ABSTRACT
Paddy soils are the dominant source of nitrogen for rice via mineralization, and knowledge about the available nitrogen (AN) in paddy soil is important for rice cultivation. Although the relationship between AN and soil physicochemical parameters has been studied, no systematic studies of the relationships between AN and the soil moisture content of various soil types in Japan have been studied. Therefore, we examined the impacts of soil types and treatment of organic matter application or paddy–upland rotation on these relationships. We sampled topsoils from 30 paddy fields throughout Japan. We compared the relationships between AN and soil physicochemical properties, such as total nitrogen (TN), cation exchange capacity, soil water-holding capacity (WHC), mass water content after harvest (MWH), and water content after harvest (WCH). The scatter diagram revealed that AN and either MWH or WCH (p < 0.01) had the highest positive linear correlation coefficient; the pattern became clearer after separating Andosols and non-Andosols. We analyzed the relationship between AN and either TN or MWH in 100 topsoils, including soils from treatment plots with successive organic matter application or paddy-upland rotation. A significantly positive correlation between AN and TN was observed, although the regression slopes for volcanic or non-volcanic soils differed. The decrease in AN due to frequent paddy–upland rotation was greater than that for TN. On the other hand, a highly positive correlation between AN and MWH was observed, and the coefficient of determination was higher than that for AN and TN. TN and the WHC increased when the accumulation of organic matter in paddy soil was greater. As there was a positive correlation between WHC and MWH, a positive correlation was also observed between AN and MWH. Additionally, we investigated year-to-year variation in MWH using topsoil samples collected before autumn tillage, for 3–5 consecutive years in 24 plots, for various soil types throughout Japan. In order to eliminate the effects of precipitation and to minimize the fluctuation of MWH in paddy topsoil, it was desirable to collect topsoil samples within 24–96 hours of the last precipitation of 1 mm h−1 or more, for the measurement of moisture content.
1. Introduction
Paddy rice (Oryza sativa L.) utilizes the nitrogen (N) mineralized in paddy soils at a high rate. Hirokawa, Ito, and Kitagawa (Citation1993) estimated the utilization rate of this N to be approximately 65% in Gray Lowland soil, while Fujii et al. (Citation1989) reported an average N utilization rate of 85% in the soils of the Yamagata Prefecture, which were mainly of heavy texture. These findings indicate that soil N fertility is essential. A standard parameter for evaluating soil N fertility is the available nitrogen (AN), which is an estimate for the amount of N mineralized from paddy soil, and which reflects the potential N supply for rice. The AN value is calculated as the amount of N mineralized from air-dried paddy soil that is incubated at 30°C for 4 weeks under submerged conditions. The Ministry of Agriculture, Forestry and Fisheries (Citation2008) set a target of 80–200 mg kg−1 AN, which is used as an indicator of improved fertilizer applications in some Japanese prefectures (Kitada Citation1990; Ueno et al. Citation1992). AN has been found to vary with region and organic matter applications. Furthermore, the temperature, soil type, and drainage in paddy fields affects the decomposition of organic matter, which is a source of AN in various regions (Konno and Sugihara Citation1986; Shibahara and Inubushi Citation1997; Shiratori et al. Citation2007). The Miyagi Prefectural Agricultural Research Center (Citation1992) reported that the relationship between AN and total carbon (TC) in Andosols differs from that in other soils because the organic matter content of Andosols is considerably higher. Kubota (Citation1992) reported that the decomposition rate of rice straw (RS) in semi-poorly drained paddy fields was lower than that in well-drained paddy fields. As drainage conditions affect the decomposition of organic matter, the source of AN, the drainage of paddy fields can affect AN levels. Increases in AN due to the application of organic matter, such as compost, have been found to be similar to the increases in TC and total N (TN) relative to levels in control plots treated with standard fertilizers (Wada et al. Citation1981; Azuma, Takahashi, and Kato Citation2015). Takakai et al. (Citation2010) indicated that in fields subjected to paddy–upland rotation, the annual budget for N was negative (−10.0 g N m−2 year−1), in an upland soybean (Glycine max) field in the Akita Prefecture that had been converted from being a rice paddy with Gray Lowland soil. Furthermore, in the Tohoku region, Toyama Prefecture, and Fukuoka Prefecture, frequent rotations have led to a decrease in AN, with the rate of AN decrease being higher than that of TN (Sumida, Kato, and Nishida Citation2005; Nishida, Sekiya, and Yoshida Citation2013; Hirokawa, Inahara, and Koike Citation2011; Odahara et al. Citation2012).
Azuma, Takahashi, and Kato (Citation2015) used regression plots to demonstrate the relationship between an increase in AN after an application of organic matter treatment relative to control plots, and the decrease in AN caused by frequent paddy–upland rotation relative to the control plots. The results of the regression analysis indicated that changes in AN were closely correlated with changes in water content of the soil after harvest (WCH).
In the current study, moisture content or water retentivity were defined and calculated as follows:
The mass water content after harvest (MWH) is the ratio of the weight of water in field moist soil collected after harvest to that of oven-dried soil.
MWH = Weight of water evaporated (g)/Weight of oven-dried soil (g)
(b) Water content after harvest (WCH) is the amount of water in field moist soil.
WCH = Weight of water evaporated (g)/Weight of field moist soil (g)
(c) Soil water-holding capacity (WHC) is the amount of water that can be retained in air-dried soil. WHC = (Weight of water added into the air-dried soil (g) – Weight of water that cannot be retained in air-dried soil (g))/Weight of oven-dried soil (g)
TN and cation exchange capacity (CEC) reflect soil fertility, as mentioned above; there are many studies on the relationship between AN and these physicochemical soil parameters. Although MWH, WCH, and WHC are direct reflections of the soil moisture conditions in paddy soils, there has been no systematic study of the relationships between AN and the soil moisture content of various soil types in Japan. Kobo and Oba (Citation1973) investigated the maximum water capacity of soils of volcanic ash origin collected from uncultivated land throughout Japan and demonstrated that the maximum water capacity of soils was generally high. Therefore, the relationships between AN and physicochemical properties in volcanic soils were assumed to be different from those of other soil types. In the current study, we investigated the relationship between AN and physicochemical properties, and examined the impact of soil type and treatment of organic matter application or paddy–upland rotation on these relationships, in paddy fields throughout Japan. The samples are the same as those described in our previous report, from which the WCH, TN, TC, and AN values are quoted (Azuma, Takahashi, and Kato Citation2015).
Additionally, we investigated year-to-year variations in MWH across 24 sampled soils after harvesting for 3–5 consecutive years to examine the stability of MWH.
2. Materials and methods
2.1. Soil samples and incubation methods to measure the available nitrogen
From September 2012 to October 2012, after the crops had been harvested, we collected samples of topsoil from 100 paddy fields with known cultivation histories, from 29 public agricultural research centers and two farmers’ fields in 23 prefectures, from Iwate to Kagoshima (, Tables S1 and S2). For each sample, approximately 3–4 kg of soil was uniformly collected from the topsoil of the central part of each field. Soils were divided into groups for MWH analysis or air-drying. The soil samples used for MWH analysis were stored in sliding zipper bags (Ziploc, Asahi Kasei) and kept at 5°C until analysis. The soil types of the 31 sites included 16 Gray Lowland soils (including one of Shirasu soil, a type of porous volcanic soil found in Kagoshima, in the southernmost part of Kyushu, Japan), 10 Gley soils, four Andosols, and one Yellow soil. Counts of soil textures (determined by the method of the International Society of Soil Science; ISSS) were light clay (LiC), 13; silty clay (SiC), one; clay loam (CL), seven; loam (L), one; silty loam (SiL), one; sandy clay loam (SCL), four; and sandy loam (SL), four. The recent cultivation histories of the 100 fields were 89 sites with continuous use for paddy rice; two sites using paddy (rice)–upland (soybean) rotation, with soybean cropping once every 2–3 years; four sites with double cropping of paddy rice and cabbage (Brassica oleracea L.); and five sites with double cropping of paddy rice and wheat (Triticum L.). The municipality was indicated for each test site. The incorporation of RS was reported at 45 sites (including RS compost, RSC), and the application of farmyard manure (excluding RSC) was reported at 37 sites. Amendments included stubble and root residue from previous crops. The application of organic matter had been performed for several years, and the number of application years is presented in Tables S1 and S2. All soils were sampled after the harvesting of paddy rice, and soils from multiple fields at a single research center were sampled on the same day.
Figure 1. Locations of 31 soil sampling sites.
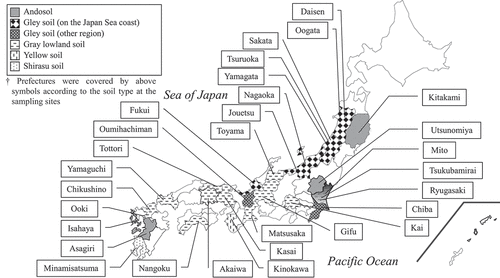
To obtain air-dried samples, the soil samples were placed in a drying oven at 40°C, and moisture was reduced to a level at which soils could be crushed. Subsequently, samples were passed through a 2 mm sieve, and the sieved sample was kept in the drying oven at 40°C for another 24 hour (h) to obtain air-dried soils.
Soil incubation under submerged conditions used a subsample (15 g) of air-dried soil packed in a glass test tube (30 mm diameter of and 120 mm height). Distilled water (40–50 mL) was added, and the suspension was stirred until no clouds remained. Water was added until the air layers in the test tube disappeared. The test tube was then sealed with a butyl rubber stopper and was incubated for 4 weeks at 30°C. Samples were transferred to 250 mL shaker bottles and 100 mL 10% (w/v) KCl solution was added, according to the method described by Toriyama (Citation1994). After shaking for 30 min, the suspension was filtered, and the ammonia-N content in the sample was measured using an Auto-Analyzer (QuAAtro 2-HR; BL-TEC). The AN was calculated by subtracting the latter measurements from the amount of ammonia-N in the soils before incubation. Two independent replicates were created for each incubation experiment.
To study temporal fluctuations, we measured the MWH at the same sites for several years. Soil samples were collected from nine locations at the Toyama Prefectural Agricultural Research Institute and at the growth observation field for rice in Toyama Prefecture, for 3–5 years (2015–2019). The soil types found at the sites were three Gray Lowland soils, two Gley soils, two Andosols, and two Gley Upland soils. Counts of soil textures were SL, two; L, two; CL, three; and LiC, two. The durations over which samples were collected were shortened at four sites (Kamikuzuro, Hironoshin, Kume, and Yatsuomachi-Shinden) because these sites were not used for the survey to document rice growth (). Additionally, to study year-to-year variation in MWH in other regions, soil samples were collected at five sites (Ogata, Yamagata, Joetsu, Ryugasaki, and Gifu) in the autumns of 2011, 2012, and 2013. The sampling dates for the nine sites in Toyama Prefecture are listed in , and those for the five sites in other regions in .
Table 1. Test site (location), soil classification, soil texture, sampling year, total nitrogen of soil (TN), total carbon of soil (TC), available nitrogen of soil (AN), and Nearby AMeDAS in Toyama prefecture
Table 2. Mass water content after harvest of soil (MWH), sampling day, and situation of precipitation at each sites in 3–5 years in Toyama prefecture
Table 3. Mass water content after harvest of soil (MWH), sampling day, and situation of precipitation at each sites in 3 years throughout Japan
2.2. Analytical methods
The MWH and WCH were determined by weighing 20 g of the field-moist soil in a pre-weighed beaker, then drying the soil at 105°C for 48 h, then reweighing the beaker. The WCH was calculated by dividing the weight of water evaporated by the weight of field-moist soil. The MWH was calculated by dividing the weight of the evaporated water by the weight of oven dried soil. The TN and TC were measured using an Automatic High-Sensitivity NC Analyzer (SUMIGRAPH NCH-22 F; Sumika Chemical Analysis Service). The particle-size distribution was determined using the pipette method, and the CEC was measured using the Schollenberger method, as described in Dojou Kankyou Bunseki (Citation1997). The soil WHC was measured following the method described by the Food and Agricultural Materials Inspection Center (FAMIC) (Citation1984), with minor modifications. A filter paper disk (ADVANTEC TOYO No. 5B) was placed in a funnel, and 100 g of air-dried soil was added into the filter. Distilled water (200 mL) was passed through the soil and filter, and the water that passed through was collected and weighed to obtain the amount of water absorbed by the filter paper and the weight of the water retained by the air-dried soil. Then, the WHC was calculated as the difference between the amount of water poured in and the amount that passed through. In the original method, 100 mL water was added to soil; however, this amount was insufficient for Andosols. For consistency, 200 mL of water was added to the measurements of all soil types, in calculations of WHC. Furthermore, the original method called for drying soils at 100°C for 5 h, but to avoid soil denaturation, we used air-dried soil.
2.3. Statistical analysis
All analyses were conducted using Microsoft Excel 2016 for Windows (Microsoft Corp., Tokyo, Japan) and Excel-Toukei ver. 6.0 (ESUMI Co., Ltd., Tokyo, Japan). Correlation analyses were conducted on AN and physicochemical properties. A significant difference test was performed on the slopes of different regression lines, for each comparison. A two-way analysis of variance was conducted, followed by Tukey’s test, to assess the differences in MWH among treatments. A p-value of <0.05 was considered to be statistically significant.
3. Results
3.1. Relationship between AN and CEC, WHC, TN, MWH, and WCH
We investigated the relationship between AN and other soil physicochemical properties in 30 control plots cultivated with standard amounts of CF. Plots were categorized into four groups: Gley soil, Andosol, Shirasu soil, and Other soils (). The range of AN in the control plots was 19–239 mg kg−1, whereas the AN in Gley soils on the coast of the Sea of Japan (Tsuruoka, Nagaoka, Jouetsu, and Fukui) and Andosols in Asagiri exceeded 200 mg kg−1 (Table S2).
Figure 2. Relationships between available nitrogen of soil (AN) and physicochemical properties of soil in control plots. a) Cation exchange capacity vs AN, b) Soil water-holding capacity vs AN (Total n = 30; Gley soil, n = 9; Andosol, n = 4; Shirasu, n = 1; Others (Gray Lowland soil and Yellow Soil), n = 16).
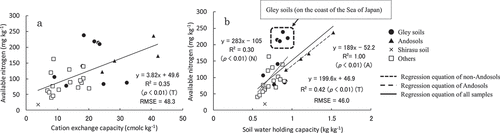
Figure 3. Relationships between available nitrogen of soil (AN) and physicochemical properties of soil in control plots. a) Total nitrogen vs AN, b) Mass water content after harvest vs AN (Total n = 30; Gley soil, n = 9; Andosol, n = 4; Shirasu, n = 1; Others (Gray Lowland soil and Yellow Soil), n = 16).
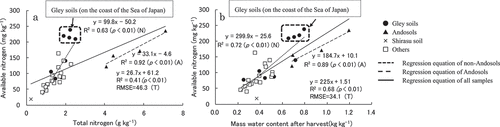
In the control plots, the range of CEC values was 3.7–43.0 cmolc kg−1, whereas in Shirasu soils, the values of CEC were relatively low, while the values of Gley soils and Andosols were relatively high (Tables S1 and S2). A significantly positive correlation between AN and CEC (, p < 0.01) was observed, but its coefficient of determination (R2) was lower than that for the relationship between AN and other soil physicochemical properties. Thus, the influence of soil type on the relationship between AN and CEC was unclear. In particular, the data points of the Gley soils were scattered all across the plotted diagrams.
The WHC for the control plots ranged from 0.57 to 1.52 kg kg−1, with values less than 1.0 kg kg−1 for all soils except Andosols (Tables S1 and S2). A significant positive correlation between WHC and AN (p < 0.01) was observed, but the plotted values for the Gley soils from the coast of the Sea of Japan were positioned above the regression line in .
The range of TN in the control plots was 0.23–7.10 g kg−1, with an extremely high TN, 4.1 g kg−1 and above, in all Andosols (Tables S1 and S2). A significantly positive correlation was observed between AN and TN (, p < 0.01). However, Gley soils on the coast of the Sea of Japan and Andosols showed different relationships from those of other soils (, scatter diagram). Plots for Andosols were distributed below the overall regression line, whereas plots for Gley soils on the coast of the Sea of Japan were mainly distributed above the regression line.
The MWH of the control plots was within the range of 0.22–1.29 kg kg−1 (Tables S1 and S2), and most of the MWH of the Gley soils and Andosols were 0.5 kg kg−1 or more. The WCH of the control plots was within the range of 0.19–0.54 kg kg−1 (Tables S1 and S2). The values for Andosols (0.41–0.54 kg kg−1) were the highest, followed by Gley soils (0.20–0.45 kg kg−1) and others, the latter mainly including Gray Lowland soils (0.19–0.34 kg kg−1). A significant positive correlation between AN and MWH was observed. The R2 values were as high as 0.68 (, p < 0.01), and the corresponding root mean square error (RMSE) was low (). The slope of the regression lines depicting the relationship between AN and MWH was smaller for the Andosols than that for other soils (excluding Shirasu soil). In Shirasu soil, AN was the lowest among the soils tested, but MWH was 0.37 kg kg−1, and the deviations from the regression line were relatively large.
On the other hand, a significant positive correlation between AN and WCH was observed (Fig. S1, p < 0.01). The slope of the regression lines depicting these relationships was not statistically different between Andosols and other soils (excluding Shirasu soil) (p > 0.1), although the intercepts were different (p < 0.01).
3.2. The influence of organic matter application and paddy–upland rotation on the relationship between AN and TN, MWH, and WCH
The relationships between AN and either TN or MWH are shown in ; 100 samples were used, including those for organic matter applications and paddy–upland rotation. The Chiba soils were contaminated by the surrounding Andosols (personal communication), and Shirasu soils were derived from pyroclastic flow so both were classified as volcanic soil. In the results described below, the correlations for non-volcanic soils (n = 79) and volcanic soil (n = 21) were examined independently of each other. AN increased with the application of organic matter; in particular, the AN of the Gley soils plots on the coast of the Sea of Japan with organic matter applications greatly exceeded 200 mg kg−1 (Table S2). Whether the soil was volcanic or not, with the application of organic matter, AN increased with increasing TN (). The tendency was the same as shown by the comparison with control plots, which had been fertilized only CF (). On the other hand, for the paddy–upland rotation fields in Nagaoka, the rate of decrease in AN due the frequency of soybean cultivation was larger than that of TN, and the AN/TN in the field where soybeans were planted once every 2 years was about half that of the continuously cropped paddy rice plot (Table S2).
Figure 4. Influence of organic matter application (OMA), and paddy-upland rotation on the relationships between available nitrogen of soil (AN) and physicochemical properties of soil, a) total nitrogen vs AN, b) mass water content after harvest vs AN.
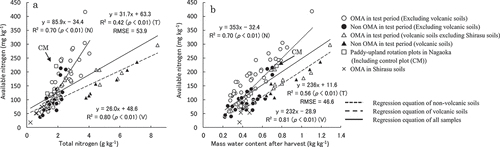
A significantly positive correlation between AN and MWH was observed (, p < 0.01). The slope of the regression line for non-volcanic soil was larger than the slope of the regression line for volcanic soil (p < 0.01), and the intercepts of the regression lines for each soil type were similar.
A significantly positive correlation between AN and WCH was observed (Fig. S2, p < 0.01). The distribution of volcanic soil and non-volcanic soil on the relationship between AN and WCH was similar to that of the only-CF plot (Fig. S1).
3.3. Year-to-year variation of MWH
To examine temporal fluctuations, we investigated the MWH at nine sites in Toyama Prefecture and five sites in the other areas. In Toyama Prefecture, the range of year-to-year variation in MWH was 0.457–0.686 kg kg−1 in Gray Lowland soil, 0.625–0.847 kg kg−1 in Gley soil, 0.866–1.132 kg kg−1 in Andosol, and 0.682–0.946 kg kg−1 in Gley Upland soil (). In the other Prefecture sites, the range of MWH in Gray Lowland soil and Gley soil was 0.297–0.571 kg kg−1 and 0.504–0.881 kg kg−1, respectively (). In the control plots in which only CF was applied, the MWH was significantly different between Gray Lowland soil and Gley soil (p < 0.01; data not shown). A significant difference was also observed between plots with non-organic matter application and organic matter application at each site, except for the RS plot in Gifu (p < 0.05).
3.3.1. Effect of continuous no-precipitation days on MWH
In Gray Lowland soil in Toyama Prefecture, the MWH values measured for the samples from Yoshioka examined in 2017, Nunoichi in 2015, and Urayama in 2015 were 7% lower or more, than the average for 5 years, because a continuous lack of precipitation lasted more than 3 days before soil sampling. In addition, the total precipitation was less than 50 mm week−1 before sampling (). In the Andosol sample from Takeuchi examined in 2017, in the case with a continuous lack of precipitation lasting 4 days prior to soil sampling, the MWH was 13% lower than the five-year average. Similarly, the MWH of Gray Lowland soils in other regions of Japan were 4–14% lower than the three-year averages for Yamagata in 2013, Ryugasaki in 2011, and Gifu in 2012 (). In all cases, the lack of precipitation lasted 4 days or more. The decreases in the MWH of plots with organic matter applications were larger than those for the control plots at each location.
A negative correlation was observed in the nine soils collected from Toyama Prefecture between continuous no-precipitation days before sampling and a relative MWH value for the average MWH at each site (the ratio of MWH in each year to the average value of MWH at the overall experimental duration). The same tendency was observed among all 15 sampling locations across Japan (, p < 0.01).
Figure 5. Relationship between the accumulation of continuous no-precipitation days before sampling and rate against average in mass water content after harvest (MWH) in each site.
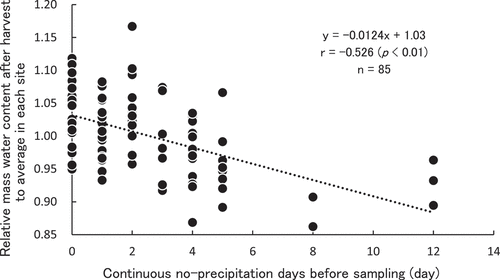
3.3.2. Effect of precipitation before soil sampling on MWH
The MWH values of Gray Lowland soils were 5% higher or more, than the five-year average, when precipitation of 1 mm or more occurred within 24 h or when precipitation accumulation was 100 mm week−1 or more, before soil sampling at Nunoichi in 2018 and 2019 and Urayama in 2016 and 2019. In 2018, the MWH at Hamakurosaki was 6% higher than the five-year average when the total precipitation during the week before soil sampling was as high as 98.5 mm. The MWH in Andosol was 5% higher or more, than the five-year average for Takeuchi in 2018 and 2019, when precipitation of 1 mm or more occurred within 24 h before soil sampling (). The MWH at Ryugasaki and Jouetsu in 2013 tended to be higher than the three-year average when higher precipitation (more than 90 mm week−1) was reported for the week prior to soil sampling ().
At Kamikuzuro in 2015, when combined harvesting was delayed due to poor drainage before the harvest, MWH was higher than in other years. In Oogata, 362.5 mm of precipitation was recorded in September 2011 before the harvest, and fields did not dry out the harvest season so combine-harvesting was not performed that year. As a result, the MWH of all plots at Oogata was higher in 2011 than in the two other years ().
4. Discussion
4.1. Relationship between AN and CEC, WHC, TN, and MWH in paddy fields
To examine the relationship between AN and other soil physicochemical parameters, we compared the distribution of data points on scatter diagrams of the corresponding measurements for different soil types. A significantly positive linear correlation between AN and CEC () was observed. Shibahara and Inubushi (Citation1997) reported a similar correlation between AN and CEC from 23 samples. However, R2 was the lowest among the relationships tested between AN and the soil physicochemical properties examined (). It may be difficult to convert measurements of CEC into AN because CEC is strongly influenced by parent materials, particularly clay types (Harada Citation1984).
In contrast, no decrease in CEC was observed in paddy–upland (soybean) rotation fields, compared with continuously cropped paddy rice fields (Table S2). Kamisato (Citation1958) and Takahashi, Sunaga, and Kanke (Citation1984) also reported that the decreases in CEC for the paddy–upland rotation scheme were small.
The AN and WHC were significantly correlated (p < 0.01), but data points from plots with Gley soils on the coast of the Sea of Japan were distributed above the regression line in the scatter diagram (). In addition, the slopes of the regression lines of Andosols and non-Andosols were different. Kobo and Oba (Citation1973) indicated that the maximum water capacity of soils originating from volcanic ash collected from uncultivated land throughout Japan was generally high, and these soils accumulated a substantial amount of organic matter. Therefore, we considered that these relationships between AN and WHC were affected mainly by the difference in mineralization of organic matter between Andosols and other soils.
A significantly positive linear correlation between AN and TN () was observed. Thus, the source of AN may represent organic matter that decomposes easily. AN tended to accumulate linearly as TN increased, in the soil samples that were collected throughout Japan. Plotted data for Andosols were distributed under the overall regression line (). Shoji and Takahashi (Citation2002) indicated that in northeastern Japan, the percentage of mineralizable N (the N mineralization potentially divided by the pool of total organic N) in upland fields with volcanic soils was less than half that of non-volcanic soils. Kobo and Oba (Citation1974) indicated that the organic matter in uncultivated Andosols was bound to allophane and Al3+. Even though the TN in Andosols was higher than that in the other soils, the N available for mineralization was low.
Contrarily, the data points from Gley soils on the coast of the Sea of Japan were positioned above the AN/TN regression line for all samples (). Shibahara and Inubushi (Citation1997) also stated that data points from clayey Gley soils on the coast of the Sea of Japan are located above the AN/TN regression line compared with Gray or Brown Lowland soils. Ando et al. (Citation1986) also reported that the decomposition rate of RS in poorly drained paddy fields with smectite-based soils in Fukushima prefecture was lower than that in well-drained paddy fields. Therefore, we considered the organic matter that was a potential source of AN to tend to accumulate more in clayey Gley soils.
A positive correlation between AN and MWH was observed, and higher R2 were obtained when Andosols and non-Andosols were separated (). The intercept of each regression line that depicted the relationship between AN and MWH was close to 0. This is the first report that clarified the correlation of the relationship between AN and MWH.
4.2. The influence of organic matter application and paddy–upland rotation on the relationship between AN and either TN or MWH
4.2.1. Fluctuation of AN due to fluctuation of TN treated by organic matter application and paddy–upland rotation
The AN and TN increased with the application of organic matter (Tables S1 and S2) and linear relationships between AN and TN were obtained, although the slopes of the regression lines were different for volcanic versus non-volcanic soils (). This finding was consistent with Wada et al. (Citation1981). Shibahara and Inubushi (Citation1997) also reported that TN and AN linearly increased after successive applications of organic matter.
The slope of the regression line depicting the relationships between TN and AN in volcanic soils was lower than that for other soils; because the ammonification rate (AN/TN) in Andosols ranged from 2.8 to 4.6% and tended to be lower than that in other soils (Table S2).
There were many soil samples for which the amount of AN exceeded 200 mg kg−1 due to the application of organic matter in the viscous Gley soils on the coast of the Sea of Japan, especially in the Tohoku and Hokuriku regions, such as Tsuruoka, Sakata, Nagaoka, Jouetsu, and Fukui (, Table S2). Kubota (Citation1992) found that the decomposition of RS into semi-poorly drained paddy fields was lower than in well-drained fields at the Niigata Agricultural Research Institute. These findings may explain why the decomposition of applied organic matter was reduced by soil-poor-drainage properties and high precipitation, especially on the coast of the Sea of Japan where the high soil moisture condition was retained during the winter, such as in Nagaoka (Shiratori et al. Citation2007).
In contrast, in Nagaoka, the AN and TN decreased in paddy–upland rotation plots, compared with those of the control plots (Table S2). However, the ammonification rate (AN/TN) in paddy soil was negatively affected by paddy–upland rotation, so, we reconfirmed that the amount of decrease in AN in the paddy–upland rotation plots was not fully explained by the amount of decrease in TN. (Sumida, Kato, and Nishida Citation2005; Nishida, Sekiya, and Yoshida Citation2013; Hirokawa, Inahara, and Koike Citation2011).
4.2.2. The relationship between TN and WHC
The highly correlative relationship (p < 0.01) between TN and WHC is presented in . As the TN increased due to organic matter application, the WHC increased. There was no statistically significant difference between the regression lines for volcanic and non-volcanic soils (p > 0.05). A highly positive correlation between TC and WHC was also obtained (p < 0.01, data not shown). These findings suggest that the soil organic matter content strongly affects WHC, which is an approximate indicator for the amount of water retained in the soil pore space after gravitational water has been leached out.
Figure 6. Relationship between total nitrogen of soil and soil water holding capacity. (Total n = 100).
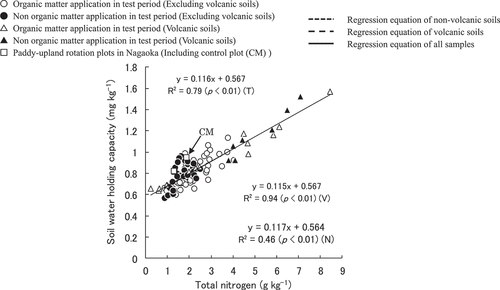
4.2.3. The relationship between WHC and MWH
The correlation between WHC and MWH is presented in . The WHC measurements obtained in the laboratory through the analysis of air-dried soil were larger than those for MWH (Tables S1 and S2). There was a highly positive correlation between WHC and MWH (, p < 0.01), but the regression line did not pass through the origin.
Figure 7. Relationship between soil water holding capacity and mass water content after harvest (Total n = 100).
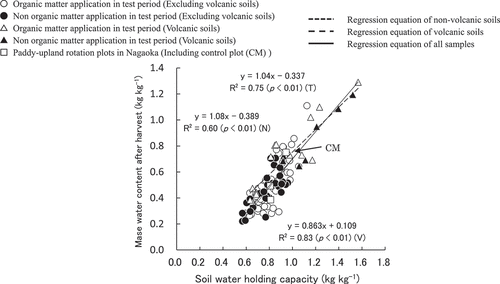
4.2.4. The relationship between AN and MWH
There is a high correlation between MWH and AN (, p < 0.01), due to the accumulation of organic matter. The slopes of the regression lines were different for volcanic and non-volcanic soils, and the trends for these differences were similar to those for the ammonification rate (AN/TN), which were similarly different for volcanic soils and non-volcanic soils.
The slopes of the regression lines that were obtained using the data from all soil samples, including plots with organic matter application between MWH and AN were 232 () for volcanic soils, and 353 () for non-volcanic soils, respectively. These values are larger than the slopes of the regression lines obtained for the control plots at each site shown in . Therefore, the differences in AN were compared with the differences in MWH, between the control plots and other plots, which are organic matter application plots, fertilizer-free plots, nitrogen-free plots, and paddy–upland rotation plots. As shown in , there was a positive correlation between these differences. The slopes of the regression lines in were larger than those of the regression lines for all the samples of the control plots ( regression equation (T)). From these results, it was confirmed that agricultural practices, such as organic matter application or paddy–upland rotation, influence the AN and MWH; as was demonstrated by the substantial changes in AN compared with soils in rice farming that were only treated with CF.
Figure 8. Relationship between the difference of available nitrogen of soil and the difference of mass water content after harvest (the difference obtained by subtracting the control plots from organic matter application, no-fertilizer, no-nitrogen, and paddy–upland rotation test plots). (n = 67).
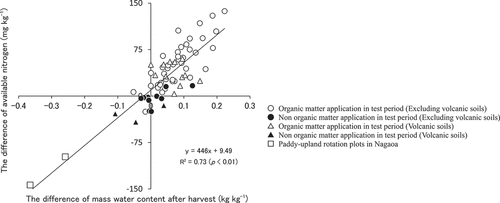
In short, both TN and WHC were altered by the accumulation or decomposition of organic matter in soil, and there was a positive correlation between them. AN was also correlated with TN, although the regression lines for volcanic soil differed from those for non-volcanic soil. In addition, there was a highly positive correlation between WHC and MWH. As a result, a positive correlation was observed between AN and MWH.
Nakano (Citation1980) found that the clayey Gley soil of paddy fields in Jouetsu on the coast of the Sea of Japan exhibited low water permeability in subsoils and high moisture in topsoils. However, repeated upland crop cultivation with improved subsoil drainage lowered moisture retention in these fields. Shiratori et al. (Citation2007) reported that improved drainage resulted in a decrease in the soil moisture and TN of clayey Gley soil, a semi-poorly drained paddy field, in Nagaoka on the coast of the Sea of Japan. These findings indicate that both the clay content and subsoil permeability affect moisture retention in topsoil and may alter soil organic matter, which is a source of AN. The decrease in decomposable organic matter during upland cultivation (e.g., soybean) in paddy fields may have opposite characteristics than the accumulation of organic matter via organic matter applications. For Gley soils on the coast of the Sea of Japan, there were many plots in which AN exceeded 200 mg kg−1. In these areas, precipitation is relatively high, and topsoil is likely to remain under wet conditions during the non-planting period (winter season). Nira and Miura (Citation2019) reported that the AN in fields treated with a winter flooding system was greater than that of non-treated fields. Although further study is needed, it is speculated that the moisture condition of topsoil during the non-planting period can affect the AN content of paddy soil.
4.3. Stability of MWH as an analysis factor
From the results of a year-to-year survey of MWH, it was confirmed that the MWH in a paddy field before autumn tillage was significantly affected by no-precipitation days before soil sampling. Fluctuations in MWH were observed in all soil types due to precipitation, especially in Gray Lowland soils and Andosols. The decreases in MWH values caused by long periods without precipitation were larger in plots with organic matter application than in the plots of non-organic matter application, as examined at Yamagata in 2013, Ryugasaki in 2011, and Gifu in 2012. Matsuo (Citation1962) surveyed soil water content with different pF values in unmodified soils and humus-decomposed soils treated with hydrogen peroxide, using three types of paddy soils collected throughout Japan. As a result of the pF value rose to 4.0 from 2.42, the difference in water content in unmodified soils was greater than that in humus-decomposed soils. These observations suggest that the fluctuations in MWH due to continuous no-precipitation days and precipitation just before soil sampling may be more pronounced in soils with high organic matter content. Furthermore, although the effect of precipitation varied with the timing of soil sampling each year and at each site, differences in the MWH between organic matter application plots and non-organic matter application plots remained significant (). Thus, the MWH content can be considered to be indicative of the effect of organic matter applications.
A negative correlation was present between continuous no-precipitation days before soil sampling and the values of MWH in the year of sampling, divided by the average of MWH over all the sampled years (). In particular, the MWH of fields with no-precipitation for 4 days or more tended to be lower than the average MWH. Nonoyama (Citation1981) investigated the pF value in fine-textured Gray Lowland soil from the Chugoku agricultural experiment station the field after harvesting, and found that the pF value increased sharply from 1 day after the last precipitation; and that it rose to 1.2 value 2 days after precipitation, to 1.6 value 3 days after precipitation, and to 2.0 value 6 days after precipitation. In view of the effect of dryness caused by no-precipitation, it was desirable to collect topsoil samples within 24–96 h after the last precipitation event as this period is assumed to be most closely representative of the state of field capacity (pF 1.5).
When precipitation was 90 mm week−1 or more before soil sampling in Gray Lowland soils of Toyama Prefecture in 2016 or in Ryugasaki and Jouetsu in 2013, the MWH was higher than in other years. Although the effect of precipitation within a week was unclear at other sites, it was assumed that the moisture content would not decrease with field capacity after heavy precipitation in fields where permeability of the plow-sole is low. Therefore, it was desirable to collect topsoil samples when the total precipitation for the preceding 7 days was less than 90 mm week−1.
In Gley soil, with high groundwater levels, at Kamikuzuro in 2015 and Oogata in 2011, the combined-harvesting operations were disrupted due to the low load bearing capacity of soil, caused by insufficient midsummer drainage or precipitation around the harvest season. Under such conditions, MWH was high. Yamanaka and Matsuo (Citation1962) reported a negative correlation between water content and soil hardness, and the decrease in soil hardness with the increase in water content of fine-textured soil was greater than that for coarse-textured soil. Therefore, when combine-harvesting in fine-textured Gley soil caused by low soil hardness was difficult, the value of MWH could be higher than its average value in other years.
Tillage and differences in sampling season may cause variations in MWH, probably due to the condition of incorporated organic matter and irregularity in topsoil dryness. Shiratori et al. (Citation2007) reported that soil moisture in the plowed layer in early spring before submergence of Gley soil in Nagaoka fluctuated due to weather conditions, including the total precipitation, number of rainy days, or percentage of rainy days after snow had thawed.
To obtain a representative value of MWH, it is desirable to collect topsoil within 24–96 h after the last precipitation event of 1 mm h−1 or more. In addition, a field is not suitable for sampling if the load bearing capacity of the soil (to support the weight of combined harvesters) could not be established, or if the precipitation was 90 mm or more within the 7 days before soil sampling. For example, for Andosols in Hironoshin, where the soil was sampled when the above conditions were satisfied, the coefficient of variation of MWH was relatively low for 4 years, at 0.026 ().
In addition, the soil structure in the topsoil of the paddy field was uniform due to tillage and puddling (Misono and Kawajiri Citation1967). For this reason, the soil samples in the paddy field could be uniformly corrected from the topsoil, which contributed to the significant relationship between AN and MWH.
By October, the rice harvest is concluded in almost all regions of Japan, and the monthly precipitation is typically 100–200 mm. This season is optimal for soil sampling, considering the above effects of precipitation.
Supplemental Material
Download Zip (177.6 KB)Acknowledgments
Special thanks are due to Dr. I. Uezono, Dr. S. Ito, Mr. T. Washio, and Mr. T. Wada for their advice with respect to available nitrogen. Paddy soil samples were provided by staff at the prefectural Agricultural Research Institutes of Iwate, Akita, Yamagata, Ibaraki, Tochigi, Chiba, Yamanashi, Niigata, Toyama, Fukui, Gifu, Mie, Shiga, Wakayama, Hyougo, Tottori, Okayama, Yamaguchi, Kochi, Fukuoka, Nagasaki, Kumamoto, Kagoshima, and the National Agricultural Research Center, to whom we are grateful. Part of this research was funded by the Ministry of Agriculture, Forestry and Fishery Department Project Research ‘Research and Development for Improving Profitability.’
Disclosure statement
No potential conflict of interest was reported by the author(s).
Supplementary material
Supplemental data for this article can be accessed here.
Correction Statement
This article has been republished with minor changes. These changes do not impact the academic content of the article.
References
- Ando, H., S. Shoji, T. Oikawa, and T. Sugano. 1986. “Decomposition of Rice Straw and Behavior of Nitrogen in Paddy Field Soil.” Japanese Journal of Soil Science and Plant Nutrition 57: 359–364. in Japanese. doi:https://doi.org/10.20710/dojo.57.4_359.
- Azuma, H., S. Takahashi, and N. Kato. 2015. “Nitrogen Mineralization Pattern in Moist and Air-dried Japanese Paddy Soils and the Relationship between Rice Yields and Nitrogen Mineralization.” Japanese Journal of Soil Science and Plant Nutrition 86: 175–187. in Japanese with English summary. doi:https://doi.org/10.20710/dojo.86.3_175.
- Dojou Kankyou Bunseki. 1997. “Analysis Methods of Soils and Environments.” edited by Editorial Board of Dojou Kankyou Bunseki, 24–29. Tokyo: Hakuyuusha. in Japanese.
- Food and Agricultural Materials Inspection Center. 1984. “Method of Cultivation Test on Harm to Plants.” http://www.famic.go.jp/ffis/fert/obj/sub2_7.html
- Fujii, H., H. Ando, T. Sato, and K. Aragaki. 1989. “The Nitrogen Mineralization Patterns of Paddy Soils in Shonai District.” Japanese Journal of Soil Science and Plant Nutrition 60: 8–14. in Japanese with English summary. doi:https://doi.org/10.20710/dojo.60.1_8.
- Harada, Y. 1984. “Exchangeable Capacity of Soil Cation and Anion: Measurement Method and Its Background.” Japanese Journal of Soil Science and Plant Nutrition 55: 273–283. in Japanese. doi:https://doi.org/10.20710/dojo.55.3_273.
- Hirokawa, T., M. Inahara, and J. Koike. 2011. “Declines in Soi1 Nitrogen Fertility after Rotation of Medium and Coarse-textured Gray Lowland Soi1 and Restoration Methods with Green Manure and Cattle Manure Compost.” Bulletin of the Agricultural Research Institute, Toyama Prefectural Agricultural, Forestry and Fisheries Research Center. 2: 11–12. in Japanese with English summary.
- Hirokawa, T., S. Ito, and Y. Kitagawa. 1993. “Behavior of Fertilizer Nitrogen and Soil Nitrogen in Gray Lowland Paddy Soil Improvement of Fertilization.” Bulletin of the Toyama Agricultural Research Center. 15: 1–66. in Japanese with English summary.
- Kamisato, C. 1958. “Soil Fertilizer Scientific Research of Soil with Upland and Paddy Field Rotation”. Abstracts of Annual Meeting Japanese Journal of Soil Science and Plant Nutrition 4: 62–63. doi:https://doi.org/10.20710/dohikouen.4.0_62.
- Kitada, K. 1990. “Kinetic Analysis for Nitrogen Mineralization of Paddy Soils.” Japanese Journal of Soil Science and Plant Nutrition 61: 241–247. in Japanese with English summary. doi:https://doi.org/10.20710/dojo.61.2_187.
- Kobo, K., and Y. Oba. 1973. “Cross Section of Volcanic Ash Soil and Volume Maximum Water Capacity.” Japanese Journal of Soil Science and Plant Nutrition 44: 1–10. in Japanese. doi:https://doi.org/10.20710/dojo.44.1_1.
- Kobo, K., and Y. Oba. 1974. “Effect of Accumulation Factors of Humus and Soil on Volcanic Ash Soil.” Japanese Journal of Soil Science and Plant Nutrition 45: 293–297. in Japanese. doi:https://doi.org/10.20710/dojo.45.6_293.
- Konno, T., and S. Sugihara. 1986. “Temperature Index for Characterizing Biological Activity in Soil and Its Application to Decomposition of Soil Organic Matter.” Bulletin of National Institute of Agro-environmental Science. 1: 51–68. in Japanese with English summary.
- Kubota, M. 1992. “Reasonable Application of Rice Straw to Wet and Semiwet Paddy Fields with Heavy Gley Soil in Niigata.” Journal of the Niigata Agricultural Experiment Station. 39: 1–87. in Japanese with English summary.
- Matsuo, K. 1962. “Relationship between Particle-size Composition of Soil and Water Retentivity (Part 2).” Japanese Journal of Soil Science and Plant Nutrition 33: 138–142. in Japanese. doi:https://doi.org/10.20710/dojo.33.3_138.
- Ministry of Agriculture, Forestry and Fisheries. 2008. “Basic Guidelines for Enhancement of Soil Fertility.” http://www.maff.go.jp/j/seisan/kankyo/hozen_type/h_dozyo/pdf/chi4.pdf
- Misono, S., and M. Kawajiri. 1967. “Study of the Three-phase Structure of Soils Report 1: 3-phase Structure under Field Condition.” Bulletin of the National Institute of Agricultural Sciences. 18: 49–128. in Japanese with English summary.
- Miyagi Prefectural Agricultural Research Center. 1992. “Estimation of Available Nitrogen by Soil Humus Content.” http://www.naro.affrc.go.jp/org/tarc/seika/jyouhou/H04/tnaes92023.html
- Nakano, K. 1980. “Changes in Physical Properties by Drainage of Montmorillonitic Heavy Clay Paddy Soils.” Journal of the Clay Science Society of Japan 20: 37–46. in Japanese with English summary and English tables and figures. doi:https://doi.org/10.11362/jcssjnendokagaku1961.20.37.
- Nira, R., and S. Miura. 2019. “Rice Yield and Soil Fertility of an Organic Paddy System with Winter Flooding.” Soil Science and Plant Nutrition 65 (4): 377–385. doi:https://doi.org/10.1080/00380768.2019.1632675.
- Nishida, M., H. Sekiya, and K. Yoshida. 2013. “Status of Paddy Soils as Affected by Paddy Rice and Upland Soybean Rotation in Northeast Japan, with Special Reference to Nitrogen Fertility.” Soil Science and Plant Nutrition 59 (2): 208–217. doi:https://doi.org/10.1080/00380768.2012.762588.
- Nonoyama, Y. 1981. “Effect of Plowing on the Physical Properties of Surface Soil in Well-drained Paddy Fields for Direct Sowing.” Bulletin of the Chugoku Agricultural Experiment Station. Series E. 18: 63–81. in Japanese with English summary.
- Odahara, K., Y. Fukushima, M. Araki, A. Kaneko, and K. Aramaki. 2012. “The Soil Fertility Status and Soybean Productivity in Paddy-upland Rotation Fields in Japan’s Chikugo River Basin.” Japanese Journal of Soil Science and Plant Nutrition 83: 405–411. in Japanese with English summary. doi:https://doi.org/10.20710/dojo.83.4_405.
- Shibahara, F., and K. Inubushi. 1997. “Effects of Organic Matter Application on Microbial Biomass and Available Nutrients in Various Types of Paddy Soils.” Soil Science and Plant Nutrition 43 (1): 191–203. doi:https://doi.org/10.1080/00380768.1997.10414727.
- Shiratori, Y., H. Watanabe, Y. Furukawa, H. Tsuruta, and K. Inubushi. 2007. “Effectiveness of a Subsurface Drainage System in Poorly Drained Paddy Field on Reduction of Methane Emissions.” Soil Science and Plant Nutrition 53 (4): 387–400. doi:https://doi.org/10.1111/j.1747-0765.2007.00171.x.
- Shoji, S., and T. Takahashi. 2002. “Environmental and Agricultural Significance of Volcanic Ash Soils.” Global Environmental Research 6 (2): 113–135.
- Sumida, H., N. Kato, and M. Nishida. 2005. “Depletion of Soil Fertility and Crop Productivity in Succession of Paddy Rice-soybean Rotation.” Bulletin of the National Agricultural Research Center Tohoku Region. 103: 39–52. in Japanese with English summary.
- Takahashi, K., S. Sunaga, and B. Kanke. 1984. “Changes of Soil Fertility in Converted Field from Paddy: (1) Effect of Cropping System on the Physical and Chemical Properties of the Soils and the Soil Microflora.” Bulletin of the Fukushima Prefecture Agricultural Experiment Station. 24: 17–28. in Japanese with English summary.
- Takakai, F., M. Takeda, K. Kon, K. Inoue, S. Nakagawa, K. Sasaki, A. Chida, et al. 2010. “Effects of Preceding Compost Application on the Nitrogen Budget in an Upland Soybean Field Converted from a Rice Paddy Field on Gray Lowland Soil in Akita, Japan.” Soil Science and Plant Nutrition 56 (5): 760–772. doi:https://doi.org/10.1111/j.1747-0765.2010.00503.x.
- The national institute of agro-environmental science. 1983. “Classification of Cultivated Soils in Japan Second Approximation Revised Edition.”
- Toriyama, K. 1994. “Studies on Estimation of Nitrogen Mineralization Pattern of Lowland Rice Field and Nitrogen Fertilizing Model for Rice Plant.” Bulletin of the Hokuriku Agricultural Experiment Station. 36: 147–198. in Japanese with English summary.
- Ueno, M., K. Kumagai, Y. Sato, and N. Tanaka. 1992. “Nitrogen Mineralization Potentials of Paddy Soil and Estimation of Soil Nitrogen Mineralization Amount by a Kinetic Analysis.” Bulletin of the Yamagata Prefectural Agricultural Experiment Station. 26: 121–136. in Japanese with English summary.
- Wada, H., K. Inubushi, Y. Uehara, and Y. Takai. 1981. “Relationship between Total Nitrogen Content and Ammonia Conversion.” Japanese Journal of Soil Science and Plant Nutrition 52: 246–252. in Japanese. doi:https://doi.org/10.20710/dojo.52.3_246.
- Yamanaka, K., and K. Matsuo. 1962. “Studies on Soil Hardness (Part 1).” Japanese Journal of Soil Science and Plant Nutrition 33: 343–347. in Japanese. doi:https://doi.org/10.20710/dojo.33.3_138.