doi:10.2136/sssaj2006.0111.
- Shiota, Y., K. Sano, and I. Okimura. 1984. “Short and Long-term Effect of Successive Application of Rice Straw Compost Evaluated by Nitrogen Uptake by Rice Plants.” Research Bulletin of the Aichi-ken Agricultural Research Center 16: 43–51.
- Sugiyama, M., and N. Ae. 2000. “Uptake Response of Crops to Potassium from Andosol and Potassium−Bearing Minerals Applied to Andosol.” The Journal of Soil Science and Plant Nutrition Nutr.,71: 786–793.
- Surapaneni, A., R. W. Tillman, J. H. Kirkman, P. E. H. Gregg, and A. H. C. Roberts. 2002. “Potassium-supplying Power of Selected Pallic Soils of New Zealand 2. Soil Testing Procedures.” New Zealand Journal of Agricultural Research 45 (2): 123–128. doi:10.1080/00288233.2002.9513501.
- Swobodacolberg, N. G., and J. I. Drever. 1993. “Mineral Dissolution Rates in Plot-scale Field and Laboratory Experiments.” Chemical Geology 105 (1–3): 51–69. doi:10.1016/0009-2541(93)90118-3.
- Takahashi, E. 1974. “Effect of Soil Moisture on the Uptake of Silica by Rice Plant Seedlings.” The Journal of Soil Science and Plant Nutrition 45: 591–596.
- Takahashi, T., and S. Shoji. 2002. “Distribution and Classification of Volcanic Ash Soils.” Global Environmental Research-english Edition 6 (2): 83–98.
- Tanji, H. 1998. “Demand Estimation of Irrigation Water of Japan in the 21th Century.” J. Japan Soc. Hydrol. and Water Resour. 11: 757–767. doi:10.3178/jjshwr.11.757.
- Usui, Y., and T. Kasubuchi. 2013. “Diurnal Variation in CO2, Dissolved Oxygen (DO), pH and RpH and Their Correlations in Ponded Paddy Field Water.” Japanese J. Limnology 74: 15–20. doi:10.3739/rikusui.74.15.
- Wakabayashi, S., S. Itoh, N. Kihou, H. Matsunami, M. Hachinohe, S. Hamamatsu, and S. Takahashi. 2016. “Influence of Water Management and Fertilizer Application on 137Cs and 133Cs Uptake in Paddy Rice Fields.” Journal of Environmental Radioactivity 157: 102–112. doi:10.1016/j.jenvrad.2016.03.010.
- Wei, Z. Q., J. H. Ji, Z. Li, and X. Yan. 2017. “Changes in Organic Carbon Content and Its Physical and Chemical Distribution in Paddy SoilsCultivated under Different Fertilization Practices.” Journal of Soils and Sediments 17 (8): 2011–2018. doi:10.1007/s11368-017-1676-6.
- Yamano, H., H. Kayanne, F. Matsuda, and Y. Tsuji. 2002. “Lagoonal Facies, Ages, and Sedimentation in Three Atolls in the Pacific.” Marine Geology 185 (3–4): 233–247. doi:10.1016/s0025-3227(02)00188-3.
- Zeng, T., M. A. Khaliq, H. Li, P. Jayasuriya, J. Guo, Y. Li, and G. Wang. 2020. “Assessment of Cd Availability in Rice Cultivation (Oryza Sativa): Effects of Amendments and the Spatiotemporal Chemical Changes in the Rhizosphere and Bulk Soil.” Ecotoxicology and Environmental Safety 196: 110490. doi:10.1016/j.ecoenv.2020.110490.
Appendixes
Appendix 1. Exchangeable potassium (K) and non-exchangeable K contents in FRC-soils
Appendix 2. Exchangeable potassium (K) and non-exchangeable K contents in ERC- soils
Appendix 3. Extractable aluminum contents by 5-sequential extraction in FRC- soils
Appendix 4. Extractable aluminum contents by 5-sequential extraction in ERC- soils