ABSTRACT
Distinguishing headwater components can provide fundamental information for water resources management; however, this is difficult with the hydrometric method for regions with a thick unsaturated zone. We sampled headwaters, precipitation and groundwater in a river on China’s Loess Plateau to determine the isotopic composition, and identified headwater components by an isotope mass balance method. The isotopic composition of precipitation varies greatly, whereas that of groundwater is almost constant, which validates the applicability of the isotope mass balance method. During the dry season, the contributions of precipitation and groundwater to headwaters are both 50% for the upper reach, while they are 20 and 80%, respectively, for the lower reach; however, during the wet season, the contributions are, respectively, 67 and 33% for the upper reach, and 43 and 57% for the lower reach. The headwaters respond quickly to rainfall but are dominated by groundwater. Groundwater protection should be of high priority to sustain the catchment-scale hydrological cycle.
EDITOR D. Koutsoyiannis ASSOCIATE EDITOR M.D. Fidelibus
1 Introduction
Headwaters, defined as the source of a river, play an important role in the catchment-scale water cycle (Alexander et al. Citation2007, Freeman et al. Citation2007). Protection of headwaters is thus of utmost importance for the sustainability of the hydrological cycle. However, the headwaters comprise different components, such as groundwater discharge and rainfall-generated overland runoff (Pearce et al. Citation1986, Iwagami et al. Citation2010, Šanda et al. Citation2014). Against such a background, identifying headwater sources can provide fundamental information for water resources management.
Hydrometric- and tracer-based methods are two major approaches for water source identification (Pinder and Jones Citation1969, Klaus and Mcdonnell Citation2013). Hydrometric-based methods are very effective through analysing flow observations (Arnold et al. Citation1995, Tallaksen Citation1995); however, they are not applicable to ungauged catchments, or those regions with great water storage, which increases the complexity of hydrological process dynamics (Camacho Suarez et al. Citation2015). In such cases, tracer methods are superior for water source identification (Mcdonnell et al. Citation1990). In particular, the application of stable isotopes has challenged some existing theories in catchment hydrology (Klaus and Mcdonnell Citation2013); thus, the isotope-based methods for water source identification have been widely used.
After determining the isotopic composition of different water samples, water sources can be identified by a multi-component mass balance model (Kennedy et al. Citation1986, Ladouche et al. Citation2001, Iwagami et al. Citation2010). With these methods, a paradox has been observed in a wide variety of catchments, i.e. stream discharge responds promptly to rainfall, but with flows composed of water that can be years or decades old (Kirchner Citation2003, Klaus and Mcdonnell Citation2013). The paradox is attributed to the differences between flow velocity and celerity (Sklash and Farvolden Citation1979, Mcdonnell and Beven Citation2014). So far, the paradox is exhibited mostly in areas with a shallow water table, or the vadose zone where pressure propagation can easily produce large groundwater contributions to the stream (Sklash and Farvolden Citation1979). Would this phenomenon occur in catchments with a thick vadose zone? More studies should be carried out to improve our understanding of hydrological processes at the catchment scale.
The Loess Plateau of China (CLP) is a region with thick loessial soil layers (50–200 m thickness), and the loess tablelands have a soil layer with thickness greater than 170 m where the water table ranges from 30 to 100 m below ground level. The great water storages in the unsaturated zone increase the complexity of hydrological processes. In particular, little groundwater recharge is expected due to the existence of desiccation layers (Li Citation1983, Wang et al. Citation2010). If so, is streamflow dominated by groundwater in these areas? By answering this question, the hydrological changes as well as adaption measures can be clarified. For example, streamflow reduction and groundwater depletion are exhibited simultaneously across the CLP (Gao et al. Citation2015, Liang et al. Citation2015). However, the relationships between surface water and groundwater have rarely been studied, though a few studies have qualitatively concluded that groundwater had important effects on streamflow (Dou et al. Citation2009, Song et al. Citation2009, Su et al. Citation2009, Liu et al. Citation2010, Zhu et al. Citation2010); therefore, it is necessary to identify the water sources of streamflow, especially those of headwaters.
The objectives of this study are: (i) to analyse the spatiotemporal variation in isotopic composition of different water samples, and (ii) to determine the headwater sources in a watershed of the CLP. This study will improve the understanding of headwater sources in ungauged catchments. Furthermore, the results will be helpful for attributing the hydrological changes and sustainably managing water resources on the CLP.
2 Materials and methods
2.1 Study area
The Heihe River watershed (not the second largest inland river in northwest China, but a first-order tributary of the Jing River on the CLP), located in the tableland–gully regions of the CLP (Fig. 1), was selected as the study area. It has an area of 1506 km2. Based on climate data from the China Meteorological Administration, the mean annual precipitation is 571 mm year−1, with 55% of precipitation amounts falling during July–September (Fig. 2(a)), and the mean annual temperature is 9.4°C (1961–2012). The soil is predominantly silt loam, with silt content greater than 50%. With two main land-use types of farmland and medium grassland, the watershed is a typical agricultural watershed, as farmlands account for about 60% of the watershed (Li et al. Citation2010).
Based on the streamflow observations of the Yellow River Conservancy Commission, the mean annual runoff depth is 46.4 mm year−1 for the period of 1972–2000, which accounts for 9% of mean annual precipitation (Li et al. Citation2009). Although water shortage greatly limits regional development, streamflow and water table depth have been declining simultaneously over these years ()), further exacerbating the water-related environmental problems. Against such a background, it is necessary to know the mechanism of hydrological changes. Further, to take some countermeasures, the importance of each hydrological component should first be determined.
Figure 1. Sample sites for precipitation, headwaters and groundwater in the Heihe watershed for the period 2012–2013. The sampling sites for headwater are not on the main stream, but on the outlets of tributaries.
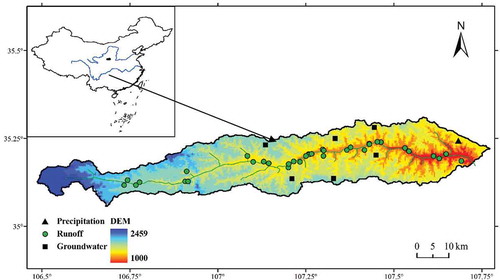
Figure 2. (a) Seasonal patterns of precipitation (P), potential evapotranspiration (ET0) and runoff depth (R). (b) Annual total runoff depth and mean water table in the Heihe watershed. Climate, runoff and water table data were collected, respectively, from the China Meteorological Administration, the Yellow River Conservancy Commission and the Water Conservancy Bureau of Changwu County.
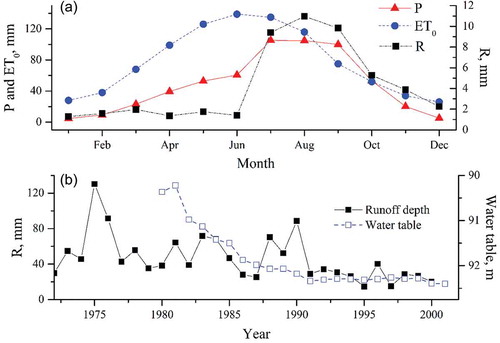
The tableland–gully regions have two main landforms – tablelands and gullies. The tablelands are large and flat, and the gullies have many steep slopes (Fig. 3(a)). The gullies cut below the bedrock; thus, the tablelands appear to be isolated islands (Zhu Citation1986). The hydrogeological conditions are similar across the catchment ()). The three top layers are composed of loess: the first layer is Malan Loess (Q3, Upper Pleistocene), with a thickness of 15 m; the second layer, i.e. Lishi Loess (Q2, middle Pleistocene), has a thickness of 71–74 m and is an aquifer due to unconsolidated sediments of relatively high porosity; and the third layer is Wucheng Loess (Q1, lower Pleistocene), with a thickness of 22–78 m, which forms an aquitard due to its low permeability (Huang et al. Citation2013).
Figure 3. (a) Landforms of the tableland–gully region (Zhu Citation1986). (b) Hydrogeological profile of the Heihe watershed.
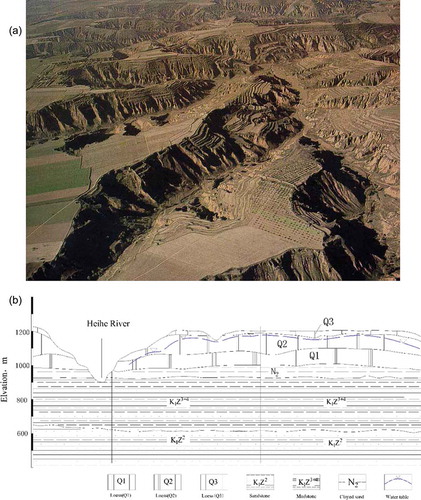
With these landforms and hydrogeological characteristics, the flow paths in this area can be described as follows. As the tablelands are large and flat, almost all precipitation infiltrates into the soil and recharges the groundwater. In the gullies, the overland flow generated from precipitation cannot recharge the groundwater since the water table (30–100 m) is higher than the bedrock (>100 m). Accordingly, the groundwater in the tablelands can only be discharged to gullies, while the streamflow in gullies flows into the main stream. Thus, the streamflow in gullies is the headwaters of the Heihe River, and it comprises groundwater discharged from the tablelands and overland flow generated from precipitation.
2.2 Water sampling and analysis
Three kinds of water, i.e. precipitation, groundwater and headwaters, were sampled during 2012–2013 to determine the isotopic composition. Precipitation was collected on a daily basis at one site in the lower reach. All rainfall water flowed directly into a bottle connected to a funnel, and a pingpong ball was put in the funnel to minimize evaporation. Groundwater in the form of springwater was sampled once per month in the head regions of six gullies at the middle and lower reaches (). The headwaters were sampled twice per year, i.e. June and August, at the outlets of the gullies before they join the main stream. June and August were chosen to represent the dry and wet seasons, respectively, according to the seasonal pattern of the hydroclimate ()). The streamflow in June is the smallest due to the greatest evaporation and moderate precipitation; however, the streamflow in August is the greatest due to the greatest precipitation and moderate evaporation. To quantify the contribution of rainfall inputs, the headwaters were sampled 1 day after a rainfall event. All precipitation, groundwater and runoff samples were stored in 100-mL polyethylene bottles and refrigerated at temperatures of about 4°C before analysis. The stable isotopic composition of the above samples was determined using an LGR LIWA V2 isotopic water liquid analyser with a precision of 0.5‰ for δ2H and 0.1‰ for δ18O. Besides water sampling, the stream discharge in a headwater catchment was measured to analyse the reliability of our sampling scheme, which will be shown in the discussion of uncertainties (Section 4.3). The data for isotopic composition of precipitation in 2005 and 2010 from our previous study (Chen et al. Citation2016) were used in this study to fully represent the overall isotopic characteristics.
2.3 Identifying headwater composition
The two-component isotope mass balance method was used to determine the water sources of the headwaters (Payne and Ortiz Citation1979, Song et al. Citation2006). This method requires that the two water sources, i.e. old water and new water, have distinct isotopic signatures, and it is especially efficient when old water (groundwater) has a constant isotopic composition and new water (generated from rainfall) can be characterized by the isotopic composition of the bulked rainfall (Mcdonnell et al. Citation1990). Then, water source identification can be carried out using the following mass balance equations:
where Q is flow amount, C is tracer concentration, and subscripts hw, pr and gw stand for headwaters, headwaters from precipitation, and headwaters from groundwater, respectively.
The feasibility of the two-component mass balance method was investigated first through comparing isotopic compositions of water samples. Then the contributions of precipitation and groundwater to the headwaters were quantified for different reaches and for different seasons. When carrying out water source identification, 18O was used as the tracer since it is linearly related to deuterium (D). The precipitation isotopic composition used in the equations was represented by that of the wet events occurring 1 day before the sample collection.
3 Results
3.1 Spatiotemporal variation in water isotopic composition
The daily-based isotopic composition of precipitation shows a wide range of variation, with values ranging from −19.6 to −1.2‰ for δ18O and −142.0 to −0.5‰ for δD. The isotopic signature exhibits a significant amount effect, i.e. the wet events with larger amounts have a more depleted isotopic composition (). In addition, the isotopic composition indicates a clear seasonal variation, i.e. the values in the first half-year (January–June) are more enriched than those in the second half-year (July–December) (). Taking δ18O as an example, the volume-weighted mean values for June and August are, respectively, −5.8 and −11.3‰ (Chen et al. Citation2016). The large variation in isotopic composition on a daily or monthly scale suggests that the precipitation isotopic composition can be used as a tracer for new water inputs of the headwaters.
Although groundwater was sampled at different locations and for different times, the δ18O values are relatively stable at −10.3 ± 0.6‰, which implies a negligible spatiotemporal variation (). Therefore, the old or pre-event water has almost constant isotopic composition.
Figure 4. Precipitation amount and oxygen isotopic composition in Changwu county within the Heihe watershed.
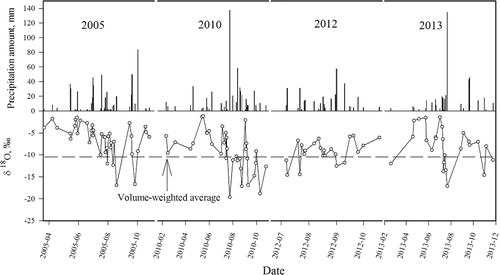
Figure 5. Dual isotopic comparisons between precipitation, groundwater and headwater for (a) June and (b) August. LMWL: local meteoric water line developed from precipitaton isotopic composition, δD = 7.36δ18O + 3.59 (R2 = 0.94). LEL: local evaporation line developed from headwater isotopic composition, δD = 5.439δ18O – 18.6 (R2 = 0.86) for June and δD = 7.552δ18O + 3.29 (R2 = 0.96) for August. Circle size denotes the monthly mean precipitation amount; numbers stand for the calendar month.
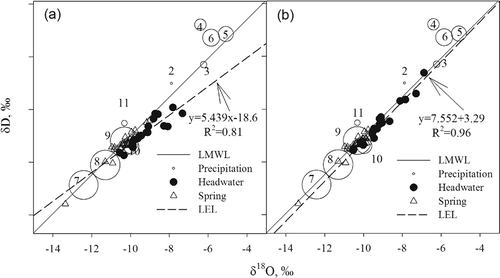
Across all sampling locations and time, the isotopic composition of the headwaters ranges from −10.6 to −6.9‰ for δ18O and −77.4 to −46.2‰ for δD. Averaged for each month, the mean isotopic composition is very similar; specifically, the δ18O in June and August is −9.4 and −9.2‰ for 2012, while values were −8.9 and −9.2‰ for 2013 (). However, the δ18O is actually temporally variable, especially in the upper reach. Taking 2012 as an example, the δ18O in June is more depleted than that in August because of different water inputs (Fig. 6). Although the δ18O of the headwaters varies temporally at the same sampling sites, the spatial pattern over the whole watershed is quite similar (); specifically, the δ18O in the upper reach is more enriched than that in the lower reach. Separating the watershed into upper and lower reaches by the longitude of 107.2º, the δ18O of headwaters is −8.2‰ and −9.7‰, respectively, for the upper and lower reaches, and a t-test showed that δ18O is significantly different in the two reaches (p < 0.01).
Table 1. Isotopic composition of headwater for the period 2012 − 2013.
3.2 Water sources of the headwaters
We compared the dual isotopic composition of different water samples to qualitatively interpret the water sources of the headwaters (). The local evaporation line (LEL) developed from all headwater samples is δD = 6.65δ18O − 6.22 (R2 = 0.86), with slope and interception smaller than those of the local meteoric water line (LMWL, δD = 7.36δ18O + 3.59, R2 = 0.94), which suggests that headwaters originate from precipitation but are subject to evaporation. However, the impacts of evaporation are quite different between June and August. Specifically, the LELs for June and August are δD = 5.439δ18O − 18.6 (R2 = 0.86) and δD = 7.552δ18O + 3.29 (R2 = 0.96), respectively. The smaller slope and interception of the LEL in June than those of the LMWL and LEL derived from two-month data ()) imply that the headwaters have experienced strong evaporation in June. Nevertheless, the LEL for August is almost parallel to the LMWL, and some points even fall above the LMWL ()), which indicates that the headwaters are more sensitive to precipitation inputs in August.
The contributions of precipitation and groundwater to headwaters were quantified for different reaches and seasons by the isotope mass balance method (). In the dry season (June in this study), the headwaters from precipitation and groundwater are both about 50% for the upper reach, while those for the lower reach are about 20% and 80%, respectively. During the wet season (August in this study), the contributions of precipitation and groundwater to headwaters are 67 and 33% for the upper reach, and 43 and 57% for the lower reach. From June to August, the contribution of groundwater to headwaters decreases, while that of precipitation increases; precipitation becomes the main water source of the upstream headwaters, but groundwater still dominates downstream headwaters.
Table 2. Contributions of precipitation and groundwater to headwaters for different reaches in June and August.
4 Discussion
4.1 Why are headwater sources spatially and temporally variable?
The isotopic composition of precipitation and headwaters varies temporally, whereas that of groundwater is almost constant, which implies that precipitation might play a major role in headwater generation. However, quantitative results from the mass balance method reveal that this conclusion is only applicable to the upper reach; instead, precipitation is a minor source of downstream headwaters. Specifically, from dry season to wet season (June–August in this study), the contribution of precipitation to headwaters in the upper reach increases from 52 to 67%, while that in the lower reach increases from 20 to 43%. The smaller contribution of precipitation in June can be interpreted by the evaporation and runoff generation mechanism. For example, the evaporation is greatest in June ()), which would decrease overland runoff and enrich the isotopic composition. According to the rainfall–runoff relationships in a headwater catchment for 2012 (Fig. 7(a)), runoff does not respond well to rainfall in the dry season due to small rainfall amounts and low antecedent soil moisture; however, it is more sensitive to rainfall during the flood season because of rainstorms and high soil water contents.
The isotopic composition of the headwaters exhibits a spatial variation, and the values for upstream headwaters are significantly greater than those in the lower reach (), which is possibly due to the differences in evaporation or water sources. If the spatial variation is determined by evaporation, corresponding to the larger isotopic composition in the upper reach, the upstream evaporation should be greater than that in the lower reach; however, the evaporation actually increases from the upper to lower reach. Therefore, evaporation should not be the dominant factor for spatial differences in headwater isotopic composition. Loess tableland can store more water than slopes due to its deep soil profiles; however, the upper reach has fewer tablelands than the lower reach. The large tablelands in the lower reach store more groundwater than the hilly region in the upper reach, and supply more groundwater to the headwaters, which is confirmed by the quantitative analysis of headwater components. The contribution of groundwater to headwaters is 33–48% for the upper reach and 57–80% for the lower reach. The results for the lower reach are similar to those from some other watersheds, based on hydrometric methods, such as 57–63% for the Lu River (Dou et al. Citation2009) and 72–94% for the Wuding River (Zhu et al. Citation2010).
4.2 What are the implications for water resources management and hydrological modelling?
Groundwater plays an important role in headwater generation and even dominates the downstream headwaters. Therefore, streamflow reduction in the watershed should be mainly due to groundwater depletion from the tablelands. However, with the increase of afforestation and water extraction, the water table has been declining in these years ()). Specifically, water infiltration and groundwater recharge has been greatly reduced because of substantial conversion from farmlands to apple orchards. Meanwhile, groundwater extraction, due to increases in industrial and domestic requirements, has been increasing intenstively (Wang et al. Citation2015). Therefore, groundwater protection in this region should be of high priority for the sustainable use of water resources.
The groundwater recharge mechanism in tablelands is controversial. Theoretically, groundwater originates only from precipitation, since tablelands are isolated as islands and gullies cut below the bedrock. However, it is unclear how precipitation recharges groundwater. The quantified contribution of groundwater to headwaters can be further used to interpret this issue. The diffuse recharge on tablelands accounts for 2–4% of mean annual precipitation (Huang and Gallichand Citation2006); however, the total groundwater discharge accounts for 5–7% of mean annual mean precipitation, which is calculated through multiplying the runoff coefficient (9%) by the groundwater contribution (57–80%). The difference between diffuse recharge (2–4%) and groundwater discharge (5–7%) is 1–5% of mean annual precipitation. If groundwater on tablelands comes only from diffuse recharge, groundwater depletion will be more severe in future, since diffuse recharge cannot balance groundwater discharge. However, this appears to be not true according to this study, as well as some previous studies (Lin and Wei Citation2006, Gates et al. Citation2011, Li et al. Citation2017).
If groundwater recharge is from piston flow, it takes precipitation hundreds of years to reach the water table (Huang et al. Citation2013). The slow diffuse recharge suggests that precipitation and groundwater are not in a steady state. According to this logic, the isotopic composition of groundwater should be similar to that of soil water but different from that of precipitation. However, the dual isotopic comparisons show that the isotopic composition of groundwater is similar to that of precipitation in August and September (). Further, our previous study showed that groundwater has different isotopic composition from soil water at depth (Li et al. Citation2017). As a consequence, groundwater can also be recharged by preferential flow from rainstorms in the flood season (July–September).
This conclusion about preferential flow challenges the traditional framework of hydrological modelling on the CLP. The groundwater is usually considered to be the result of vertical infiltration, i.e. diffuse recharge; however, we present evidence for preferential flow. This finding is consistent with those of Gates et al. (Citation2011) and Lin and Wei (Citation2006), who both concluded that vertical infiltration should not be only from groundwater sources, and that drainage through the unsaturated zone probably contributes little to spring discharge. Therefore, hydrological modelling on the CLP should take preferential flow into account for groundwater recharge.
4.3 How uncertain are the identified water sources?
Uncertainties are exhibited in the water source identification, which is mainly related to water sampling and the isotope mass balance method. As the isotopic composition of groundwater is almost invariant, it will not be influenced by the sampling scheme. Thus, we discuss the potential errors induced by headwater and precipitation sampling. We sampled headwaters 1 day after wet events to determine the isotopic composition, which is probably not appropriate for regions where runoff responds promptly to rainfall. We present the hydrograph and precipitation of a headwater catchment, from which 1 day lag is detected for most rainfall–runoff events in the flood season ()). As runoff is generated by excess infiltration overland flow, the initial-stage rainfall is absorbed by soil, but soil water contributes little to runoff composition (Horton Citation1933). Therefore, in the Heihe watershed, the sampling scheme for headwaters can represent the signal from precipitation.
We used the isotopic compositions of daily precipitation as new water inputs in the isotope mass balance method, which introduces uncertainties since the within-storm isotopic variability or the time response of the catchment to new water is not addressed (Mcdonnell et al. Citation1990). According to our results, the precipitation isotopic composition from wet events lasting for several days becomes more depleted with time (Chen et al. Citation2016). Therefore, if the isotopic composition of initial-stage rainfall absorbed by soil is excluded, that of rainfall for overland flow will be smaller than the daily values. In most cases, the isotopic composition of the headwater is greater than that of groundwater but smaller than that of precipitation; therefore, the contribution of precipitation to headwaters would be overestimated according to Equation (3). Nevertheless, the results should still be reliable since they are comparable with those from adjacent watersheds using hydrometric methods.
5 Conclusions
By determining the isotopic composition of precipitation, groundwater and headwaters, the headwater components were identified by the stable isotope mass balance method. Spatiotemporal variation is exhibited in the headwater composition. During the dry season, the contributions of precipitation and groundwater to runoff were both about 50% for the upper reach, while they were 20 and 80% for the lower reach; during the wet season, the corresponding values are, respectively, 67 and 33% for the upper reach, and 43 and 57% for the lower reach. Groundwater plays a major role in headwater generation, especially for the lower reach. The water balance estimation shows that groundwater should be recharged by diffuse and preferential flow.
Disclosure statement
No potential conflict of interest was reported by the authors.
Additional information
Funding
References
- Alexander, R.B., et al., 2007. The role of headwater streams in downstream water quality. JAWRA Journal of the American Water Resources Association, 43 (1), 41–59. doi:10.1111/jawr.2008.43.issue-1
- Arnold, J.G., et al., 1995. Automated base flow separation and recession analysis techniques. Ground Water, 33 (6), 1010–1018. doi:10.1111/gwat.1995.33.issue-6
- Camacho Suarez, V.V., et al., 2015. Understanding runoff processes in a semi-arid environment through isotope and hydrochemical hydrograph separations. Hydrology and Earth System Sciences, 19 (10), 4183–4199. doi:10.5194/hess-19-4183-2015
- Chen, X., et al., 2016. Analysis of stable isotopic composition and vapor source of precipitation at the Changwu Loess Tableland. Acta Ecologica Sinica, 36 (1), 98–106.
- Dou, L., Huang, M.B., and Hong, Y., 2009. Statistical assessment of the impact of conservation measures on streamflow responses in a watershed of the Loess Plateau, China. Water Resources Management, 23 (10), 1935–1949. doi:10.1007/s11269-008-9361-6
- Freeman, M.C., Pringle, C.M., and Jackson, C.R., 2007. Hydrologic connectivity and the contribution of stream headwaters to ecological integrity at regional scales. JAWRA Journal of the American Water Resources Association, 43 (1), 5–14. doi:10.1111/j.1752-1688.2007.00002.x
- Gao, Z., et al., 2015. Groundwater storage trends in the Loess Plateau of China estimated from streamflow records. Journal of Hydrology, 530, 281–290. doi:10.1016/j.jhydrol.2015.09.063
- Gates, J.B., et al., 2011. Impacts of soil conservation on groundwater recharge in the semi-arid Loess Plateau, China. Hydrogeology Journal, 19 (4), 865–875. doi:10.1007/s10040-011-0716-3
- Horton, R.E., 1933. The role of infiltration in the hydrologic cycle. Eos, Transactions American Geophysical Union, 14 (1), 446–460. doi:10.1029/TR014i001p00446
- Huang, M.B. and Gallichand, J., 2006. Use of the SHAW model to assess soil water recovery after apple trees in the gully region of the Loess Plateau, China. Agricultural Water Management, 85 (1–2), 67–76. doi:10.1016/j.agwat.2006.03.009
- Huang, T., Pang, Z., and Edmunds, W.M., 2013. Soil profile evolution following land-use change: implications for groundwater quantity and quality. Hydrological Processes, 27 (8), 1238–1252. doi:10.1002/hyp.9302
- Iwagami, S., et al., 2010. Role of bedrock groundwater in the rainfall–runoff process in a small headwater catchment underlain by volcanic rock. Hydrological Processes, 24 (19), 2771–2783. doi:10.1002/hyp.7690
- Kennedy, V.C., et al., 1986. Determination of the components of stormflow using water chemistry and environmental isotopes, Mattole River basin, California. Journal of Hydrology, 84 (1–2), 107–140. doi:10.1016/0022-1694(86)90047-8
- Kirchner, J.W., 2003. A double paradox in catchment hydrology and geochemistry. Hydrological Processes, 17 (4), 871–874. doi:10.1002/(ISSN)1099-1085
- Klaus, J. and Mcdonnell, J.J., 2013. Hydrograph separation using stable isotopes: review and evaluation. Journal of Hydrology, 505, 47–64. doi:10.1016/j.jhydrol.2013.09.006
- Ladouche, B., et al., 2001. Hydrograph separation using isotopic, chemical and hydrological approaches (Strengbach catchment, France). Journal of Hydrology, 242 (3–4), 255–274. doi:10.1016/S0022-1694(00)00391-7
- Li, Y.S., 1983. The properties of water cycle in soil and their effect on water cycle for land in the loess region. Acta Ecologica Sinica, 3 (2), 91–101.
- Li, Z., et al., 2009. Impacts of land use change and climate variability on hydrology in an agricultural catchment on the Loess Plateau of China. Journal of Hydrology, 377 (1–2), 35–42. doi:10.1016/j.jhydrol.2009.08.007
- Li, Z., et al., 2010. Assessing and regulating the impacts of climate change on water resources in the Heihe watershed on the Loess Plateau of China. Science China Earth Sciences, 53 (5), 710–720. doi:10.1007/s11430-009-0186-9
- Li, Z., et al., 2017. Determination of groundwater recharge mechanism in the deep loessial unsaturated zone by environmental tracers. Science of the Total Environment.
- Liang, W., et al., 2015. Quantifying the impacts of climate change and ecological restoration on streamflow changes based on a Budyko hydrological model in China’s Loess Plateau. Water Resources Research, 51 (8), 6500–6519. doi:10.1002/2014WR016589
- Lin, R. and Wei, K., 2006. Tritium profiles of pore water in the Chinese loess unsaturated zone: implications for estimation of groundwater recharge. Journal of Hydrology, 328 (1–2), 192–199. doi:10.1016/j.jhydrol.2005.12.010
- Liu, X., et al., 2010. Spatio-temporal variations of δ2H and δ18O in precipitation and shallow groundwater in the Hilly Loess Region of the Loess Plateau, China. Environmental Earth Sciences, 63 (5), 1105–1118. doi:10.1007/s12665-010-0785-y
- Mcdonnell, J.J. and Beven, K., 2014. Debates—the future of hydrological sciences: a (common) path forward? A call to action aimed at understanding velocities, celerities and residence time distributions of the headwater hydrograph. Water Resources Research, 50 (6), 5342–5350. doi:10.1002/2013WR015141
- Mcdonnell, J.J., et al., 1990. Deuterium variations in storm rainfall: implications for stream hydrograph separation. Water Resources Research, 26 (3), 455–458. doi:10.1029/WR026i003p00455
- Payne, B.R. and Ortiz, I.S., 1979. Determination of the mechanism of recharge in the Los Naranjos Area in Mexico using environmental isotope techniques. Water Resources Research, 15 (1), 171–175. doi:10.1029/WR015i001p00171
- Pearce, A.J., Stewart, M.K., and Sklash, M.G., 1986. Storm runoff generation in humid headwater catchments: 1. Where does the water come from? Water Resources Research, 22 (8), 1263–1272. doi:10.1029/WR022i008p01263
- Pinder, G.F. and Jones, J.F., 1969. Determination of the ground-water component of peak discharge from the chemistry of total runoff. Water Resources Research, 5 (2), 438–445. doi:10.1029/WR005i002p00438
- Šanda, M., et al., 2014. Run-off formation in a humid, temperate headwater catchment using a combined hydrological, hydrochemical and isotopic approach (Jizera Mountains, Czech Republic). Hydrological Processes, 28 (8), 3217–3229. doi:10.1002/hyp.v28.8
- Sklash, M.G. and Farvolden, R.N., 1979. The role of groundwater in storm runoff. Journal of Hydrology, 43 (1–4), 45–65. doi:10.1016/0022-1694(79)90164-1
- Song, X., et al., 2006. A study of interaction between surface water and groundwater using environmental isotope in Huaisha River basin. Science in China Series D: Earth Sciences, 49 (12), 1299–1310. doi:10.1007/s11430-006-1299-z
- Song, X.F., et al., 2009. Interactions between surface water and groundwater in Chabagou Catchment using Hydrogen and Oxygen isotopes. Journal of Basic Science and Engineering, 17 (1), 8–20.
- Su, X.S., et al., 2009. Hydraulic relationship between Malianhe River and groundwater: hydrogeochemical and isotopic evidences. Journal of Jilin University (Earth Science Edition), 39 (6), 1087–1094.
- Tallaksen, L.M., 1995. A review of baseflow recession analysis. Journal of Hydrology, 165 (1–4), 349–370. doi:10.1016/0022-1694(94)02540-R
- Wang, Y., Shao, M.A., and Liu, Z., 2010. Large-scale spatial variability of dried soil layers and related factors across the entire Loess Plateau of China. Geoderma, 159 (1–2), 99–108. doi:10.1016/j.geoderma.2010.07.001
- Wang, Y., et al., 2015. Characteristics of dried soil layers under apple orchards of different ages and their applications in soil water managements on the Loess Plateau of China. Pedosphere, 25 (4), 546–554. doi:10.1016/S1002-0160(15)30035-7
- Zhu, R., Zheng, H., and Liu, C., 2010. Estimation of groundwater residence time and recession rate in watershed of the Loess Plateau. Journal of Geographical Sciences, 20 (2), 273–282. doi:10.1007/s11442-010-0273-z
- Zhu, X., 1986. Land resources in the Loess Plateau of China. Xian: Shaanxi Science and Technique Press.