ABSTRACT
Relationships between surface waters and groundwaters at basin scale are rarely investigated but have important implications for water resource development and management. Here, we integrate evidence from geochemical tracers and piezometry to advance the understanding of regional-scale, groundwater–surface water interactions in the River Awash Basin of Ethiopia. Hydrological characteristics are consistent with those observed in other semi-arid and arid basins where rivers are predominantly losing and act as a source of recharge rather than as a sink for groundwater discharge. Further, regional groundwater flow originating from the highlands exits the catchment rather than discharging to the riverine drainage. Consequently, groundwater abstraction from several regional-scale aquifers in the lowlands is not expected to impact river flow. However, salinity presents a major threat to irrigation and water supply. We identify critical areas for managing inflows, water use, wetlands and water quality that have significant implications for water security across the basin.
Editor A. Fiori Associate editor C. Abesser
1 Introduction
Research assessing surface water–groundwater interactions has, to date, focused primarily on near-channel and in-channel exchanges of water, solutes and energy (Barthel and Banzhaf Citation2016), and their impacts on riverine ecology (Winter Citation1995) and water quality (Lamontagne et al. Citation2005, (Scanlon et al. Citation2007). Exchanges between surface water and groundwater serve as pathways for chemical exchange (Lamontagne et al. Citation2005, Kalbus et al. Citation2006, Schmidt and Schubert Citation2007) as even small-scale exchanges between groundwater and surface water can substantially affect water chemistry (Darling et al. Citation1996, Telford and Lamb Citation1999). Studies of regional-scale surface water–groundwater interactions are limited despite the important influences that these can exert downstream. There is growing interest among hydrology and hydrogeology communities in understanding the role of deeper regional flows in catchment hydrology (Condon et al. Citation2020).
Regional aquifers remote from the interface between groundwater and surface water can have a major impact on exchange processes at stream-reach scales (Schaller and Fan Citation2009, Barthel and Banzhaf Citation2016). River discharge in lowlands, for example, can derive from hydrological conditions in uplands that are more humid than lowlands. Understanding regional groundwater flow and its connection to surface waters also informs conceptual and numerical models of basin water resources. Hydrological models commonly route all waters in the basin to streams (Schaller and Fan Citation2009) even though substantial amounts of water can discharge to seas or inland lakes (Kafri and Yechieli Citation2012) without passing through a surface outlet.
The development of engineering infrastructure also impacts surface water–groundwater interaction regimes and water quality. Flow regulation and water diversion for irrigation have been shown to affect surface water–groundwater interactions (Simpson and Herczeg Citation1991, Lamontagne et al. Citation2005) and influence river-water temperature (Essaid and Caldwell Citation2017) as well as water salinity at reach (Konikow and Bredehoeft Citation1974) and regional scales (Leblanc et al. Citation2012). Changes in surface water and groundwater quality can negatively impact users of water for domestic, agricultural or industrial purposes. It is thus vital to understand regional-scale surface water–groundwater interactions, as they inform “under what conditions water infrastructure development can secure benefits for poor people and preserve the environmental assets on which they depend” (Mosello et al. Citation2015, p. 12).
The aim of this study is to characterize regional-scale surface water–groundwater interactions in the River Awash Basin (110 000 km2) of Ethiopia. Few studies of this kind and scale have been conducted in sub-Saharan Africa (Wolski and Savenije Citation2006, Kebede et al. Citation2016), and such analyses are challenging in complex data-scarce regions (Barthel and Banzhaf Citation2016). We employ a range of techniques, including geochemical and isotopic tracers as well as regional- and local-scale piezometry. We identify zones of major water gains and losses, and the factors that govern these flows. Specifically, we examine how the alteration of surface water–groundwater interactions in response to human interventions (e.g. dams, irrigation diversion) affects the salinity and volume of water resources. Substantial alterations of riverine hydrology in response to human interventions have been already noted in the River Awash Basin. The river, reported to have been intermittent during the 1960s (UNFAO Citation1965), is now perennial owing to the construction of dams and the accompanying flow regulation.
2 Basin characteristics
The River Awash Basin is the most developed of all river basins in Ethiopia, with over half (~57%) of its annual 4.9 billion m3 surface water flow already committed to irrigation, hydropower and drinking-water supply. Similar to other arid basins, the River Awash Basin is characterized by (1) inconsistent hydrological records, (2) a low mean specific discharge (Dai and Trenberth Citation2002), (3) the presence of large-storage deep aquifers with regional flow systems featuring long residence times (Bierkens Citation2015), and (4) saline groundwater bodies. The basin is situated almost entirely within the East African Rift System (), which is tectonically active (Williams et al. Citation2004) and includes hot springs, fumaroles and geysers (Battistelli et al. Citation2002) Pürschel et al. Citation2013). The locations of the various river discharge sites and the boundaries of sub-basins within the River Awash Basin are shown in .
Figure 1. Location map showing salient features of the River Awash Basin (irrigation water intake sites, geothermal spring discharge sites, extent of major aquifers, drainage, closed basins enclosed within the River Awash Basin, and place names). The major regional aquifers are those bounded inside numbered polygons
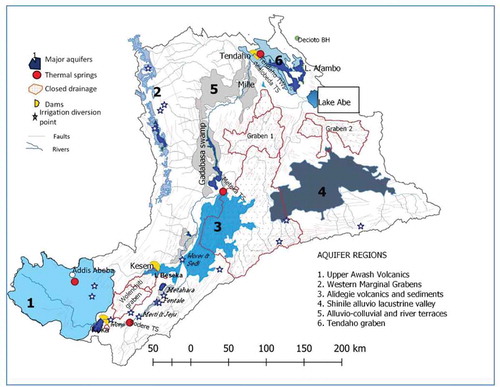
Figure 2. Locations of river gauging stations on the River Awash and its headwater tributaries; polygons inside the basin denote closed surface water sub-basins; most river flow originates from the Upper Awash Basin, upstream of Lake Koka in the southwest
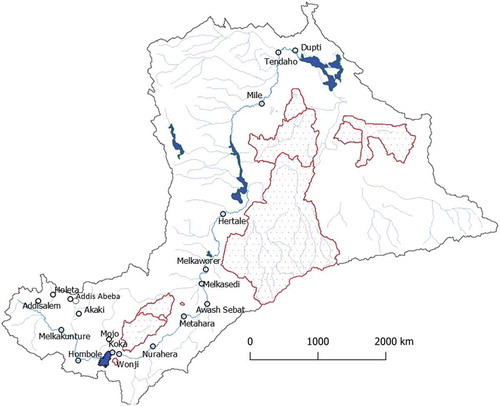
In the lower and middle parts of the basin, groundwater levels have declined dramatically since the Late Pleistocene (UNDP Citation1973, Gasse and Street Citation1978, Gasse and Fontes Citation1989, Kafri and Yechieli Citation2012). Although regional static water levels dropped in response to long-term changes in climate, aquifers possessing substantial storage still exist at depth. The most productive aquifers in Ethiopia, with the capacity to buffer rainfall variation during droughts, are found in the River Awash Basin (Calow et al. Citation2006, Ayenew et al. Citation2008a) and comprise a range of lithologies (summarized in ); their areal extent is depicted in . These aquifers are supplied by regional groundwater inflow from the plateau (Kebede et al. Citation2007) and by flash floods. Groundwater dynamics in these aquifers and their connection to river discharge remain largely unknown. Geothermal studies attribute the origin of thermal springs () in the valley floor to regional flows originating from these aquifers (Beyene Citation2000, Battistelli et al. Citation2002, Kebede et al. Citation2007, Ayenew et al. Citation2008b). River discharge occurs primarily within alluvial sediments and volcanic bedrock (). The volcanic bedrock is principally composed of basalts and ignimbrites.
Table 1. Salient features of the major aquifers in the Awash Basin. U = unconfined, SC = semi-confined, A = artesian, UWS = urban water supply, Ir = irrigation, Ind = industry, RWS = rural water supply. Source: Ministry of Water Irrigation and Electricity groundwater study reports pertaining to the stated aquifers (2008–2018)
Against a general decline in groundwater levels, Lake Beseka has experienced a dramatic increase in its level and area along with a decrease in salinity over the last six decades (Alemayehu et al. Citation2006). Electrical conductivity (EC) decreased from nearly 50 000 µS cm−1 in the early 1960s to <4500 µS cm−1 in 2014. The expansion of the lake has caused serious environmental and socio-economic problems, including flooding of urban utilities and farms as well as the inundation of roads and railway lines. Several arguments have been put forth regarding the cause of the lake’s expansion, including (1) increase in discharge of geothermal springs feeding the lake (Goerner et al. Citation2009), and (2) increase in groundwater inflow caused by seepage losses in the irrigation areas (Kebede and Zewdu Citation2019). Others describe the expansion of the lake as mysterious (Belay Citation2010). Current water resource management challenges in the River Awash Basin include upstream–downstream conflicts (Mosello et al. Citation2015), water scarcity during dry seasons (Adeba et al. Citation2015), flooding during wet seasons (Koriche and Rientjes Citation2016), and salinization of soils and water-logging (Meaza et al. Citation2017).
3 Methodology and sampling
The present analysis employs a range of methods including chemical tracers, piezometric observations and simple water-balance calculations that maximize the use of available datasets.
3.1 Theoretical considerations
222Rn: Through the natural production of 222Rn in rocks, groundwater flowing through the subsurface acquires higher 222Rn concentrations relative to surface water. The concentration of 222Rn in surface water is a function of the radon balance at the land surface and input from baseflow derived from groundwater. 222Rn has been widely used to qualitatively and quantitatively assess spatial variations in groundwater–surface water interaction (Yoneda et al. Citation1991, Cook et al. Citation2006, Citation2008, Schmidt and Schubert Citation2007, Peterson et al. Citation2008, Mullinger et al. Citation2009, Dimova and Burnett Citation2011, Kluge et al. Citation2012, Rodellas et al. Citation2012, Cartwright et al. Citation2014, Cartwright and Gilfedder Citation2015, Cartwright and Hofmann Citation2015). In rivers, 222Rn concentration typically vary with changes in baseflow from groundwater. Seasonal variations in 222Rn during high and low river stages must be characterized to effectively trace surface water–groundwater interactions (Mullinger et al. Citation2009).
Stable isotope ratios of oxygen (18O/16O) and hydrogen (2H/1H): stable isotope ratios of O and H in water are proven tracers for qualitatively and quantitatively evaluating surface water–groundwater exchanges, since surface waters are generally enriched in the heavy isotopes (18O, 2H) relative to groundwaters due to isotopic fractionation during evaporation. They are widely used to detect leakage from lakes to aquifers (Darling et al. Citation1996, Kebede et al. Citation2009, Gibson et al. Citation2016) and to detect river water outflow to adjacent groundwaters in arid environments (Cook Citation2015).
Piezometric records: maps of hydraulic heads are widely used to assess surface water–groundwater interactions. Local-scale piezometric maps (100 m) are widely used in computing surface water–groundwater interactions using Darcy’s law (Mcdonald et al. Citation2013). At regional scales, a lack of data as well as heterogeneity and anisotropy in hydraulic conductivity complicates the interpretation of piezometric evidence. Studies employing piezometric data to investigate regional surface water–groundwater interactions (Barthel and Banzhaf Citation2016) argue that piezometers need to be located hundreds of metres from the river to detect regional rather than local fluxes (Cook Citation2015).
3.2 Sampling and materials
Spot sampling of the River Awash and Lake Beseka to measure 222Rn concentrations was conducted in February 2017 (dry season) and August 2017 (wet season). A total of 40 sites were measured twice each on the main river channel. Additionally, 16 222Rn measurements of Lake Beseka and adjacent groundwaters were made in March 2014. An RAD7 radon detector, fitted with a Big Bottle System for high sensitivity (Durrige Company Inc Citation2020), was used to analyse river and groundwater samples. The RAD7 was fitted with RAD AQUA for sampling at Lake Beseka. The detection limit of the device is on the order of 40 Bq.m−3. Counting is based on the principle of liquid–gas-membrane extraction (Schmidt et al. Citation2008). An extraction module, which consists of hollow vinyl fibres, allows radon stripping from the water of interest into a connected closed air-loop. The water temperature was measured to convert 222Rn in air to 222Rn in water. The overall standard deviation varies between 25 and 30%, higher for low radon content. Radon counting was conducted over a period of 2 h in four cycles. The first reading was discarded and the average of the last three readings was taken as the mean 222Rn composition of the specific water point. Accompanying 222Rn measurement, a measurement of EC was also taken.
Stable isotope ratios of H (2H/1H) and O (18O/16O) in a total of 53 water points (boreholes, river water, springs and wetland waters) were analysed at the Centre National de l’Energie, des Sciences et des Techniques Nucleaires (CNESTEN), Morocco, using a liquid water isotope analyser. Data collected under previous studies between 1997 and 2014 from multiple sources (aquifers, geothermal waters, lakes, river) were also collated from International Atomic Energy Agency- Technical Cooperation projects (ETH8003, ETH8005, ETH8007). In total, this dataset includes 400 samples analysed in the IAEA Isotope Hydrology Laboratory, Vienna Austria (ETH8/003, ETH8005/ETH80060), and in the Laboratory of Nuclear Physics of the University of Krakow, Poland (ETH8/007). 18O-2H results are reported in per-mill (‰) notation calibrated against Vienna-Standard Mean Ocean Water (see for the location of sampling sites).
Static water levels (SWLs) were used to construct regional piezometric contours. SWLs were obtained from the Ethiopian Water Works Design and Supervision Enterprise, a government company involved in various groundwater exploration projects in the Awash Basin. SWLs were collected at the time of drilling from the 1980s to the present. Measurements are available from ~1300 sites. In the middle and lower parts of the basin, where regional groundwater flow dominates (UNDP Citation1973, Darling Citation1996), we employ SWLs amassed over the last 30 years to represent contemporary groundwater flow systems across the basin. Despite variability in the dates of recorded SWLs, we expect temporal variations in SWLs to be minor compared to the regional scale of amassed observations. In the relatively humid Upper Awash Basin, temporal changes in water level are expected to dominate spatial changes. In addition to regional water-level information, three sites with dense piezometric monitoring networks correspond to major irrigation areas in Wonji, Metahara and Melka Sedi-Melka Worer farms. The three major irrigation farms have been taking monthly water level records from multiple piezometers since the 1970s.
4 Results
4.1 Environmental tracers (18O, 2H, 222Rn)
Relative to the local meteoric water line at the IAEA station in Addis Abeba (δ2H = 7.1δ18O+11.8), stable isotope ratios of O and H in groundwater from the various zones of the basin ()) show two distinct signals (–f)) that comprise samples depleted in 18O (< −4 ‰) and those which are comparatively enriched in 18O (> −4 ‰). Isotopically depleted values (18O < −4 ‰) are typical of deep (>250 m) groundwaters in the East African Rift (Craig et al. Citation1977, Kebede et al. Citation2007, Bretzler et al. Citation2011)) and are observed in (1) deep (>250 m) and thermal wells in the Upper Awash Basin around Addis Abeba ()); (2) deep wells in the Wolenchiti graben ()); (3) deep wells around Lake Beseka ()); and (4) deep wells and thermal springs in the Lower Awash plains (Tendaho graben, )). Data on groundwater residence times are limited. Studies to date estimate residence times of these deep groundwaters to be more than 1000 years (Kebede et al. Citation2007, Bretzler et al. Citation2011).
Figure 3. The 18O-2H plot of surface and groundwaters from selected spots in the Awash Basin; (a) shows map regions for which isotope plots (b–f) are made. LMWL = local meteoric water line, TS = thermal spring, Sp = spring, BH = borehole
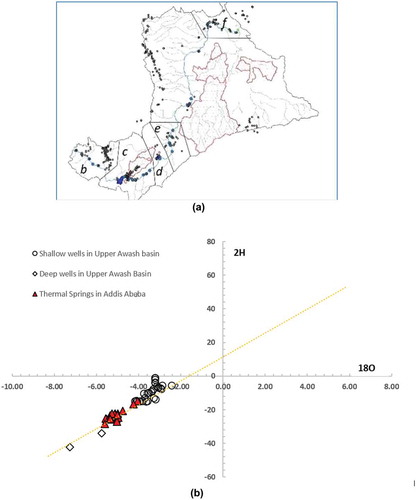
At four specific localities in the River Awash Basin (Addis Abeba, Sodere, Meteka, Tendaho; see ), 18O and 2H depleted groundwater discharges to the surface as thermal springs to the River Awash or to its tributaries. 2H-18O cross plots display the following characteristics:
(1) In the Upper Awash (including the Addis Abeba region), deep groundwaters and thermal springs are depleted in heavy isotopes, differing from shallow (<150 m) wells which are comparatively enriched in heavy isotopes ()).
(2) Between Lake Koka and Sodere, isotopically depleted waters occur in deep boreholes in Wolenchiti graben, whereas thermal springs plot along a mixing line between deep groundwaters and water of the River Awash ()), and shallow wells have similar composition to surface waters.
(3) Around Lake Beseka and Metahara plantation, isotopically depleted waters occur in deep wells, but shallow boreholes show similar isotopically enriched composition to the River Awash ()). Lake Beseka water shows the highest 18O-2H enrichment owing to evaporative water loss. The lake, the shallow wells and the River Awash plot along the local evaporation line (LEL), signifying similar origin of the waters. Deep boreholes plot off the LEL, suggesting the lake is not supplied by these. Shallow wells in the catchment of Lake Beseka, showing 18O-2H enrichment, plot along the LEL, which is consistent with an origin from excess irrigation water from the plantations.
(4) At Meteka, thermal springs show similar isotopic composition to groundwater of the Alidegie aquifer ()) suggesting the two have a similar origin.
(5) Between Mille and Afambo (Lower Awash), 18O-2H depleted waters are observed in deep wells at Decioto (just outside the basin) and Tendaho ()). The thermal springs and thermal wells show signs of a positive oxygen shift owing to isotopic exchange with silicate minerals at high temperature. Projecting the composition of these waters back to the local meteoric water line shows the initial compositions of the waters were depleted before undergoing a positive oxygen shift (i.e. enrichment in 18O without commensurate enrichment in 2H as the result of 18O exchange between water with silicates at high temperature). The 18O-2H composition of the shallow wells and the River Awash waters are indistinguishable at this site, implying the origin of the shallow wells is leakage from the River Awash.
At all locations adjacent to the River Awash, shallow boreholes and thermal springs reflect evidence of the influence of the River Awash, as witnessed by enrichment in heavy isotopes (18O, 2H). At Beseka, a region characterized by a dense network of fractures including new actively opening ground fissures (Williams et al. Citation2004), the isotopically enriched signal of the River Awash is visible in adjacent aquifers. This influence could be the outcome of leakage from irrigation canals rather than leakage from the river itself. At the start of the irrigation scheme, Lake Beseka was a small pocket of hypersaline water in a closed depression – showing no sign of major groundwater movement at shallow levels.
Headwater tributaries of the River Awash contain appreciable amounts of 222Rn in both the dry and wet seasons (). The wet-season 222Rn is lower than 200 Bq m−3 at all downstream locations. In the dry season, the river water shows higher 222Rn content in headwater tributaries and proximal to irrigation areas between Wonji and Sodere as well as between Metahara and Meteka, owing to inflow of excess irrigation water via the subsurface. Discharge of shallow aquifers in the upstream areas to the river during the wet and dry seasons is the likely cause of the high 222Rn content in both seasons. 222Rn is nearly below the detection limit in the Lower Awash delta (between Mile and Lake Afambo) in both dry and wet seasons, showing little impact of regional or local inflow of groundwaters into the river.
Figure 4. Pattern of 222Rn and electrical conductivity (EC) in the main course of the River Awash: (a) wet-season 222Rn, (b) dry-season 222Rn, (c) dry-season EC, and (d) wet-season EC. 222Rn is in Bq m−3 and EC is in µS cm−1
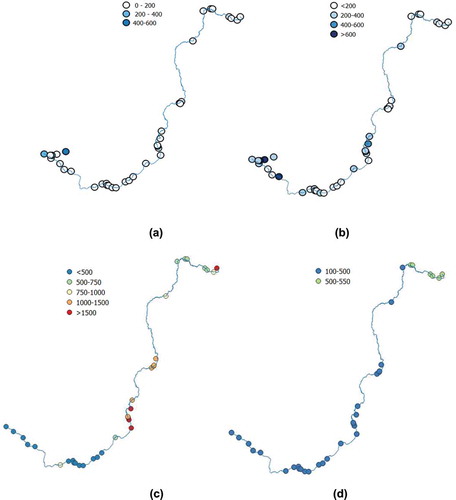
The 222Rn pattern in Lake Beseka () shows substantial spatial variation. The southern, eastern and north-western sectors of the lake show high 222Rn contents. In the north and north-east the 222Rn content falls below the detection limit. This pattern mirrors lake surface temperature (Goerner et al. Citation2009), in which warmer waters are associated with higher 222Rn. The fact that the high 222Rn content comes from the specified zones of the lake, and corresponds to the direction in which intensive irrigation is taking place, provides a plausible indication that irrigation activity is the main source of the lake water. This deduction is supported by isotopic data ()). The lake does not show a high 222Rn content in the northern part where active fractures are common, suggesting there is no link between the fissures and groundwater input to the lake from the northern sector.
4.2 Electrical conductivity
The EC of the River Awash water shows a gradual increase from its headwaters to its terminus () in both seasons. In the wet season, the EC of the river water is lower than 550 µS cm−1 at all locations. A sharp rise in EC is evident during the dry season, downstream of Lake Beseka. A small increase in EC is detected in the dry season in the headwater region upstream of the Lake (reservoir) Koka (see the sixth station downstream from the headwaters, )), which may arise from a polluted tributary, the Akaki River, draining Addis Abeba (EPHI Citation2017). The sharp rise in EC in the Middle Valley (Beseka to Mille) in the dry season is related to the inflow of the now-brackish water overflowing from Lake Beseka to the River Awash. Between Lake Beseka and Mille station, the EC of the river gradually decreases from 1800 to 830 µS cm−1, owing to the inflow of freshwater from the Gadabasa wetland located in the Middle Awash Valley, as well as to the tributary inflow from the western escarpment. Dry-season EC decreases to 660 µS cm−1 downstream of the Tendaho reservoir, related to mixing with the dilute water of this reservoir. High dry-season EC between Beseka and Meteka makes waters from the River Awash unsuitable for non-salt tolerant crop irrigation.
EC in groundwater is extremely variable. Aquifers in the Upper Awash show EC values between 200 and 2000 µS cm−1, which gradually increase towards the Rift. Locally, groundwaters with extremely high salinity (EC > 10 000 µS cm−1) are encountered in the piezometers in irrigation areas as well as in the shallow wells in Tendaho graben of the Awash Delta. High EC in the groundwater within irrigation areas is attributed to evaporative enrichment as well as dissolution of salts from evaporites in loose alluvial sediments in the Awash Delta. The highest EC value at the terminal lake Afambo ()) is the result of evaporative concentration of waters of the River Awash.
4.3 Shallow piezometers in irrigation areas
Piezometric measurements have been recorded in three main irrigation areas (Wonji, Metahara, and Melka Worer-Melka Sedi) since the 1970s, following the adoption of mechanized irrigation. Water-level measurements have been taken intermittently and manually on a monthly time step. At all three sites, piezometric records at Metahara and Melka Worer-Melka Sedi farms show a general increase in groundwater levels (). Groundwater levels at Wonji have increased by 7 m (Furi et al. Citation2011) since the start of irrigation in the late 1960s. Except for Metahara farms, hydraulic gradients run from the farmlands to the river, consistent with minor but increasing trends in EC and 222Rn content in the vicinity of irrigated farmlands.
Figure 6. (a, left) Water level changes in the Metahara plantation areas between 1988 and 2008, shown in graduated colour; numbers near the dots show the 2008 water level in metres above sea level. The blue area is Lake Beseka, and green areas are irrigation command areas. The hatching shows the new (2011) irrigation canal. (b, right) Current piezometric water level at Melka Worer-Melka Sedi farms; numbers indicate water level in metres above sea level. The inferred direction of groundwater flow and a piezometric contour map are also shown
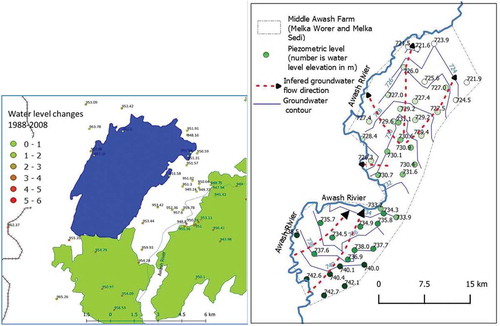
The current direction of groundwater flow is towards the lake ()) where piezometric levels are higher than the lake stage area. The highest EC (1800 µS cm−1) of the River Awash is recorded downstream of the junction of Lake Beseka outflow and the main Awash flow. At Melka Worer, current piezometric levels indicate shallow groundwater flows mainly towards the River Awash ()). Groundwater levels are shallow, often less than 3 m below ground in many parts of the irrigation area. The relatively higher 222Rn content of the River Awash at this site (shown in )) and the slight increase in EC values compared to the immediate upstream area ()) are consistent with groundwater inflow to the River Awash.
4.4 River channel water budgets
Records of river discharge along the main course of the River Awash are available from 19 stations. shows the river discharge changes along an upstream–downstream transect from the outlet at Koka Dam to Tendaho Dam from 1999 and 2001 (years with a complete monthly river discharge record at Hertale station; 1999 was a year of high flows whereas 2001 represents a low-flow year). Gaining segments coincide with major tributary inflows, but these observational data are insufficient to resolve the contribution of groundwater along these segments. Reductions in river discharge correspond to river uptake for irrigation water use. However, the water loss segment between Hartale and Mille stations is remarkable. This loss is recorded in earlier datasets of the 1980s as well (Halcrow Citation1989). The figure shows the loss between Hartaale and Mile stations is stage dependent, such that large reductions are observed during wet years. In this segment, transmission losses are ~0.5 km3. Applying the Penman equation, evaporation in the wetland environment in the region is estimated at 0.4 km3 year−1; the remaining 0.1 km3 year−1 is attributed to leakage to groundwater. The NNE-SSW trending regional graben and associated dense fracture () network are the most plausible explanations for the transmission of these flows.
4.5 Regional-scale piezometric contours
Regional piezometer maps are employed here to evaluate whether hydraulic conditions support assertions of surface water–groundwater interactions in this data-scarce environment. Of interest is whether any connection exists between the large-scale groundwater flow systems identified in and the River Awash. The following broad observations can be made from the regional piezometric contours shown in :
Hydraulic head contours follow regional structures and surface topography;
Throughout the course of the river from Melka Worer to Tendaho ( and ), hydraulic heads are below the elevation of the river bed, providing conditions consistent with a losing river;
Between Meteka and Mile stations groundwater flow is perpendicular to the river flow direction, whereas east of the river hydraulic heads are lower than the stream bed level showing a general groundwater flow towards the east – these observations confirm previous speculation that groundwater flows away from the river in a NNE direction (UNDP Citation1973) (Gasse and Street Citation1978); and
Groundwater from the Alidegie aquifer discharges via thermal springs at Gewane – this deduction disputes previous assertions that the thermal springs at Meteka originate from the Western highlands (Craig et al. Citation1977).
5 Discussion
5.1 Regional groundwater flows are largely independent of river flows
In the River Awash Basin, 18O- and 2H-depleted groundwaters have been detected in deep wells and in thermal springs connected to the deeper aquifers. These groundwaters originate from the western highlands bounding the River Awash Basin and follow deeper, regional flowpaths before they reach the rift floor (UNDP Citation1973, Schoell and Faber Citation1976, Craig et al. Citation1977). Current observations and the re-interpretation of existing data confirm that groundwaters depleted in the heavy isotopes (18O, 2H) exist at all locations beneath surveyed areas and are characteristic of the entire River Awash Basin.
A convergence of evidence from environmental tracers as well as regional- and local-scale hydraulic head contours indicate regional groundwater discharge to the River Awash in only a few locations. In the upper Awash, the tributary streams gain groundwater. In the middle and lower parts, the River Awash is predominantly a losing river. There are only a few sites where regional groundwater contributes flows to the river. These are sites where (1) geothermal upwelling favours ingress of deep groundwater into the river (Sodere, Tendaho), (2) regional groundwater flows are inhibited by impermeable rock layers that direct groundwater flow towards to the land surface (Meteka), and (3) river flow direction suddenly changes in relation to fault controls. These sites play a limited role in enhancing the salinity of the river, as previously speculated (Craig et al. Citation1977, Darling Citation1996) (). A lack of data regarding the major-ion and trace-element hydrochemistry prevents the investigation of the impact of geothermal water discharge on temperature and of individual ions of ecological significance (e.g. silica, potassium).
Overall, regional groundwater flow in the River Awash Basin contributes minimally to river flow. Where groundwater inflow to the River Awash is observed, water originates from regional flowpaths far from the site of groundwater inflow. Regional groundwater flow is of considerable importance to ecosystems and humans residing in the basin, as it sustains geothermal systems (Darling Citation1996) and supports climate resilience in arid environments (Calow et al. Citation2006) where rainfall is extremely variable (Taye et al. Citation2018). Although most regional groundwater flow is believed to have no current surface manifestations, 18O-2H and 222Rn data show that the extensive Meteka wetlands are supported by the discharge of regional groundwater flow impeded by faults. The wetlands are believed to have originated from the mixing of deep regional flow and the River Awash. Cuthbert et al. (Citation2017) postulate that regional groundwater flow has been critical in supporting isolated networks of hydro-refugia that governed hominin isolation and dispersal in the Rift Valley region.
Inflow of groundwater into the River Awash was not detected anywhere between Tendaho and Lake Abe. Along the stretch of the River Awash between the Middle Awash station and Tendaho, the river is largely losing, except at Meteka where thermal springs discharge into the river. The total discharge of the regional groundwater to springs in the Awash Basin is estimated to be 0.30 µScm-1. This flux is far lower than the recharge estimated in individual basins (). Consequently, a substantial portion of this groundwater flow does not return to riverine drainage and exits the basin via deep groundwater flows, possibly to the Red Sea or the Danakil depression outside of the River Awash Basin. Overall, results are consistent with conceptual groundwater models reported elsewhere in arid areas (e.g. Schaller and Fan Citation2009), which contend that (1) the water table falls below the upper valleys, (2) rivers are primarily losing, and (3) groundwater discharges via regional flows that can exit the basin without returning to the surface waters.
5.2 Reservoirs, wetlands and lakes need careful management to reduce water salinity
Salinization in river basins is a common problem associated with irrigation diversion and flow regulation in arid environments (Lamontagne et al. Citation2005, Scanlon et al. Citation2007), where water diverted for irrigation mobilizes salt accumulated in alluvial plains and discharges it back to the rivers through irrigation return water. In the River Awash Basin, irrigation development has had a complex impact on salinity. Despite the establishment of several irrigation schemes in the basin in the 1960s, our results show the overall salinity of the river has remained largely unchanged. The current trend and range in EC of the river are similar to the values observed in the early 1960s (1962–1964) by Pittwel (Citation1971). The EC record taken between 1962 and 1964 shows a gradual increase in value, from ~200 µS cm−1 in the upper reaches to 600 µS cm−1 in the lower reaches. We observe similar EC values in the upstream and downstream segments of the river. From our current observations, salinity increases ()) are noted along the middle section of the River Awash between Lake Beseka and the Meteka wetland. The rise in salinity in this section of the river has become a water supply threat, as the current dry-season flow is no longer suitable for irrigation or direct use for drinking water. This salinity has led some towns to change their water sources from the River Awash to groundwater sources.
The increase in salinity of the river is related to the outflow of Lake Beseka. Lake Beseka was initially a closed hypersaline lake with no surface and groundwater outflow. It has, however, expanded and now has an outlet due to irrigation-excess water entering the lake via subsurface pathways. The presence of a dense fracture network (some active fractures) and blister caves and the porous nature of the geology in the area have facilitated the flow of excess irrigation water into the lake, which has expanded to the level of flooding infrastructures and discharging saline water to the River Awash.
Downstream of Meteka wetland reservoir, the EC of the River Awash has returned to the values of the 1960s. The salinity of this downstream section of the river is kept low by large tributary inflows of freshwater coming from the western catchments as well as by inflow of freshwater from Meteka wetland, which stores water during the wet seasons and releases water to the River Awash during the dry season. Protection of the Meteka wetland and other tributaries originating from the western escarpment is thus critical to prevent increases in salinity of the River Awash downstream. Because the River Awash is losing along most of its channel, salinization of the river via groundwater inflow is limited.
5.3 Implications in water resources and water allocation modelling
The fact that the deep groundwater bodies with regional flow systems are largely disconnected from the middle and lower parts of the River Awash means there is scope for considering these two water resources independently. The possibility of identifying additional water resources in arid areas rests on finding groundwaters that are separate from surface water flows. Such systems are known to exist in many places in Africa, including for example the Zambezi region (Bäumle et al. Citation2019). Large groundwater systems in the middle and lower valleys are disconnected from the river. Hence, groundwater could potentially be developed for industrial or urban water use without substantial short-term impact on the flow of the River Awash. Currently there are plans to abstract the deep groundwaters for piped rural drinking water schemes and to export water to Djibouti. However, more research is needed to ascertain the sustainable yield of the aquifers, as recharge is limited and yields for individual wells depend on well siting.
Future surface water withdrawals from the basin need to consider their potential impact on salinity of the downstream part of the river. Tributary inflows from the western escarpment and dry-season water inflow from Gadabasa wetland to the River Awash serve to buffer the high salinity of the River Awash caused by the rise in water levels in Lake Beseka and consequent spillage into the river channel. Large-scale abstraction of water from these tributaries and the wetland in the future could lead to an increase in salinity of the River Awash. Such consequences have already been observed, as the hydrography of the marginal grabens in the western escarpment of Afar (from which the rivers emerge) is already changing from an outflow to closed drainage status because of agricultural intensification (Meaza et al. Citation2017). If tributary inflow is unable to reach the middle and lower sections of the River Awash, the intensive upstream irrigation activity will lead to a major rise in river salinity.
Hydrological models that seek to simulate the role of climate change and the human impact on hydrological systems often target how a specific change (increase in rainfall intensity, increase or decrease in diversion, increase in temperature) affects river discharge, water availability and water quality. The lesson from the River Awash Basin is that the response (direction and scale) of the system to changes in climate and human development can follow complex paths. Lake Beseka, formerly a 2.5 km2 closed hypersaline lake Total Dissolved Solids (TDS > 36 mg/L), is now a 55 km2 brackish (TDS 3 g/L) lake with outflow to the River Awash, threatening the salinization of this river downstream. Hydrological or water allocation models would not easily capture this complex path of change. In such situations, monitoring and adaptive water resources management (Mosello et al. Citation2015) may prove a viable option to complement hydrologic models. Further, hydrological models which route all groundwater to river discharge would misrepresent the water resources of this basin.
6 Conclusions
A common hydrological assumption, that topographically defined basins are self-contained whereby all groundwater returns to the surface and is routed out of the basin via surface water drainage networks, is shown not to hold in the River Awash Basin of Ethiopia, using a range of data sources including environmental tracers, hydrochemistry and piezometric data. Here, groundwater inflow is detected in tributary networks in the humid uplands, but most regional groundwater flow does not return to stream networks in the arid middle and lower reaches of the basin; thermal springs in these areas originate from regional groundwater flow systems several hundred kilometres from where they discharge. The hydrological characteristics of the middle and lower reaches River Awash Basin, confirmed by observational records, are consistent with those observed in other arid basins: (1) rivers are primarily losing and (2) regional groundwater exits the basin without returning to the surface waters or basin outlet.
Due to the substantial variations in aridity that characterize the River Awash Basin, hydrological changes in response to anthropogenic influences are complex. Increasing salinity in the middle reaches of the River Awash threatens irrigation and domestic water supply. The rise in salinity is attributed to leakage of excess irrigation water via dense fracture networks to the brackish Lake Beseka, which drains to the River Awash. This threat is mitigated somewhat by dry-season releases from natural and manmade surface water storage. Management options addressing salinity in the Awash Basin need to consider both irrigation efficiency and the protection and management of surface water storage to ensure the continuity of dry-season flows. Retaining the capacity of the River Awash to mitigate salinity through dilution will inevitably require increased irrigation efficiencies in the middle reaches of the River Awash (Metahara to Meteka) and the tributaries that feed the middle and lower reaches of the basin. Future extreme drought events could reduce the ability of the River Awash to mitigate salinity, leading to the salinization of soil and water downstream. It is vital that these dynamics and the anomalous characteristics of the River Awash Basin are explicitly considered in models and plans assessing the threats posed by climate change and further development. It is noteworthy that the regionally extensive aquifers in the middle and lower reaches of the basin offer potential sources of water for water supply as their exploitation is not expected to impact surface flows.
Acknowledgements
This work was conceived as part of UNICEF’s strategy of climate-resilient rural water supply which is intended to rely on extracting regional groundwater bodies to supply rural communities through reticulated pipe schemes. The data used in this paper derive from various sources, including the Hidden Crisis project under the NERC-ESRC-DFID UPGro Programme. Collection of primary isotope and radon data was completed as part of the REACH programme funded by the UK Department for International Development (DFID) for the benefit of developing countries (Aries Code 201880). RGT acknowledges support from NERC-ESRC-DFID UPGro GroFutures (Ref. NE/M008932/1). The views expressed and information contained herein are those of the authors alone.
Disclosure statement
No potential conflict of interest was reported by the authors.
References
- Adeba, D., Kansal, M.L., and Sen, S., 2015. Assessment of water scarcity and its impacts on sustainable development in Awash basin, Ethiopia. Sustainable Water Resources Management, 1 (1), 71–87. doi:10.1007/s40899-015-0006-7
- Alemayehu, T., Ayenew, T., and Kebede, K., 2006. Hydrogeochemical and lake level changes in the Ethiopian rift. Journal of Hydrology, 316 (1), 1–11.
- Ayenew, T., Demlie, M., and Wohnlich, S., 2008a. Hydrogeological framework and occurrence of groundwater in the Ethiopian aquifers. Journal of African Earth Sciences, 52 (3), 97–113. doi:10.1016/j.jafrearsci.2008.06.006
- Ayenew, T., Kebede, S., and Alemyahu, T., 2008b. Environmental isotopes and hydrochemical study applied to surface water and groundwater interaction in the Awash. Hydrological Processes: An International Journal, 1563 (10), 1548–1563. doi:10.1002/hyp.6716
- Barthel, R. and Banzhaf, S., 2016. Groundwater and surface water interaction at the regional-scale – a review with focus on regional integrated models. Water Resources Management, 30 (1), 1–32. doi:10.1007/s11269-015-1163-z
- Battistelli, A., et al., 2002. Reservoir engineering assessment of Dubti geothermal field, Northern Tendaho rift, Ethiopia. Geothermics, 31 (3), 381–406. doi:10.1016/S0375-6505(01)00039-6
- Bäumle, R., Himmelsbach, T., and Noell, U., 2019. Hidrogeologia e geoquímica de um aquífero tectonicamente controlado, profundamente assentado e semifóssil na Região do Zambeze (Namíbia). Hydrogeology Journal, 27 (3), 885–914. doi:10.1007/s10040-018-1896-x
- Belay, A.E., 2010. Growing lake with growing problems: integrated hydrogeological investigation on Lake Beseka, Ethiopia. Ph.D. Thesis. Universitäat Bonn, Bonn, Germany.
- Beyene, K., 2000. Chemical and isotopic studies of geothermal prospects, in the Southern Afar region, Ethiopia. Tohoku: Proceedings World Geothermal Congress, 2000, 977–983.
- Bierkens, F.P.B., 2015. Water resources research. Global Hydrology 2015: State, Trends, and Directions Marc, 51, 4923–4947.
- Bretzler, A., et al., 2011. Groundwater origin and flow dynamics in active rift systems – a multi-isotope approach in the main Ethiopian rift. Journal of Hydrology, 402 (3–4), 274–289. doi:10.1016/j.jhydrol.2011.03.022
- Calow, R., et al., 2006. Drought, water security and rural livelihoods Drought, water security and rural livelihoods. British Geological Survey Commissioned Report, CR/02/226N.
- Cartwright, I., et al., 2014. Understanding parafluvial exchange and degassing to better quantify groundwater inflows using 222Rn: the King River, southeast Australia. Chemical Geology, 380, 48–60. doi:10.1016/j.chemgeo.2014.04.009
- Cartwright, I. and Gilfedder, B., 2015. Mapping and quantifying groundwater inflows to deep creek (Maribyrnong catchment, SE Australia) using 222Rn, implications for protecting groundwater-dependant ecosystems. Applied Geochemistry, 52, 118–129. doi:10.1016/j.apgeochem.2014.11.020
- Cartwright, I. and Hofmann, H., 2015. Using radon to understand parafluvial flows and the changing locations of groundwater inflows in the Avon River, southeast Australia. Hydrology and Earth System Sciences, 20 (9), 3581–3600. doi:10.5194/hess-20-3581-2016
- Condon, L.E., et al., 2020. Where is the bottom of a watershed ? Water Resources Research, 56 (3). doi:10.1029/2019WR026010
- Cook, P.G., et al., 2006. Quantifying groundwater discharge to Cockburn River, southeastern Australia, using dissolved gas tracers 222Rn and SF6. Water Resources Research, 42 (10), 1–12. doi:10.1029/2006WR004921
- Cook, P.G., et al., 2008. Groundwater inflow to a shallow, poorly-mixed wetland estimated from a mass balance of radon. Journal of Hydrology, 354 (1–4), 213–226. doi:10.1016/j.jhydrol.2008.03.016
- Cook, P.G., 2015. Quantifying river gain and loss at regional scales. Journal of Hydrology, 531, 749–758. doi:10.1016/j.jhydrol.2015.10.052
- Craig, H., Lupton, J.E., and Horowitz, R.M., 1977. Isotopic geochemistry and hydrology of geothermal waters in the Ethiopian rift valley. La Jolla, San Diego: Scripps Institution of Oceanography, University of California.
- Cuthbert, M.O., et al., 2017. Modelling the role of groundwater hydro-refugia in East African hominin evolution and dispersal. Nature Communications, 8 (1), 1–11. doi:10.1038/ncomms15696
- Dai, A. and Trenberth, K.E., 2002. Estimates of freshwater discharge from continents: latitudinal and seasonal variations. Journal of Hydrometeorology, 3 (6), 660–687. doi:10.1175/1525-7541(2002)003<0660:EOFDFC>2.0.CO;2
- Darling, W.G., 1996. The geochemistry of fluid processes in the eastern branch of the East African rift system. Thesis (PhD). British Geological Survey, UK
- Darling, W.G., Gizaw, B., and Arusei, M.K., 1996. Lake-groundwater relationships and fluid-rock interaction in the East African rift valley: isotopic evidence. Journal of African Earth Sciences, 22 (4), 423–431. doi:10.1016/0899-5362(96)00026-7
- Dimova, N.T. and Burnett, W.C., 2011. Evaluation of groundwater discharge into small lakes based on the temporal distribution of radon-222. Limnology and Oceanography, 56 (2), 486–494. doi:10.4319/lo.2011.56.2.0486
- Durrige Company Inc, 2020. Avialable from: https://durridge.com/products/big-bottle-system/
- EPHI, 2017. Pollution status of Akaki River and its contamination effect on surrounding environment and agricultural products. Ministry of Health Report, Addis Ababa, Ethiopia.
- Essaid, H.I. and Caldwell, R.R., 2017. Evaluating the impact of irrigation on surface water – groundwater interaction and stream temperature in an agricultural watershed. Science of the Total Environment, 599–600, 581–596. doi:10.1016/j.scitotenv.2017.04.205
- Furi, W., et al., 2011. The hydrogeology of Adama-Wonji basin and assessment of groundwater level changes in Wonji wetland, main Ethiopian rift: results from 2D tomography and electrical sounding methods. Environmental Earth Sciences, 62 (6), 1323–1335. doi:10.1007/s12665-010-0619-y
- Gasse, E. and Street, F.A., 1978. Late quaternary lake-level fluctuations and environments of the northern rift valley and Afar region (Ethiopia and Djibouti). Palaeogeography, Palaeoclimatology, Palaeoecology, 24 (4), 279–325. doi:10.1016/0031-0182(78)90011-1
- Gasse, F. and Fontes, J.-C., 1989. Palaeoenvironments and palaeohydrology of a tropical closed lake (Lake Asal, Djibouti) since 10,000 yr B.P. Palaeogeography, Palaeoclimatology, Palaeoecology, 69, 67–102. doi:10.1016/0031-0182(89)90156-9
- Gibson, J.J., Birks, S.J., and Yi, Y., 2016. Stable isotope mass balance of lakes: a contemporary perspective. Quaternary Science Reviews, 131, 316–328.
- Goerner, A., Jolie, E., and Gloaguen, R., 2009. Non-climatic growth of the saline Lake Beseka, main Ethiopian rift. Journal of Arid Environments, 73 (3), 287–295. doi:10.1016/j.jaridenv.2008.09.015
- Halcrow, 1989. Master plan for the development of surface water resources in the Awash basin. Unpublished Report by Halcrow Company report. Addis Ababa, Ethiopia.
- Kafri, U. and Yechieli, Y., 2012. The relationship between current and paleo groundwater base-levels. Quaternary International, 257, 83–96. doi:10.1016/j.quaint.2011.08.028
- Kalbus, E., Reinstorf, F., and Schirmer, M., 2006. Measuring methods for groundwater – surface water interactions: a review. Hydrology and Earth System Sciences, 10 (6), 873–887. doi:10.5194/hess-10-873-2006
- Kebede, S., et al., 2016. Interaction of surface water and groundwater in the Nile basin: isotopic and piezometric evidence. Hydrogeology Journal, 25, 707–726.
- Kebede, S., Travi, Y., and Asrat, A., 2007. Groundwater origin and fl ow along selected transects in Ethiopian rift volcanic aquifers. Hydrogeology Journal, 16, 55–73.
- Kebede, S., Travi, Y., and Rozanski, K., 2009. The d 18 O and d 2 H enrichment of Ethiopian lakes. Journal of Hydrology, 365 (3–4), 173–182.
- Kebede, S. and Zewdu, S., 2019. Origin of water and in quantifying groundwater inflow rates in an alarmingly growing. Water, 11, 2591. doi:10.3390/w11122591
- Kluge, T., et al., 2012. Localising and quantifying groundwater inflow into lakes using high-precision Rn profiles. Journal of Hydrology, 450–451, 70–81.
- Konikow, L.F. and Bredehoeft, J.D., 1974. Modeling flow and chemical quality changes in an irrigated stream–aquifer system. Water Resources Research, 10 (3), 546–562.
- Koriche, S.A. and Rientjes, T.H.M., 2016. Application of satellite products and hydrological modelling for flood early warning. Physics and Chemistry of the Earth, 93, 12–23.
- Lamontagne, S., Leaney, F.W., and Herczeg, A.L., 2005. Groundwater-surface water interactions in a large semi-arid floodplain: implications for salinity management. Hydrological Processes, 19 (16), 3063–3080.
- Leblanc, M., et al., 2012. A review of historic and future hydrological changes in the Murray-Darling basin. Global and Planetary Change, 80–81, 226–246.
- Mcdonald, A.K., et al., 2013. Studies of a regulated dryland river: surface-groundwater interactions. Hydrological Processes, 27 (12), 1819–1828.
- Meaza, H., et al., 2017. Natural resource opportunities and challenges for rural development in marginal grabens – the state of the art with implications for the rift valley system in Ethiopia. Journal of Arid Environments, 147, 1–16.
- Mosello, B., et al., 2015. Building adaptive water resources management in Ethiopia. London: Overseas Development Institute.
- Mullinger, N.J., et al., 2009. Controls on the spatial and temporal variability of222Rn in riparian groundwater in a lowland Chalk catchment. Journal of Hydrology, 376 (1–2), 58–69.
- Peterson, R.N., et al., 2008. Radon and radium isotope assessment of submarine groundwater discharge in the Yellow River delta, China. Journal of Geophysical Research: Oceans, 113 (9), 1–14.
- Pitwell, L.R., 1971. Analysis of Ethiopian and other natural waters. Addis Ababa University Report, Addis Ababa, Ethiopia.
- Pürschel, M., Gloaguen, R., and Stadler, S., 2013. Geothermal activities in the main Ethiopian rift: hydrogeochemical characterization of geothermal waters and geothermometry applications (Dofan-Fantale, Gergede-Sodere, Aluto-Langano). Geothermics, 47, 1–12.
- Rodellas, V., et al., 2012. Quantifying groundwater discharge from different sources into a Mediterranean wetland by using 222Rn and Ra isotopes. Journal of Hydrology, 467, 11–22.
- Scanlon, B.R., et al., 2007. Global impacts of conversions from natural to agricultural ecosystems on water resources: quantity versus quality. Water Resources Research, 43 (3), 1–18.
- Schaller, M.F. and Fan, Y., 2009. River basins as groundwater exporters and importers : implications for water cycle and climate modeling. Journal of Geophysical Research: Atmospheres, 114, 1–21.
- Schmidt, A., et al., 2008. Continuous and discrete on-site detection of radon-222 in ground- and surface waters by means of an extraction module. Applied Radiation and Isotopes, 66 (12), 1939–1944.
- Schmidt, A. and Schubert, M., 2007. Using radon-222 for tracing groundwater discharge into an open-pit lignite mining lake - A case study. Isotopes in Environmental and Health Studies, 43, 387–400.
- Schoell, M. and Faber, E., 1976. Survey on the isotopic composition of waters from NE Africa. Geologisches Jahrbuch, 17, 197–213.
- Simpson, H.J. and Herczeg, A.L., 1991. Salinity and evaporation in the River Murray Basin, Australia. Journal of Hydrology, 124 (2), 1–27.
- Taye, M.T., et al., 2018. Climate change impact on water resources in the Awash basin, Ethiopia. Water, 10 (11), 1–16.
- Telford, R.J. and Lamb, H.F., 1999. Groundwater-mediated response to holocene climatic change recorded by the diatom stratigraphy of an Ethiopian crater lake. Quaternary Research, 52 (1), 63–75.
- UNDP, 1973. Geology, geochemistry and hydrology of hot springs of the East African rift system within Ethiopia. United Nations Development Program report, New York.
- UNFAO, 1965. Report on survey of the Awash River basin. Rome: Food and Agriculture Organization of the United Nations.
- Williams, F.M., Williams, M.A.J., and Aumento, F., 2004. Tensional fissures and crustal extension rates in the northern part of the main Ethiopian rift. Journal of African Earth Sciences, 38 (2), 183–197.
- Winter, T.C., 1995. Recent advances in understanding the interaction of groundwater and surface-water. Reviews of Geophysics, 33 (2), 985–994.
- Wolski, P. and Savenije, H.H.G., 2006. Dynamics of floodplain-island groundwater flow in the Okavango Delta, Botswana. Journal of Hydrology, 320 (3–4), 283–301.
- Yoneda, M., Inoue, Y., and Takine, N., 1991. Location of groundwater seepage points into a river by measurement of 222Rn concentration in water using activated charcoal passive collectors. Journal of Hydrology, 124 (3–4), 307–316.