ABSTRACT
Plants allocate large proportions of nitrogen (N) and iron (Fe) – principal elements in the photosynthetic electron transport system – to leaf chloroplasts. Although the molecular mechanisms to overcome N deficiency have been intensively studied, the acclimation of photosynthetic apparatus to Fe deficiency in higher plants remains to be further investigated. We previously reported about effective strategies in the chloroplasts of an Fe-deficiency-tolerant crop, barley, which allow photosynthesis under Fe-deficient conditions, not relying solely on Fe acquisition via the roots. The Fe-deficient barley leaves employ at least two strategies, viz., induction of thermal dissipation of absorbed light energy via Lhcb1 phosphorylation, which is maintained stably over Fe-deficiency periods regardless of light conditions, and improved electron flow in or around photosystem I through protein complexes with unknown composition. Previous studies have focused on healthy leaves from which chloroplasts can easily be isolated or on model organisms, such as Arabidopsis and cyanobacteria, to demonstrate typical photosystem structures and photochemical reactions. By contrast, in non-model plants, atypical photosystem structures and compositions have been reported. These structures are generally important to adapt to stress conditions or specific niches. In this review, we elucidate the mechanisms of acclimation of barley photosystems to Fe deficiency and provide an overview of the modulations in the photosystems of other plants under various stress conditions.
1. Introduction: an overview of the current understanding of mechanisms for efficient utilization of essential elements for photosystems in plants
Light absorption, conversion, electron transfer, and subsequent activation of metabolic pathways in chloroplasts are ubiquitous processes in photoautotrophs. Chloroplasts occupy a large volume of the cytoplasm and require high concentrations of essential elements for photosynthesis. Nitrogen (N), which has been intensively investigated as the most essential element in crop production, is indispensable for photosystems composed of chlorophyll and chlorophyll-binding proteins, ribulose-1,5-bisphosphate carboxylase/oxygenase (RuBisCo), and metabolic enzymes in the Calvin–Benson cycle (Terashima and Evans Citation1988). Partitioning of N to photosystems is dictated by growth irradiance, whereas that to RuBisCo correlates with N application (Terashima and Evans Citation1988; Hikosaka and Terashima Citation1996; Makino Citation2003; Niinemets Citation2007). RuBisCo is considered the most crucial target for improving photosynthesis (Evans Citation2013). It has been shown that the overproduction of RuBisCo increases the nitrogen use efficiency (NUE) of a large-grain rice cultivar (Makino Citation2021). Meanwhile, C4 plants allocate more N to the proteins on thylakoid membranes than C3 plants, owing to the low investment of N in RuBisCo accumulation, as C4 plants concentrate CO2 within the leaf cells to overcome the low affinity of RuBisCo for CO2 (Urban et al. Citation2021).
Low N availability decreases its investment in RuBisCo, whereas its allocation to mitochondria, which share the metabolic intermediates with chloroplast, is not reduced (Noguchi and Terashima Citation2006). Metabolic interactions between chloroplasts and mitochondria are altered under suboptimal conditions, such as cold stress or high light (Araújo, Nunes-Nesi, and Fernie Citation2014). Mitochondria increase NUE by regulating the carbon/nitrogen (C/N) ratio and redox status (Foyer, Noctor, and Hodges Citation2011).
Magnesium (Mg) performs pleiotropic functions in cells because it binds to various ligands and stabilizes macromolecules (Hermans et al. Citation2013). Mg is the central atom of chlorophyll and regulates photosynthesis through the acidification of the thylakoid lumen, maintaining a high stromal pH, and modulating the activity of key photosynthetic enzymes (Shaul Citation2002). Plants exhibit a wide range of Mg-deficiency symptoms, but the most evident symptom is interveinal chlorosis in older leaves, and it develops under high light conditions (Farhat et al. Citation2016; Hauer-Jákli and Tränkner Citation2019). Transcriptome analysis demonstrated that photosynthesis-related genes are downregulated at an early stage of Mg deficiency (Kobayashi and Tanoi Citation2015). Mg significantly contributes to NUE owing to its involvement in photosynthesis regulation (Tian et al. Citation2021). The importance of the balance between Mg and manganese (Mn) in photorespiration has also been highlighted, as they antagonistically bind to various enzymes, such as RuBisCo and malic enzyme (Shi and Bloom Citation2021). Mg application and genetic improvement of its uptake have been investigated to overcome its deficiency (Chaudhry et al. Citation2021).
As described above, the utilization of essential elements for photosynthesis has mainly been investigated with respect to macronutrients and stromal metabolism. There is a lack of studies on the responses of photosystems to deficiencies in essential elements, specifically transition metals that catalyze electron transport.
2. Transition metals involved in catalyzing electron transport in photosystems
Protein complexes of photosystem II (PSII), the cytochrome b6f complex, and photosystem I (PSI) catalyze multistep redox reactions to convert light energy to chemical energy. These proteins require transition metals to transfer electrons. Mn is necessary for the functioning of the O2-evolving complex of PSII; Fe is found in the reaction center of PSI, PSII, and the cytochrome b6f complex; and copper (Cu) is a component of plastocyanin (Raven, Evans, and Korb Citation1999). These transition metals are indispensable micronutrients for photochemical reactions in plants. The acclimation of chloroplasts to fluctuating light has been well investigated, and strategies to mitigate excess energy have been intensively studied and described in textbooks (Taiz et al. Citation2015 Chapter 7 and 9 In Plant Physiology and Development). The responses of photosystems to drought or heat/cold stress have also been reported (Hüner et al. Citation2012; Minagawa Citation2013; Wang et al. Citation2018). In contrast, the responses of photosystems to Fe-, Mn-, or Cu-deficiencies have been reported in algae (see sections 4 and 5, Bibby, Nield, and Barber Citation2001; Moseley et al. Citation2002; Merchant et al. Citation2020), but few studies have been conducted on similar lines in higher plants or crops.
A phenomenon termed ‘winterkill,’ which leads to the loss of winter barley and wheat without clear visual symptoms, was a major problem in northern Europe caused by Mn deficiency (Hebbern et al. Citation2005; Schmidt et al. Citation2013). Mn-deficiency-tolerant barley cultivars have been screened from among eight cultivars of winter barley (Hebbern et al. Citation2005). The difference in Vmax of high-affinity Mn transporters was identified as one of the factors underlying tolerance to Mn deficiency (Pedas et al. Citation2005). The functions of photosystems in Mn-deficient plants have also been investigated (Husted et al. Citation2009), and chlorophyll a fluorescence has been proposed as an indicator of latent Mn deficiency (Schmidt et al. Citation2013). Schmidt et al. (Citation2015) revealed that Mn-deficiency-tolerant barley cultivar, Vanessa, had more Mn per unit of PSII than susceptible cultivar Antonia, despite having lower or similar overall Mn concentration in leaves. A broad survey of Mn requirement among barley genotypes, including ancient landraces and modern elite cultivars, revealed that Mn transport and PSII maintenance are essential traits for Mn deficiency tolerance (Schmidt et al. Citation2019).
3. An overview of the reasons for high Fe demand in photosystems and the primary target of Fe deficiency
The thylakoid membrane is an Fe sink, which contains approximately 60–80% of Fe in the leaves of photoautotrophic higher plants (calculated from Terry and Low Citation1982 using sugar beet; Saito et al. Citation2021 using barley). PSI requires many Fe atoms, with 12 Fe atoms per unit of the three 4Fe-4S clusters (Raven, Evans, and Korb Citation1999; Schmidt, Eisenhut, and Schneider Citation2020), which makes it the potential primary target of Fe deficiency. This hypothesis was confirmed when the PSI electron transport rate was remarkably recovered by resupplying Fe compared with that of PSII (Pushnik and Miller Citation1989) and Fe deficiency caused a relative decrease in the efficiency of PSI and light-harvesting complex I (LHCI) compared with that of PSII, as estimated using chlorophyll fluorescence (Fodor et al. Citation1995). Moreover, the accumulation of PSI reaction center proteins dramatically decreased upon Fe deficiency (Saito et al. Citation2010, Citation2014; Higuchi et al. Citation2022). As a direct cause of PSI failure under Fe-deficient conditions, SUFB, a critical molecule in the sulfur utilization factor (SUF) machinery, which generates Fe-S clusters and inserts them into target proteins in chloroplasts, is immediately downregulated by Fe deficiency in several phototrophs (Liang et al. Citation2014; Georg et al. Citation2017; Hantzis et al. Citation2018; Higuchi et al. Citation2022). Preferential reduction of photosynthetic functions and the prioritization of mitochondrial functions under Fe-deficient conditions, similar to that under N- and Mg-deficiency conditions, may suggest the highest priority for the maintenance of organs by basic respiration, while photosynthesis-supported growth can be stalled. However, maintaining photosystem to a certain extent and the quick resumption of photochemical reactions when Fe is resupplied is beneficial for survival because the concentration of available Fe in soil often fluctuates due to changes in soil pH and redox potential (Lucas and Davis Citation1961; Schinas and Rowell Citation1977). Moreover, a long-term decline in photosynthesis during prolonged Fe deficiency causes energy depletion in plants and consequently profound loss of crop yield. ().
Figure 1. Model of the thylakoid membrane showing the PSII-LHCII complex, and the putative location of protein complexes. In addition to S-LHCII and M-LHCII, L-LHCII (loosely bound LHCII), which is not bound to the PSII reaction center, forms a large pool of LHCII trimer. Protein complexes indicated in red bind Fe to transfer electrons. Modified from Rantala, Rantala, and Aro (Citation2020), Pan et al. (Citation2020), and Cao et al. (Citation2018) .
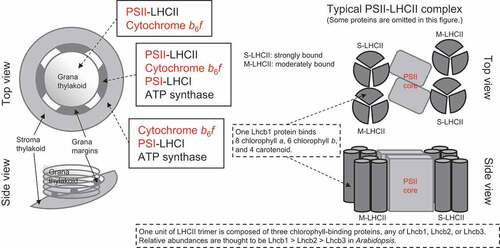
4. Multiple ways of acclimation to Fe deficiency in the barley chloroplast
The impact of Fe deficiency on photosystems is characterized by a dramatic decrease in the activity of PSI compared with that of PSII (Pushnik and Miller Citation1989; Fodor et al. Citation1995; Saito et al. Citation2010, Citation2014; Higuchi et al. Citation2022). This is different from the effect of other well-studied stresses, such as high light, high/low temperatures, or drought, which do not significantly alter the PSII/PSI ratio (Hüner et al. Citation2012; Minagawa Citation2013; Wang et al. Citation2018). The typical linear-electron flow in green plants starts from PSII; thus, electrons generated in PSII may not be adequately transported to PSI in Fe-deficient chloroplasts, causing oxidative stress. Any stress to photosystems stalls electron flow within them and causes oxidative stress; however, the origin and target of the oxidative stress differ according to the adverse growth condition. It is reasonable to assume that different mechanisms contribute to the acclimation to oxidative stress caused by various factors. Nevertheless, whether mechanisms underlying Fe-deficiency-specific acclimation are also operative in the chloroplasts of higher plants has not been investigated to date.
Barley is a well-known Fe deficiency-tolerant crop. Mugineic-acid family phytosiderophores (MAs), which solubilize Fe(OH)3, were originally found in barley root washings and are synthesized and excreted by graminaceous species (Takagi, Nomoto, and Takemoto Citation1984). It is believed that barley acquires more Fe as it has higher levels of MAs, and thereby, exhibits excellent tolerance (Takagi, Nomoto, and Takemoto Citation1984; Marschner, Römheld, and Kissel Citation1986). Overexpression of NAAT (nicotianamine aminotransferase), a gene involved in the biosynthesis of MAs, in rice caused them to become Fe-deficiency-tolerant (Takahashi et al. Citation2001). When barley and rice were grown in the same container under Fe-deficient conditions, the development of new rice leaves was arrested, whereas barley leaves continued to grow (Maruyama et al. Citation2005), further highlighting the high Fe-deficiency tolerance in barley. It has been shown that such co-cultivation allows rice to utilize more Fe using MAs released from barley roots and deprives barley of Fe-MAs, indicating a lower demand for Fe in the barley leaves than in rice leaves. This finding did not contradict the result that in barley, Fe is more preferentially distributed to the thylakoid membranes in Fe-deficient chloroplasts than it is in sorghum (Mikami et al. Citation2011). The difference in the demand for Fe is also observed among barley cultivars. Recently, we revealed that despite the high Fe-deficiency tolerance of the barley cultivar, Sarab 1, this cultivar exhibits a lower ability to acquire Fe from a nutrient solution with a very low concentration of Fe than do other cultivars with a weaker Fe-deficiency tolerance (Higuchi et al. Citation2022).
To identify candidate molecules that lead to Fe deficiency tolerance in leaves, we performed a transcriptome analysis using gene chips. We used leaves from the barley cultivar, Ehimehadaka 1, grown with 10 μM Fe in the case control plants or with 0.3 μM Fe in the case of Fe-deficient plants for 25 days (Higuchi et al. Citation2011). These conditions were determined based on cultivation with a stepwise gradient of Fe concentrations in the nutrient solution (Hirai et al. Citation2007). The most promising molecule was Lhcb1, whose homologs HvLhcb1.11 and 1.12 were induced in young leaves upon prolonged Fe deficiency (Saito et al. Citation2010). In cyanobacteria and certain eukaryotic algae, Fe-deficiency induces specific light-harvesting antenna proteins that dissipate excess light energy around PSI or support electron transfer around PSI under Fe-deficient conditions (Bibby, Nield, and Barber Citation2001; Moseley et al. Citation2002). However, these light-harvesting antenna proteins found in Fe-deficient algae are evolutionarily distinct from Lhcb1 in higher plants.
Lhcb1 is the major component of LHCII (Light-Harvesting antenna protein Complex of photosystem II), which binds chlorophylls and xanthophylls, but not Fe (Rantala, Rantala, and Aro Citation2020). Multiple genes encode Lhcb1 (5 in Arabidopsis thaliana, 3 in rice, and 17 in barley), forming the largest Lhcb subfamily. Therefore, qualitative, and quantitative changes in Lhcb1 significantly affect the overall structure of the thylakoid membrane. Among the major LHCII subunits (Lhcb1–3), Lhcb1 and Lhcb2 are reversibly phosphorylated on their N-terminal side, depending on the light intensity, via a specific kinase, STN7 (and partially via STN8), and phosphatase, TAP38/PPH1 (Pesaresi et al. Citation2011). Phosphorylation of LHCII proteins dramatically alters the light absorption properties, as described below. Lhcb3, on the contrary, is not phosphorylated and its presence regulates the overall phosphorylation (Damkjær et al. Citation2009). Hence, Lhcb3 is considered to fine-tune the behavior of LHCII (‘state transitions,’ as described below).
The basic structure of Lhcb1 and Lhcb2 is similar, and they have partially redundant functions. However, the phosphorylated forms of Lhcb1 and Lhcb2 have different roles in the acclimation of the photosynthetic machinery (Leoni et al. Citation2013; Pietrzykowska et al. Citation2014; Crepin and Caffarri Citation2015; Longoni et al. Citation2015; Rantala and Tikkanen Citation2018).
In Arabidopsis thaliana, Lhcb2 is more rapidly phosphorylated than Lhcb1. Under dark conditions, Lhcb2-containing LHCII trimers are dephosphorylated and exist in the grana thylakoids; they are located in the vicinity of PSII, and some of them bind directly to PSII forming PSII-LHCII supercomplexes (State I). By contrast, under weak light conditions, Lhcb2 subunits are phosphorylated. This can increase the fluidity of LHCII trimers in the membrane, which migrate from grana to non-appressed membranes (grana margin and stroma lamellae); this is followed by association with the PSI reaction center (State II). The ability of LHCII trimers to change their binding partner from PSII to PSI in a time window as small as 10 minutes, or state transition, is essential for adjusting the fast excitation energy balance between the photosystems and for increasing the efficiency of photosynthesis, especially under changing light conditions ().
Figure 2. Arrangement of LHCII containing Lhcb1 and acclimation to stress. Modified from Rantala, Rantala, and Aro (Citation2020), Yokono and Akimoto (Citation2018), and Xu, Chen, and Chen (Citation2015).
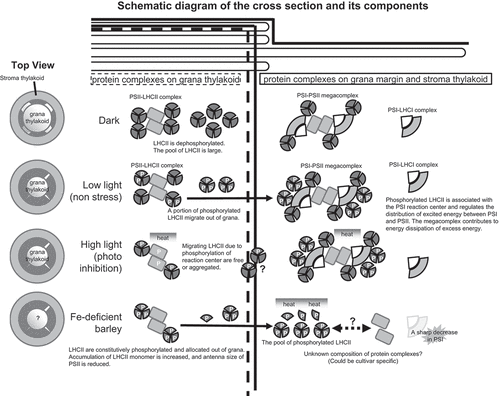
Unlike algae, in which state transition is a major component of nonphotochemical quenching (NPQ; Pietrzykowska et al. Citation2014) and is important for tolerance to high light stress, the role of state transition in higher plants is to support photosynthesis under low-light conditions. In fact, knockdown of Lhcb2 in Arabidopsis did not significantly affect the thermal dissipation of excess light energy (qE-type NPQ, described below; Pietrzykowska et al. Citation2014), suggesting that the main role of Lhcb2 is not photoprotective; it rather functions in the optimal alignment of LHCII in photosystems to maintain photosynthetic efficiency under various light conditions.
In contrast to Lhcb2, phosphorylated Lhcb1 does not bind well to PSI and, therefore, has a less obvious function in state transition (Crepin and Caffarri Citation2018). Instead, Lhcb1 is essential for the induction of the photoprotective thermal dissipation referred to as ‘energy-dependent quenching’ (qE), which is one of the components of NPQ. Hence, Lhcb1 is a vital antenna protein that reduces the occurrence of photooxidative stress under light stress conditions (although it usually has a light-harvesting function).
The pattern of Lhcb1 phosphorylation varies in different environments. In conifers, a specific long-lasting energy dissipation state, designated as ‘sustained NPQ,’ is induced in the winter season (Grebe et al. Citation2020). It has been suggested that phosphorylation of Lhcb1 in conifers has a role in sustained NPQ through the formation of the PSI-PSII megacomplex, with the ability to quench absorbed light energy as heat. Moreover, Lhcb1 was found to be phosphorylated at multiple sites in the PSI-PSII megacomplex; however, the physiological relevance of these phosphorylated forms remains unclear (Bag et al. Citation2020; Grebe et al. Citation2020).
In conifers (Grebe et al. Citation2020), the loosening of heterogeneity between photosystems as a consequence of Lhcb1 phosphorylation may be a key step in the assembly of the PSI-PSII megacomplex by increasing the frequency of encounter between PSI and PSII. Notably, the PSI-PSII megacomplex functions as an efficient quencher of light energy (a molecule that is involved in NPQ). This is because of direct energy transfer from PSII to PSI without electron transport, referred to as PSII-PSI spillover (Yokono and Akimoto Citation2018; Bag et al. Citation2020; Grebe et al. Citation2020; Rantala, Rantala, and Aro Citation2020) ; . However, it remains unclear whether Lhcb1 phosphorylation is an essential factor in the formation of the megacomplex in all higher plants. Other NPQ mechanisms involving Lhcb1 include structural changes in xanthophyll pigments in LHCII and function of the small PSII-subunit, PsbS, which interacts with LHCII (Wilk et al. Citation2013; Sacharz et al. Citation2017).
In addition to the aforementioned findings, we predicted that in Fe-deficient barley leaves, Lhcb1 plays an important role in the protection of photosystems. The amount of Lhcb1 that accumulates with Fe deficiency is clearly correlated with the extent of NPQ induction (Saito et al. Citation2010). Further analysis revealed that in Fe-deficient barley, Lhcb1 is stably phosphorylated, independent of light conditions; the phosphorylation is maintained even under dark and high light conditions (Saito et al. Citation2014). This phosphorylation of Lhcb1 causes a drastic change in the localization of Lhcb1 in the thylakoid membranes from grana to the non-appressed grana margin and stroma lamellae (Saito et al. Citation2014; ). Because LHCII trimers play a central role in the formation of the granum structure by facilitating a face-to-face contact between the N-terminus of two LHCII trimers, LHCII phosphorylation loosens the thylakoid-appressed membranes and decreases the grana core area. Such a loosening of the thylakoid membrane can be observed after LHCII phosphorylation (Wood et al. Citation2019; Grebe et al. Citation2020). Consistent with these findings, Fe-deficient barley showed the poor development of grana stacks and swollen thylakoid membranes; this was associated with the phosphorylation, and change in the localization, of Lhcb1 (Saito et al. Citation2014), . Although the link between Lhcb1 phosphorylation and acclimation of barley to Fe deficiency is not yet fully understood, increased amounts of phosphorylated Lhcb1 in the stroma membrane may cause the formation of efficient quenchers around PSI, such as the PSII-PSI megacomplex (Yokono and Akimoto Citation2018), LHCII aggregates (Ruban and Wilson Citation2021), or detached free antenna molecules (Saito et al. Citation2010), without inducing state transitions (Saito et al. Citation2014). Given that Fe deficiency can easily cause photoinhibition at any time because the plastoquinone pool becomes reductive due to the decrease in 4Fe-4S containing PSI (Saito et al. Citation2010), steady-state phosphorylation of Lhcb1 observed in barley would be advantageous for survival under prolonged Fe-deficient condition, like in the case of evergreen conifers that endure the harsh winter months (Bag et al. Citation2020; Grebe et al. Citation2020).
Figure 3. Grana stack in Fe-sufficient and -deficient leaves. We used barley, Ehimehadaka 1. The left panel shows a typical grana stack in Fe-sufficient leaves. The right panel shows a swollen grana-like structure in severely chlorotic leaves.
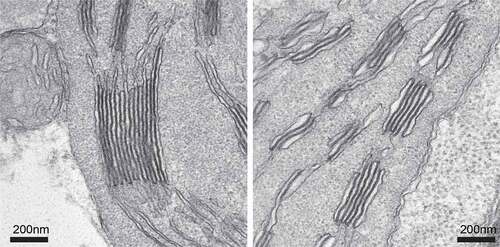
Our analysis to date has shown that the NPQ induced by Fe deficiency is a universal mechanism in Hordeum vulgare (Saito et al. Citation2021). In Fe-deficient spinach, NPQ increases with some changes in LHCII antenna organization, although the link to Fe-deficiency tolerance has not been discussed (Timperio et al. Citation2007). In contrast, Fe-deficient rice markedly decreases photosystem proteins, including Lhcb1, and does not induce NPQ (Saito et al. Citation2010). Similarly, some studies have shown that Arabidopsis plants fail to induce NPQ under Fe deficiency (Araki and Shikanai Citation2014; Hantzis et al. Citation2018). These results indicate that the induction of NPQ in leaves to cope with Fe deficiency might be a photoprotective mechanism acquired by some plant species that are tolerant to Fe deficiency. The barley mutant line, chlorina, which cannot accumulate Lhcb1 in the thylakoid membrane due to the absence of chlorophyll b (with little or no effect on the accumulation of other Lhcb isoforms), fails to elevate NPQ and exhibits necrotic spots on the leaves under Fe-deficient conditions (Saito et al. Citation2010). Thus, at least in barley, Lhcb1 is essential for survival under Fe-deficient conditions via the photoprotective induction of NPQ.
Barley-specific HvLhcb1.12, one of the major Lhcb1 isoforms in Fe-deficient barley, has more putative phosphorylation sites in the N-terminal region (Thr-37, Ser-45, Ser-46, Ser-47, and Ser-48), most of which are not found in Lhcb1 isoforms in other plants (Saito et al. Citation2014). Thus, an increased number of unique phosphorylation sites in HvLhcb1.12 may contribute to the steady-state Lhcb1 phosphorylation (Saito et al. Citation2014). To validate this assumption, HvLhcb1.12-specific mutants are needed. However, the barley chlorina lines that we have used thus far are mutants with reduced total Lhcb1 proteins including all the HvLhcb1 isoforms. Therefore, we attempted to create HvLhcb1.12-deficient barley mutants, although the specific knockdown/knockout of HvLhcb1.12 in barley has not been successful to date. To overcome this difficulty, we have developed transgenic rice lines harboring HvLhcb1.12 and are characterizing the structure and function of this molecule.
Recently, we investigated the photosynthetic Fe-use efficiency (PIUE) in an attempt to elucidate the differences in Fe economy among barley varieties grown under prolonged Fe-deficient conditions. PIUE is an index analogous to photosynthetic N-use efficiency (PNUE). PIUE was calculated as the net CO2 assimilation rate per leaf Fe content. Interestingly, we observed that relative PIUE change correlated better with Fe-deficiency tolerance based on plant biomass than conventional chlorophyll meter SPAD values or Fe content in the leaves of barley varieties (Saito et al. Citation2021). Furthermore, the difference in Fe-deficiency tolerance within H. vulgare varieties was found to be associated with the ability to increase PIUE under Fe-deficient conditions. Indeed, the ability to enhance PIUE during Fe deficiency was found only in Fe-deficiency-tolerant barley varieties. In this respect, the adaptation to Fe deficiency by elevated PIUE is variety-specific in barley. Thus, this mechanism may have been acquired through an evolutionary process that is different from the mechanism of NPQ induction universal to the H. vulgare subspecies described above. For this reason, an understanding of the mechanism underlying PIUE enhancement during Fe deficiency is crucial for providing further Fe-deficiency tolerance among barley species.
Among the various functions of photosynthesis, electron transfer downstream of PSII positively correlates with the relative PIUE value, suggesting a possible relation between the regulation of PSI function under Fe deficiency to PIUE (Saito et al. Citation2021). Although details of the underlying molecular mechanism are still unclear, our recent approaches highlight the need for a specific organization of photosystems to increase PIUE for photosynthesis (question mark in ).
It is unknown whether other plant species have developed Fe-deficiency acclimation mechanisms similar to that in barley because Fe-deficiency acclimation in chloroplast has not yet been investigated in many plant species. None of the C4 sorghum varieties that we measured could increase PIUE in Fe-deficiency conditions (Saito et al. Citation2021). Changes in PIUE among rice varieties are currently under investigation. In Arabidopsis, it was recently reported that the transcription factor ILR3 is required for phototolerance during Fe deficiency. Along with ILR3, the transcription factor PYE also confers photoprotection during Fe deficiency to prevent the accumulation of singlet oxygen, potentially by promoting the reduction in grana stacking to limit excitation and facilitating the repair of D1 protein in PSII (Akmakjian, Riaz, and Guerinot Citation2021). Further understanding of the linkage between the photoprotection process and known Fe deficiency-responsive elements for Fe transport systems in plants is awaited.
5. Perspective: resilient crops may have developed unknown atypical photosystems to adapt to adverse conditions
We found that barley cultivars in which PIUE is elevated under Fe-deficient conditions tend to be predominantly found in neutral or alkaline soils, which are at risk of Fe deficiency (Saito et al. Citation2021). The apparatus and function of photosystems are well-conserved among green plant species; the general structures of photosystems are universally conserved. However, the precise composition of proteins on the thylakoid membrane and the transfer of energy and electrons could be unexpectedly different among distinct genotypes (cultivars) in diverse environmental conditions. The differences in the acclimation of photosystems to Fe deficiency among barley cultivars suggests that we may have accelerated the adaptation of photosystems through breeding.
In addition to Mn and Fe, the structure of the thylakoid membrane and electron flow were affected by Cu deficiency in sugar beet (Droppa, Terry, and Horvath Citation1984) and wheat (Casimiro, Barroso, and Pais Citation1990). Moreover, one of the two plastocyanin genes from Arabidopsis was reported to respond to Cu addition (Abdel-Ghany Citation2009). However, there is little literature on the genetic variation in photosystems in response to Cu deficiency. Algae reduce plastocyanin to spare Cu under Cu-deficient conditions (Merchant et al. Citation2020). In an ocean diatom, Thalassiosira oceanica (CCMP1003), Hippmann et al. (Hippmann et al. Citation2017) reported a decrease in the cellular concentrations of proteins associated with the PSII core, an increase in those associated with the LHCII complex, and an increase in detached LhcII oligomers that occurred in response to low Cu to shift from light harvesting to photoprotection; however, strains with high Cu requirement did not exhibit a clear modulation of photosystems under Cu-limited conditions. Such a response to Cu deficiency in higher plants is unknown. This could be owing to the difficulty in obtaining many uniform cells acclimated to metal limitation in the leaves of higher plants because the developmental stages of mesophyll cells gradually progress from the bottom to the tip of the leaf.
The responses of photosystems to toxic elements have recently been reported. Cadmium (Cd) exhibits antagonistic behavior to essential transition metals and is known to disturb photosynthesis (Gallego et al. Citation2012). Cd has been reported to inhibit electron flow, especially around the reaction center of PSII (Lysenko et al. Citation2020; Adamakis et al. Citation2021). The direct inhibition of photosynthesis has been proposed to be more critical than micronutrient deficiency in the Cd/Zn hyperaccumulator Arabidopsis helleri under excess Cd conditions (Morina and Küpper Citation2020). Thioredoxin-like protein alleviates Cd-induced photoinhibition via cyclic electron flow and the xanthophyll cycle in tobacco plants (Zhang et al. Citation2021). Genetic variations in the responses of photosystems to Cd toxicity have also been reported. Chen et al. (Citation2019) investigated two rice cultivars and reported that tolerant cultivars alleviated the generation of reactive oxygen species and cell death independently of Cd concentration or antioxidant activities. Cd-resistant pea mutants are thought to acclimate to higher Cd concentrations in leaves than the wild-type owing to molecular mechanisms related to photosynthetic integrity (Belimov et al. Citation2020).
Except in halophytes, plant cells are pleiotropically affected by the accumulation of Na+ in the cytoplasm. The reduction in photosynthetic rate in the presence of excess Na+ was reported to be not only caused by stomatal closure but also by elevated NPQ followed by a decrease in quantum yield of PSII in cucumber (Stępień and Kłbus Citation2006). An invasive weed, salt-reed-grass (Desmostachya bipinnata), increased the quantity of photosystem proteins and the electron transfer rate and maintained the photosynthesis rate under 100 mM Na+ conditions (Asrar et al. Citation2017). Grass pea (Lathyrus sativus) is a commonly cultivated legume that can achieve a certain yield even when its leaves are wilted in the presence of excess Na+. This plant exhibits high plasticity – protein complexes in the photosystems in stem chloroplasts are reorganized, allowing the stem to function as an organ for CO2 assimilation, whereas Na+ is sequestrated in the leaves (Tokarz et al. Citation2021).
Chen et al. (Citation2020) recently demonstrated that enhancing the repair of D1 subunit protein in PSII reaction centers improves the photosynthetic efficiency and yield in Arabidopsis, tobacco, and rice. Cultivars that have acquired modified photosystems and unknown acclimation mechanisms to meet inadequate supply of essential elements will be extremely beneficial in agriculture as they can be successfully cultivated without excess fertilization or extreme exploitation of soils.
Disclosure statement
No potential conflict of interest was reported by the author(s).
References
- Abdel-Ghany, S. E. 2009. “Contribution of Plastocyanin Isoforms to Photosynthesis and Copper Homeostasis in Arabidopsis Thaliana Grown at Different Copper Regimes.” Planta 229 (4): 767–779. doi:10.1007/s00425-008-0869-z.
- Adamakis, I. D. S., I. Sperdouli, A. Hanć, A. Dobrikova, E. Apostolova, and M. Moustakas. 2021. “Rapid Hormetic Responses of Photosystem II Photochemistry of Clary Sage to Cadmium Exposure.” International Journal of Molecular Sciences 22 (1): 41. doi:10.3390/ijms22010041.
- Akmakjian, G. Z., N. Riaz, and M. L. Guerinot. 2021. “Photoprotection during Iron Deficiency Is Mediated by the bHLH Transcription Factors PYE and ILR3.” Proceedings of the National Academy of Sciences of USA 118 (40): e2024918118. doi:10.1073/pnas.2024918118.
- Araki, R., and T. Shikanai. 2014. “Analysis of Photosynthetic Electron Transport in Arabidopsis Thaliana under Iron Deficiency.” Journal of Crop Research 59: 11–15. https://www.jstage.jst.go.jp/article/jcr/59/0/59_KJ00009498022/_pdf/-char/ja.
- Araújo, W. L., A. Nunes-Nesi, and A. R. Fernie. 2014. “On the Role of Plant Mitochondrial Metabolism and Its Impact on Photosynthesis in Both Optimal and sub-optimal Growth Conditions.” Photosynthesis Research 119 (1–2): 141–156. doi:10.1007/s11120-013-9807-4.
- Asrar, H., T. Hussain, S. M. S. Hadi, B. Gul, B. L. Nielsen, and M. A. Khan. 2017. “Salinity Induced Changes in Light Harvesting and Carbon Assimilating Complexes of Desmostachya Bipinnata (L.) Staph.” Environmental and Experimental Botany 135: 86–95. doi:10.1016/j.envexpbot.2016.12.008.
- Bag, P., V. Chukhutsina, Z. Zhang, S. Paul, A. G. Ivanov, T. Shutova, R. Croce, A. R. Holzwarth, and S. Jansson. 2020. “Direct Energy Transfer from Photosystem II to Photosystem I Confers Winter Sustainability in Scots Pine.” Nature Communications 11: 6388. doi:10.1038/s41467-020-20137-9.
- Belimov, A. A., I. C. Dodd, V. I. Safronova, and K.-J. Dietz. 2020. “Leaf Nutrient Homeostasis and Maintenance of Photosynthesis Integrity Contribute to Adaptation of the Pea Mutant SGECdt to Cadmium.” Biologia Plantarum 64: 447–453. doi:10.32615/bp.2020.061.
- Bibby, T. S., J. Nield, and J. Barber. 2001. “Iron Deficiency Induces the Formation of an Antenna Ring around Trimeric Photosystem I in Cyanobacteria.” Nature 412: 743–745. doi:10.1038/35089098.
- Cao, P., X. Su, X. Pan, Z. Liu, W. Chang, and M. Li. 2018. “Structure, Assembly and Energy Transfer of Plant Photosystem II Supercomplex.” Biochimica et Biophysica Acta – Bioenergetics 1859 (9): 633–644. doi:10.1016/j.bbabio.2018.03.007.
- Casimiro, A., J. Barroso, and M. S. Pais. 1990. “Effect of Copper Deficiency on Photosynthetic Electron Transport in Wheat Plants.” Physiologia Plantarum 79 (3): 459–464. doi:10.1111/j.1399-3054.1990.tb02103.x.
- Chaudhry, A. H., S. Nayab, S. B. Hussain, M. Ali, and Z. Pan. 2021. “Current Understandings on Magnesium Deficiency and Future Outlooks for Sustainable Agriculture.” Frontiers in Plant Science 22: 1819. doi:10.3390/ijms22041819.
- Chen, Y. E., H. T. Mao, N. Wu, A. Khan, A. M. U. Din, C. B. Ding, Z. W. Zhang, S. Yuan, and M. Yuan. 2019. “Different Tolerance of Photosynthetic Apparatus to Cd Stress in Two Rice Cultivars with the Same Leaf Cd Accumulation.” Acta Physiologiae Plantarum 41 (12): 191. doi:10.1007/s11738-019-2981-z.
- Chen, J. H., S. T. Chen, N. Y. He, Q. L. Wang, Y. Zhao, W. Gao, and F. Q. Guo. 2020. “Nuclear-encoded Synthesis of the D1 Subunit of Photosystem II Increases Photosynthetic Efficiency and Crop Yield.” Nature Plants 6: 570–580. doi:10.1038/s41477-020-0629-z.
- Crepin, A., and S. Caffarri. 2015. “The Specific Localizations of Phosphorylated Lhcb1 and Lhcb2 Isoforms Reveal the Role of Lhcb2 in the Formation of the PSI-LHCII Supercomplex in Arabidopsis during State Transitions.” Biochimica Et Biophysica Acta (BBA) – Bioenergetics 1847 (12): 1539–1548. doi:10.1016/j.bbabio.2015.09.005.
- Crepin, A., and S. Caffarri. 2018. “Functions and Evolution of Lhcb Isoforms Composing LHCII, the Major Light Harvesting Complex of Photosystem II of Green Eukaryotic Organisms.” Current Protein & Peptide Science 19 (7): 699–713. doi:10.2174/1389203719666180222101534.
- Damkjær, J. T., S. Kereïche, M. P. Johnson, L. Kovacs, A. Z. Kiss, E. J. Boekema, A. V. Ruban, P. Horton, and S. Jansson. 2009. “The Photosystem II light-harvesting Protein Lhcb3 Affects the Macrostructure of Photosystem II and the Rate of State Transitions in Arabidopsis.” Plant Cell 21 (10): 3245–3256. doi:10.1105/tpc.108.064006.
- Droppa, M., N. Terry, and G. Horvath. 1984. “Effects of Cu Deficiency on Photosynthetic Electron Transport.” Proceedings of the National Academy of Sciences USA 81 (8): 2369–2373. doi:10.1073/pnas.81.8.2369.
- Evans, J. R. 2013. “Improving Photosynthesis.” Plant Physiology 162 (4): 1780–1793. doi:10.1104/pp.113.219006.
- Farhat, N., A. Elkhouni, W. Zorrig, A. Smaoui, C. Abdelly, and M. Rabhi. 2016. “Effects of Magnesium Deficiency on Photosynthesis and Carbohydrate Partitioning.” Acta Physiologiae Plantarum 38: 145. doi:10.1007/s11738-016-2165-z.
- Fodor, F., B. Böddi, É. Sárvári, G. Záray, E. Cseh, and L. Ferenc. 1995. “Correlation of Iron Content, Spectral Forms of Chlorophyll and chlorophyll-proteins in Iron Deficient Cucumber (Cucumis Sativus).” Physiologia Plantarum 93: 750–756. doi:10.1111/j.1399-3054.1995.tb05127.x.
- Foyer, C. H., G. Noctor, and M. Hodges. 2011. “Respiration and Nitrogen Assimilation: Targeting mitochondria-associated Metabolism as a Means to Enhance Nitrogen Use Efficiency.” Journal of Experimental Botany 62 (4): 1467–1482. doi:10.1093/jxb/erq453.
- Gallego, S. M., L. B. Penaa, R. A. Barcia, C. E. Azpilicueta, M. F. Iannone, E. P. Rosales, M. S. Zawoznik, M. D. Groppa, and M. P. Benavides. 2012. “Unravelling Cadmium Toxicity and Tolerance in Plants: Insight into Regulatory Mechanisms.” Environmental and Experimental Botany 83: 33–46. doi:10.1016/j.envexpbot.2012.04.006.
- Georg, J., G. Kostova, L. Vuorijoki, V. Schön, T. Kadowaki, T. Huokko, D. Baumgartner, et al. 2017. “Acclimation of Oxygenic Photosynthesis to Iron Starvation Is Controlled by the sRNA IsaR1.” Current Biology 27 (10): 1425–1436. doi:10.1016/j.cub.2017.04.010.
- Grebe, S., A. Trotta, A. A. Bajwa, I. Mancini, P. Bag, S. Jansson, M. Tikkanen, and E. M. Aro. 2020. “Specific Thylakoid Protein Phosphorylations are Prerequisites for Overwintering of Norway Spruce (Picea Abies) Photosynthesis.” Proceedings of the National Academy of Sciences 117 (30): 17499–17509. doi:10.1073/pnas.2004165117.
- Hantzis, L. J., G. E. Kroh, C. E. Jahn, M. Cantrell, G. Peers, M. Pilon, and K. Ravet. 2018. “A Program for Iron Economy during Deficiency Targets Specific Fe Proteins.” Plant Physiology 176 (1): 596–610. doi:10.1104/pp.17.01497.
- Hauer-Jákli, M., and M. Tränkner. 2019. “Critical Leaf Magnesium Thresholds and the Impact of Magnesium on Plant Growth and photo-oxidative Defense: A Systematic Review and meta-analysis from 70 Years of Research.” Frontiers in Plant Science 10: 766. doi:10.3389/fpls.2019.00766.
- Hebbern, C. A., P. Pedas, J. K. Schjoerring, L. Knudsen, and S. Husted. 2005. “Genotypic Differences in Manganese Efficiency: Field Experiments with Winter Barley (Hordeum Vulgare L.).” Plant and Soil 272: 233–244. doi:10.1007/s11104-004-5048-9.
- Hermans, C., S. J. Conn, J. Chen, Q. Xiao, and N. Verbruggen. 2013. “An Update on Magnesium Homeostasis Mechanisms in Plants.” Metallomics 5 (9): 1170–1183. doi:10.1039/c3mt20223b.
- Higuchi, K., A. Saito, Y. Mikami, and E. Miwa. 2011. “Modulation of Macronutrient Metabolism in Barley Leaves under Fe-deficient Condition.” Soil Science and Plant Nutrition 57 (2): 233–247. doi:10.1080/00380768.2011.564574.
- Higuchi, K., K. Kurita, T. Sakai, N. Suzui, M. Sasaki, M. Katori, Y. Wakabayashi, et al. 2022. ““Live-Autoradiography” Technique Reveals Genetic Variation in the Rate of Fe Uptake by Barley Cultivars.” Plants 11: 817. doi:10.3390/plants11060817.
- Hikosaka, K., and I. Terashima. 1996. “Nitrogen Partitioning among Photosynthetic Components and Its Consequence in Sun and Shade Plants.” Functional Ecology 10 (3): 335–343. doi:10.2307/2390281.
- Hippmann, A. A., N. Schuback, K. M. Moon, J. P. McCrow, A. E. Allen, L. J. Foster, B. R. Green, and M. T. Maldonado. 2017. “Contrasting Effects of Copper Limitation on the Photosynthetic Apparatus in Two Strains of the Open Ocean Diatom Thalassiosira Oceanica.” PLoS One 12 (8): e0181753. doi:10.1371/journal.pone.0181753.
- Hirai, M., K. Higuchi, H. Sasaki, T. Suzuki, T. Maruyama, M. Yoshiba, and T. Tadano. 2007. “Contribution of Iron Associated with high-molecular-weight Substances to the Maintenance of SPAD Value of the Young Leaves of Barley under iron-deficient Conditions.” Soil Science and Plant Nutrition 53 (5): 612–620. doi:10.1111/j.1747-0765.2007.00190.x.
- Hüner, N. P. A., R. Bode, K. Dahal, L. Hollis, D. Rosso, M. Krol, and A. G. Ivanov. 2012. “Chloroplast Redox Imbalance Governs Phenotypic Plasticity: The “Grand Design of Photosynthesis” Revisited.” Frontiers in Plant Science 3: 255. doi:10.3389/fpls.2012.00255.
- Husted, S., K. H. Laursen, C. A. Hebbern, S. B. Schmidt, P. Pedas, A. Haldrup, and P. E. Jensen. 2009. “Manganese Deficiency Leads to genotype-specific Changes in Fluorescence Induction Kinetics and State Transitions.” Plant Physiology 150 (2): 825–833. doi:10.1104/pp.108.134601.
- Kobayashi, N. I., and K. Tanoi. 2015. “Critical Issues in the Study of Magnesium Transport Systems and Magnesium Deficiency Symptoms in Plants.” International Journal of Molecular Sciences 16 (9): 23076–23093. doi:10.3390/ijms160923076.
- Leoni, C., M. Pietrzykowska, A. Z. Kiss, M. Suorsa, L. R. Ceci, E. M. Aro, and S. Jansson. 2013. “Very Rapid Phosphorylation Kinetics Suggest a Unique Role for Lhcb2 during State Transitions in Arabidopsis.” The Plant Journal 76 (2): 236–246. doi:10.1111/tpj.12297.
- Liang, X., L. Qin, P. Liu, M. Wang, and H. Ye. 2014. “Genes for iron-sulfur Cluster Assembly are Targets of Abiotic Stress in Rice, Oryza Sativa.” Plant, Cell and Environment 37 (3): 780–794. doi:10.1111/pce.12198.
- Longoni, P., D. Douchi, F. Cariti, G. Fucile, and M. Goldschmidt-Clermont. 2015. “Phosphorylation of the light-harvesting Complex II Isoform Lhcb2 Is Central to State Transitions.” Plant Physiology 169 (4): 2874–2883. doi:10.1104/pp.15.01498.
- Lucas, R. E., and J. F. Davis. 1961. “Relationships between pH Values of Organic Soils and Availabilities of 12 Plant Nutrients.” Soil Science 92 (3): 177–182. doi:10.1097/00010694-196109000-00005.
- Lysenko, E. A., A. A. Klaus, A. V. Kartashov, and V. V. Kusnetsov. 2020. “Specificity of Cd, Cu, and Fe Effects on Barley Growth, Metal Contents in Leaves and Chloroplasts, and Activities of Photosystem I and Photosystem II.” Plant Physiology and Biochemistry 147: 191–204. doi:10.1016/j.plaphy.2019.12.006.
- Makino, A. 2003. “RuBisCo and Nitrogen Relationships in Rice: Leaf Photosynthesis and Plant Growth.” Soil Science and Plant Nutrition 49 (3): 319–327. doi:10.1080/00380768.2003.10410016.
- Makino, A. 2021. “Photosynthesis Improvement for Enhancing Productivity in Rice.” Soil Science and Plant Nutrition. doi:10.1080/00380768.2021.1966290.
- Marschner, H., V. Römheld, and M. Kissel. 1986. “Different Strategies in higher-plants in Mobilization and Uptake of Iron.” Journal of Plant Nutrition 9 (3–7): 695–713.
- Maruyama, T., K. Higuchi, M. Yoshida, and T. Tadano. 2005. “Comparison of Iron Availability in Leaves of Barley and Rice.” Soil Science and Plant Nutrition 51 (7): 1035–1042. doi:10.1111/j.1747-0765.2005.tb00142.x.
- Merchant, S. S., S. Schmollinger, D. Strenkert, J. L. Moseley, and C. E. Blaby-Haas. 2020. “From Economy to Luxury: Copper Homeostasis in Chlamydomonas and Other Algae.” Biochimica et Biophysica Acta 1867 (11): 118822. doi:10.1016/J.BBAMCR.2020.118822.
- Mikami, Y., A. Saito, E. Miwa, and K. Higuchi. 2011. “Allocation of Fe and Ferric Chelate Reductase Activities in Mesophyll Cells of Barley and Sorghum under Fe-deficient Conditions.” Plant Physiology and Biochemistry 49: 513–519. doi:10.1016/j.plaphy.2011.01.009.
- Minagawa, J. 2013. “Dynamic Reorganization of Photosynthetic Supercomplexes during Environmental Acclimation of Photosynthesis.” Frontiers in Plant Science 4: 513. doi:10.3389/fpls.2013.00513.
- Morina, F., and H. Küpper. 2020. “Direct Inhibition of Photosynthesis by Cd Dominates over Inhibition Caused by Micronutrient Deficiency in the Cd/Zn Hyperaccumulator Arabidopsis Halleri.” Plant Physiology and Biochemistry 155: 252–261. doi:10.1016/j.plaphy.2020.07.018.
- Moseley, J. L., T. Allinger, S. Herzog, P. Hoerth, E. Wehinger, S. Merchant, and Hippler, M., et al. 2002. “Adaptation to Fe-deficiency Requires Remodeling of the Photosynthetic Apparatus.” EMBO Journal 21:6709–6720. doi:10.1093/emboj/cdf666.
- Niinemets, Ü. 2007. “Photosynthesis and Resource Distribution through Plant Canopies.” Plant, Cell and Environment 30 (9): 1052–1071. doi:10.1111/j.1365-3040.2007.01683.x.
- Noguchi, K., and I. Terashima. 2006. “Responses of Spinach Leaf Mitochondria to Low N Availability.” Plant, Cell and Environment 29 (4): 710–719. doi:10.1111/j.1365-3040.2005.01457.x.
- Pan, X., P. Cao, X. Su, Z. Liu, and M. Li. 2020. “Structural Analysis and Comparison of light-harvesting Complexes I and II.” Biochimica Et Biophysica Acta – Bioenergetics 1861 (4): 148038. doi:10.1016/j.bbabio.2019.06.010.
- Pedas, P., C. A. Hebbern, J. K. Schjoerring, P. E. Holm, and S. Husted. 2005. “Differential Capacity for high-affinity Manganese Uptake Contributes to Differences between Barley Genotypes in Tolerance to Low Manganese Availability.” Plant Physiology 139 (3): 1411–1420. doi:10.1104/pp.105.067561.
- Pesaresi, P., M. Pribil, T. Wunder, and D. Leister. 2011. “Dynamics of Reversible Protein Phosphorylation in Thylakoids of Flowering Plants: The Roles of STN7, STN8 and TAP38.” Biochimica Biophysica Acta 1807 (8): 887–896. doi:10.1016/j.bbabio.2010.08.002.
- Pietrzykowska, M., M. Suorsa, D. A. Semchonok, M. Tikkanen, E. J. Boekema, E. M. Aro, and S. Jansson. 2014. “The light-harvesting Chlorophyll a/b Binding Proteins Lhcb1 and Lhcb2 Play Complementary Roles during State Transitions in Arabidopsis.” Plant Cell 26 (9): 3646–3660. doi:10.1105/tpc.114.127373.
- Taiz, L., E. Zeiger, I. M. Møller, and A. Murphy eds. 2015. Plant Physiology and Development, Sixth, Massachusetts, USA: Sinauer Associates. Taiz, L., E. Zeiger, I. M. Møller, and A. Murphy eds
- Pushnik, J. C., and G. W. Miller. 1989. “Iron Regulation of Chloroplast Photosynthetic Function: Mediation of PS I Development.” Journal of Plant Nutrition 12 (4): 407–421. doi:10.1080/01904168909363962.
- Rantala, S., and M. Tikkanen. 2018. “Phosphorylation-induced Lateral Rearrangements of Thylakoid Protein Complexes upon Light Acclimation.” Plant Direct 2 (2): e00039. doi:10.1002/pld3.39.
- Rantala, M., S. Rantala, and E. M. Aro. 2020. “Composition, Phosphorylation and Dynamic Organization of Photosynthetic Protein Complexes in Plant Thylakoid Membrane.” Photochemical and Photobiological Science 19: 604–619. doi:10.1039/D0PP00025F.
- Raven, J. A., M. C. W. Evans, and R. E. Korb. 1999. “The Role of Trace Metals in Photosynthetic Electron Transport in O2-evolving Organisms.” Photosynthesis Research 60 (2–3): 111–149. doi:10.1023/A:1006282714942.
- Ruban, A. V., and S. Wilson. 2021. “The Mechanism of non-photochemical Quenching in Plants: Localization and Driving Forces.” Plant and Cell Physiology 62 (7): 1063–1072. doi:10.1093/pcp/pcaa155.
- Sacharz, J., V. Giovagnetti, P. Ungerer, G. Mastroianni, and A. V. Ruban. 2017. “The Xanthophyll Cycle Affects Reversible Interactions between PsbS and light-harvesting Complex II to Control non-photochemical Quenching.” Nature Plants 3: 16225. doi:10.1038/nplants.2016.225.
- Saito, A., T. Iino, K. Sonoike, E. Miwa, and K. Higuchi. 2010. “Remodeling of the Major light-harvesting Antenna Protein of PSII Protects the Young Leaves of Barley (Hordeum Vulgare L.) from Photoinhibition under Prolonged Iron Deficiency.” Plant and Cell Physiology 51 (12): 2013–2030. doi:10.1093/pcp/pcq160.
- Saito, A., M. Shimizu, H. Nakamura, S. Maeno, R. Katase, E. Miwa, K. Higuchi, and K. Sonoike. 2014. “Fe Deficiency Induces Phosphorylation and Translocation of Lhcb1 in Barley Thylakoid Membranes.” FEBS Letters 588: 2042–2048. doi:10.1016/j.febslet.2014.04.031.
- Saito, A., S. Shinjo, D. Ito, Y. Doi, A. Sato, Y. Wakabayashi, J. Honda, et al. 2021. “Enhancement of Photosynthetic iron-use Efficiency Is an Important Trait of Hordeum Vulgare for Adaptation of Photosystems to Iron Deficiency.” Plants 10: 234. doi:10.3390/plants10020234.
- Schinas, S., and D. L. Rowell. 1977. “Lime-induced Chlorosis.” European Journal of Soil Science 28 (2): 351–368. doi:10.1111/j.1365-2389.1977.tb02243.x.
- Schmidt, S. B., P. Pedas, K. H. Laursen, J. K. Schjoerring, and S. Husted. 2013. “Latent Manganese Deficiency in Barley Can Be Diagnosed and Remediated on the Basis of Chlorophyll a Fluorescence Measurements.” Plant and Soil 372: 417–429. doi:10.1007/s11104-013-1702-4.
- Schmidt, S. B., D. P. Persson, M. Powikrowska, J. Frydenvang, J. K. Schjoerring, P. E. Jensen, and S. Husted. 2015. “Metal Binding in Photosystem II Super- and Subcomplexes from Barley Thylakoids.” Plant Physiology 168 (4): 1490–1502. doi:10.1104/pp.15.00559.
- Schmidt, S. B., T. S. George, L. K. Brown, A. Booth, J. Wishart, P. E. Hedley, P. Martin, J. Russell, and S. Husted. 2019. “Ancient Barley Landraces Adapted to Marginal Soils Demonstrate Exceptional Tolerance to Manganese Limitation.” Annals of Botany 123 (5): 831–843. doi:10.1093/aob/mcy215.
- Schmidt, S. B., M. Eisenhut, and A. Schneider. 2020. “Chloroplast Transition Metal Regulation for Efficient Photosynthesis.” Trends in Plant Science 25 (8): 817–828. doi:10.1016/j.tplants.2020.03.003.
- Shaul, O. 2002. “Magnesium Transport and Function in Plants: The Tip of the Iceberg.” Biometals 15: 307–321. doi:10.1023/A:1016091118585.
- Shi, X., and A. Bloom. 2021. “Photorespiration: The Futile Cycle?” Plants 10: 908. doi:10.3390/plants10050908.
- Stępień, P., and G. Kłbus. 2006. “Water Relations and Photosynthesis in Cucumis Sativus L. Leaves under Salt Stress.” Biologia Plantarum 50 (4): 610–616. doi:10.1007/s10535-006-0096-z.
- Takagi, S., K. Nomoto, and T. Takemoto. 1984. “Physiological Aspect of Mugineic Acid, a Possible Phytosiderophore of Graminaceous Plants.” Journal of Plant Nutrition 7 (1–5): 469–477.
- Takahashi, M., H. Nakanishi, S. Kawasaki, N. K. Nishizawa, and S. Mori. 2001. “Enhanced Tolerance of Rice to Low Iron Availability in Alkaline Soils Using Barley Nicotianamine Aminotransferase Genes.” Nature Biotechnology 19: 466–469. doi:10.1038/88143.
- Terashima, I., and J. R. Evans. 1988. “Effects of Light and Nitrogen Nutrition on the Organization of the Photosynthetic Apparatus in Spinach.” Plant and Cell Physiology 29 (1): 143–155. doi:10.1093/oxfordjournals.pcp.a077461.
- Terry, N., and G. Low. 1982. “Leaf Chlorophyll Content and Its Relation to the Intracellular Location of Iron.” Journal of Plant Nutrition 5 (4–7): 301–310. doi:10.1080/01904168209362959.
- Tian, X. Y., D. D. He, S. Bai, W. Z. Zeng, Z. Wang, M. Wang, L. Q. Wu, and Z. C. Chen. 2021. “Physiological and Molecular Advances in Magnesium Nutrition of Plants.” Plant and Soil 468: 1–17. doi:10.1007/s11104-021-05139-w.
- Timperio, A. M., G. M. D’Amici, C. Barta, F. Loreto, and L. Zolla. 2007. “Proteomics, Pigment Composition, and Organization of Thylakoid Membranes in iron-deficient Spinach Leaves.” Journal of Experimental Botany 58: 3695–3710. doi:10.1093/jxb/erm219.
- Tokarz, K. M., W. Wesołowski, B. Tokarz, W. Makowski, A. Wysocka, R. J. Jędrzejczyk, K. Chrabaszcz, K. Malek, and A. Kostecka-Gugała. 2021. “Stem photosynthesis—A Key Element of Grass Pea (Lathyrus Sativus L.) Acclimatisation to Salinity.” International Journal of Molecular Sciences 22 (2): 685. doi:10.3390/ijms22020685.
- Urban, A., P. Rogowski, W. Wasilewska-Dębowska, and E. Romanowska. 2021. “Understanding Maize Response to Nitrogen Limitation in Different Light Conditions for the Improvement of Photosynthesis.” Plants 10: 1932. doi:10.3390/plants10091932.
- Wang, Q. L., J. H. Chen, N. Y. He, and F. Q. Guo. 2018. “Metabolic Reprogramming in Chloroplasts under Heat Stress in Plants.” International Journal of Molecular Sciences 19 (3): 849. doi:10.3390/ijms19030849.
- Wilk, L., M. Grunwald, P.-N. Liao, and W. Kühlbrandt. 2013. “Direct Interaction of the Major light-harvesting Complex II and PsbS in Nonphotochemical Quenching.” Proceedings of the National Academy of Sciences 110 (14): 5452–5456. doi:10.1073/pnas.1205561110.
- Wood, W. H. J., S. F. H. Barnett, S. Flannery, C. N. Hunter, and M. P. Johnson. 2019. “Dynamic Thylakoid Stacking Is Regulated by LHCII Phosphorylation but Not Its Interaction with PSI.” Plant Physiology 180 (4): 2152–2166. doi:10.1104/pp.19.00503.
- Xu, D., Y. Chen, and G. Chen. 2015. “Light-harvesting Regulation from Leaf to Molecule with the Emphasis on Rapid Changes in Antenna Size.” Photosynthesis Research 124 (2): 137–158. doi:10.1007/s11120-015-0115-z.
- Yokono, M., and S. Akimoto. 2018. “Energy Transfer and Distribution in Photosystem super/megacomplexes of Plants.” Current Opinion in Biotechnology 54: 50–54. doi:10.1016/j.copbio.2018.01.001.
- Zhang, H., X. Liu, H. Zhang, Y. Wang, T. Li, Y. Che, J. Wang, D. Guo, G. Sun, and X. Li. 2021. “Thioredoxin-like Protein CDSP32 Alleviates Cd-induced Photosynthetic Inhibition in Tobacco Leaves by Regulating Cyclic Electron Flow and Excess Energy Dissipation.” Plant Physiology and Biochemistry 167: 831–839. doi:10.1016/j.plaphy.2021.09.016.