Abstract
Within the last decade, nanoscale lipid bilayers have emerged as powerful experimental systems in the analysis of membrane proteins (MPs) for both basic and applied research. These discoidal lipid lamellae are stabilized by annuli of specially engineered amphipathic polypeptides (nanodiscs) or polymers (SMALPs/Lipodisqs®). As biomembrane mimetics, they are well suited for the reconstitution of MPs within a controlled lipid environment. Moreover, because they are water-soluble, they are amenable to solution-based biochemical and biophysical experimentation. Hence, due to their solubility, size, stability, and monodispersity, nanoscale lipid bilayers offer technical advantages over more traditional MP analytic approaches such as detergent solubilization and reconstitution into lipid vesicles. In this article, we review some of the most recent advances in the synthesis of polypeptide- and polymer-bound nanoscale lipid bilayers and their application in the study of MP structure and function.
Keywords:
Introduction
The lamellar bilayer of biological membranes is an aggregate of lipids stabilized by noncovalent interactions. A given biomembrane is composed of a defined collection of lipids, each with distinct features, including molecular geometry (cylindrical, cone, and inverted cone), head group identity (variable size and charge distribution), and acyl chain properties (variable lengths and degrees of saturation). Together, these attributes define fundamental features of the bilayer such as lateral pressure, polarity, and lipid tail mobility profiles (Figure ) as well as elastic properties, curvature, and polymorphic behavior. The lipid bilayer is also the natural milieu of membrane proteins (MPs). In the broadest sense, this class of proteins includes integral MPs (those that contain one or more hydrophobic transmembrane spanning segments), peripheral MPs (those that associate, often reversibly, at the bilayer surface with lipids or integral MPs through electrostatic and/or hydrophobic interactions), and lipid-anchored proteins (those that are covalently modified with acyl chains or isoprenoid groups, which stably attach to a bilayer leaflet). MPs comprise 20–30% of the proteome of any given organism (Wallin & von Heijne, Citation1998), yet on an individual basis, natural membranes vary considerably with respect to protein content. The myelin sheath is up to 30% protein, a typical eukaryotic plasma membrane is approximately 50% protein, and the energy-conserving membranes of thylakoids and mitochondria contain an astonishing 75% protein by mass. Together, lipids and proteins serve to carry out the specialized biological function of each membrane, including compartmentalization and maintenance of selective permeability, ion and metabolite transport, signal and energy transduction, and cell recognition.
Figure 1. The lamellar lipid bilayer of biological membranes is an anisotropic arrangement of lipid monolayers with many physiochemical gradients along the bilayer normal (z-axis), as shown for this representation of a phosphatidylcholine bilayer. (A) Bilayer dimensions. The thickness of a typical hydrocarbon core, defined by the alkyl chains of both leaflets, is ~ 3 nm. The thermal thickness of the combined interfacial regions (polar head groups and waters of hydration) comprise roughly half of the bilayer thickness, (B) Lateral pressure profile. Bilayer pressure (π) is composed of repulsive lateral pressure profiles in the headgroup and acyl chain regions and interfacial tension at the polar/nonpolar interface originating from the hydrophobic effect (Marsh, Citation2007), (C) Dielectric profile. The multilayer dielectric (ε) profile includes a very low dielectric core in the hydrocarbon center flanked by regions of intermediate dielectric followed by a steep gradients in the polar headgroup region toward high ε values similar to bulk water (Nymeyer & Zhou, Citation2008), (D) Acyl chain order profile of hydrocarbon chains. Measurements of segmental order parameter (S) reveal consistently high order for several chain segments near the headgroup followed by progressively greater disorder (lower anisotropy) toward the bilayer center (Seelig & Seelig, Citation1974), and (E) Time-averaged probability distributions of water and principal lipid structural groups for the thermally disordered fluid bilayer in the liquid crystalline phase, based on X-ray and neutron scattering profiles (phos, phosphate; glyc, glycerol backbone) (White, Ladokhin, Jayasinghe, & Hristova, Citation2001).
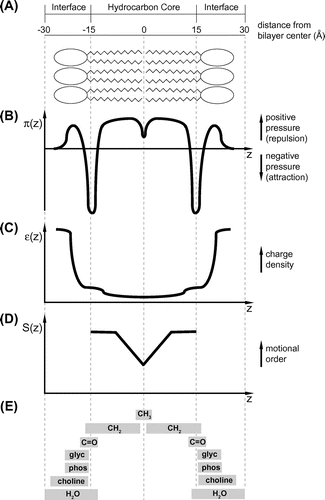
For solution-based structural and functional studies, individual MPs or protein complexes must be isolated from the membrane. This presents a technical challenge, as the hydrophobic surfaces of MPs are aggregation-prone in water due to the hydrophobic effect, which in this case maximizes solvent entropy by minimizing the contact area of water with nonpolar molecules. The solubilization of membranes with nonionic surfactants (detergents in the vernacular) has traditionally been the means by which MPs were isolated and characterized. Detergents commonly used for this purpose include DDM, Triton X-100, and those of the Brij series. Indeed, the nonpolar domains of detergents shield the aggregation-sensitive regions of MPs; however, the resulting micellar structures do not recapitulate the physiochemical properties of the lamellar bilayer from which the proteins were extracted (Figure ). Hence, MPs in detergent micelles are prone to altered conformational dynamics, misfolding, and loss of function (Seddon, Curnow, & Booth, Citation2004). By contrast, liposomes, vesicles composed of synthetic lipid bilayers, are good mimetics of the native lamellar membrane. However, the large size of proteoliposomes (MP-containing liposomes) makes them unsuitable for many spectroscopic techniques due to light scatter and low rotational mobility; they offer poor control over the oligomeric state of the MP, leading to inhomogeneous samples; and their vesicular nature precludes experimental access to both sides of the bilayer. To circumvent these issues, researchers have employed bicelles, phospholipid bilayers encircled by a rim of high-curvature short chain lipids or surfactants. Bicelles provide the lamellar environment of a native membrane while being small enough for techniques such as solution NMR. However, bicelles can be inherently unstable and the specific combination of lipids required for their synthesis may not be commensurate with the lipid requirements of the reconstituted MP.
The most recent advances in the development of model membrane systems have been with nanoscale bilayers that are stabilized by annuli of amphipathic proteins or copolymers. These experimental systems allow for the reconstitution of MPs and protein complexes into stable and monodisperse lamellar bilayers of precisely defined lipid composition. In this article, we review current progress in the development of these nanoscale bilayers and highlight key examples of their use in the investigation of MP structure and function using solution-based biochemical and biophysical techniques.
The experimental systems
Nanodiscs
Nanodiscs, developed originally by Stephen Sligar and colleagues, are discoidal patches of lipid bilayer stabilized by membrane scaffold protein (MSP) (Figure (A)). The Sligar group genetically engineered these scaffolding proteins based on the sequential amphipathic α-helices at the C-terminal lipid-binding domain of apolipoprotein A-1, whose physiological role is to form high-density lipoprotein particles involved in reverse cholesterol transport. Incubation of MSP with mixed micelles of lipid and detergent (typically cholate) at optimized molar ratios, followed by the selective removal of detergent using dialysis or hydrophobic adsorbents (e.g. BioBeadsTM), allows for nanodisc self-assembly (Bayburt, Grinkova, & Sligar, Citation2002; Bayburt & Sligar, Citation2003). The lipids (as single species or mixtures) form discs bound by two copies of MSP in which the hydrophobic faces of the amphipathic helices make contact with the acyl chains of each leaflet and the hydrophilic faces of the helices are oriented toward the polar solvent. Because the MSP length determines the circumference of the resulting nanodiscs, it was shown early on that disc diameter could be modulated by the selective addition or removal of α-helical segments from the MSP (Denisov, Grinkova, Lazarides, & Sligar, Citation2004); to date, nanodiscs with diameters ranging from 8 to 17 nm have been reported using MSPs of different lengths. The tunable nature of nanodisc diameter is an important feature, as it allows MPs with different numbers of transmembrane segments and oligomeric states to be reconstituted along with lipids. The structure and composition of nanodiscs has been confirmed using a host of techniques including small-angle X-ray scattering (SAXS), atomic force microscopy, direct measurements of lipid to MSP stoichiometry, and molecular dynamics (Schuler, Denisov, & Sligar, Citation2013). Depending on the hydrocarbon chain and lipid cross-sectional area, the thickness and total numbers of lipids per nanodisc match the dimensions expected of a pure lipid bilayer (Figure (A)). Furthermore, the structural homogeneity of nanodisc preparations has recently been corroborated by native mass spectrometry (Marty et al., Citation2012) and by small-angle scattering measurements (Kynde et al., Citation2014). Finally, nanodisc bilayers recapitulate the polymorphic behavior of native membranes; for example, the gel to liquid crystalline phase transition is similar to that of pure lipid bilayers, although broadened and shifted higher by a few degrees owing to MSP interactions with lipids at the perimeter (Denisov, McLean, Shaw, Grinkova, & Sligar, Citation2005). A wide range of MPs with differing topologies and oligomeric states have been functionally reconstituted into nanodics, as recently reviewed (Schuler et al., Citation2013). The fact that nanodiscs maintain the fold and native stability of MPs is supported by a recent single-molecule atomic force microscopy study analyzing discrete mechanical unfolding transitions of bacteriorhodopsin (bR). This protein displayed the same unfolding intermediates regardless of whether it was extracted from the native purple membrane or from DMPC-containing nanodiscs, underscoring the utility of these model bilayers in studies of MP folding and bilayer interactions (Zocher et al., Citation2012).
Figure 2. Nanoscale lipid bilayers. (A) Nanodiscs (left, upper panel) are lipid discoids bound by two copies of the MSP polypeptide, each one containing amphipathic α-helices (11 or 22 residues in length) that are separated by proline and glycine residues. Commonly used MSP variants include MSP1 (with 10 amphipathic helices, Ø – 9.8 nm) and MSP1E1 (Ø – 10.6 nm), MSP1E2 (Ø – 11.9 nm), and MSP1E3 (Ø – 12.9 nm), containing one, two, and three 22-mer helical inserts in the center of the MSP1 unit, respectively (Denisov et al., Citation2004; Schuler et al., Citation2013). Left, lower panel, electron micrograph of MSP1E3-bound nanodiscs at a magnification of 180,000 x (Schwall et al., Citation2012). Right panels, top-down schematics showing that the number of lipids per nanodisc depends on the MSP length and the cross-sectional area occupied by each lipid. MSP1 nanodiscs are approximately 10 nm in diameter, producing a lipid bilayer disc with a diameter of 8 nm (bilayer area = 50.2 nm2). In the liquid crystalline phase, the disaturated lipid 1,2-dipalmitoyl-sn-glycero-3-phosphocholine (DPPC, 16:0/16:0 PC) has an area of 0.54 nm2 and the monounsaturated lipid 1-palmitoyl-2-oleoyl-sn-glycero-3-phosphocholine (POPC, 16:0/18:1 PC) has an area of 0.70 nm2. In agreement with experimental measurements, MSP1 discs contain approximately 78 DMPC lipids and 68 POPC lipids per leaflet. The bilayer thickness of nanodiscs containing DPPC or POPC, measured by SAXS, is ~5.6 nm and ~4.6 nm, respectively, and (B) SMALPs/Lipodisqs® (upper panel) are lipid discoids bound by the styrene maleic acid copolymer containing styrene and maleic acid groups in molar ratios of 3:1 or 2:1. Lower panel, electron micrograph of 3:1 SMA-bound discs at a magnification of 120,000× (Long et al., Citation2013).
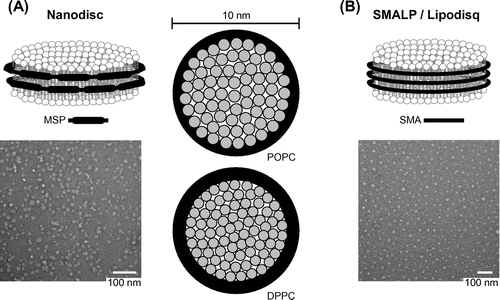
SMALPs/Lipodisqs®
More recently, lipid bilayers stabilized by rings of the copolymer styrene maleic acid (SMA) have been developed under the nomenclature styrene maleic acid lipid particles (SMALPs [Knowles et al., Citation2009]) or Lipodisqs® (Orwick et al., Citation2012; Orwick-Rydmark et al., Citation2012) (Figure (B)). The amphipathic SMA polymer with hydrophobic (styrene) and hydrophilic (maleic acid) pendant groups in molar ratios of 3:1 or 2:1 have been shown to solubilize lipids from DMPC vesicles, forming monodisperse lipid-polymer discs 9–10 nm in diameter. Lipids at the perimeter of 3:1 SMA-stabilized lipid particles are likely to be motionally constrained, contacting the styrene and maleic acid groups at the alkyl chain and headgroup regions, respectively (Orwick et al., Citation2012). Although the polymer belt imposes lateral pressure on the bilayer and decreases the cooperativity of the gel to liquid crystalline phase transition, the motional freedom of acyl chains at the bilayer core appears unperturbed (Orwick et al., Citation2012). As a means of reconstituting MPs into soluble discs, the SMA copolymer has been shown to extract both α-helical and β-barrel proteins directly from DMPC vesicles into polymer-bound particles that contained both the protein and lipid, and retained native protein function (Knowles et al., Citation2009; Orwick et al., Citation2012; Orwick-Rydmark et al., Citation2012). This approach allows for the single-step extraction of MPs directly from bilayers in a manner that obviates the requirement for detergents; therefore, there is no potentially problematic step involving detergent removal prior to disc formation. Moreover, because bilayers are stabilized by polymer, not polypeptide, there is no additional protein signal that must be deconvoluted from the target protein during analysis. On the other hand, in contrast to nanodiscs, the lipid content of the polymer-bound discs is constrained to that of the originating membrane. Moreover, as of yet, there is no clear means of systematically adjusting the diameters of SMA-bound particles based on polymer size alone.
Methods for MP reconstitution
MP reconstitution into nanodiscs
Traditional methods for the reconstitution of MPs into nanodiscs entail the in vivo expression and subsequent purification of the target protein or protein complex, which is kept soluble in a compatible detergent and included in the nanodisc self-assembly reaction. By this approach, a wide range of mild detergents can be used to maintain the solubility of MPs prior to nanodisc assembly. Indeed, it was recently demonstrated that polytopic MPs that were denatured even in an aggressive ionic detergent-like SDS were able to properly refold in the nanodisc self-assembly reaction following detergent removal (Shenkarev et al., Citation2013).
Recent advances in heterologous cell-free (CF) expression systems, however, are presenting exciting new approaches for the preparative scale (milligram quantity) production of MPs and their cotranslational reconstitution into membrane mimetics (Junge et al., Citation2011). Several groups have successfully used CF systems to incorporate MPs into nanodiscs that were pre-assembled and added to the translation reaction (Katzen et al., Citation2008; Lyukmanova et al., Citation2012; Yang, Cirico, Katzen, Peterson, & Kudlicki, Citation2011) or to co-express the target protein and MSP in the presence of phospholipids, thereby enabling the in situ assembly of the MP-containing nanodisc (Cappuccio et al., Citation2008). The technical advantages of using such CF expression systems are manifold: (i) the potential cytotoxicity and subsequent low yields associated with in vivo expression of some MPs is avoided; (ii) there is no requisite detergent solubilization of the target MP; (iii) CF expression systems are open, facilitating the addition of cofactors and site-specific labeling of the MP with reporters such as fluorescent and EPR probes for upstream analyses. Some recent studies illustrate the range of MPs with different topologies that can be cotranslationally inserted into nanodiscs using CF systems. These include the homodimeric membrane domain of the receptor tyrosine kinase ErbB3, the voltage-sensing domain of the KvAP K+ channel, and bR (Lyukmanova et al., Citation2012); the bacterial proton pump proteorhodopsin, the multidrug resistance transporters EmrE and SugE, and the phosphor-MurNAc-pentapeptide translocase MraY (Roos et al., Citation2012); and the human endothelin A and B receptors (Proverbio et al., Citation2013). In comparison with other membrane mimetics (detergents, bicelles, and liposomes), nanodiscs consistently enhanced the yield and solubility of these translated proteins. These studies also highlight the importance of nanodisc size and lipid content in promoting efficient MP incorporation. For example, the MraY translocase, containing 10 transmembrane helices, required larger diameter nanodiscs and the presence of anionic phospholipids for efficient integration and activity. In the absence of protein integration machinery, the mechanism by which MPs are incorporated into such pre-assembled model membranes remains elusive, although the cotranslational mode of integration and key lipids are likely to be important factors, as we discuss in length elsewhere (Long, O’Brien, & Alder, Citation2012). In the case of nanodiscs, the observed accelerated desorption of lipids in comparison to lipid vesicles (Nakano et al., Citation2009) may be a mechanistic underpinning for the ability of transmembrane segments to cotranslationally displace existing lipids. Taken together, these studies highlight the promise of using nanodisc-bound MPs generated by CF systems for structural and functional studies.
MP reconstitution into SMALPs/Lipodisqs®
Established methods for the reconstitution of MPs into SMA-bound particles entail the dropwise addition of an SMA solution to the sample of the target protein reconstituted into liposomes followed by centrifugation to remove nonsolubilized protein and subsequent purification steps (e.g. size exclusion chromatography). To date, this approach has been used to reconstitute bR (Knowles et al., Citation2009; Orwick-Rydmark et al., Citation2012), the β-barrel lipid A palmitoyltransferase PagP (Knowles et al., Citation2009), and the KCNQ1 channel regulatory protein KCNE1 (Sahu et al., Citation2013). A further application of this technology is the reconstitution of MPs from native cellular membranes directly into SMA-bound particles. It was recently reported that the SMA copolymer solubilized mitochondrial membranes, enabling the reconstitution of the multisubunit respiratory complex IV into polymer-bound lipoprotein particles in a manner that retained the redox activity of the holoenzyme (Long et al., Citation2013). Hence, this approach presents a means for detergent-free extraction of functional MPs from both synthetic and natural membranes into soluble nanoscale bilayers.
Structural studies
Despite their natural abundance, MPs represent <1% of the unique structures published in the Protein Data Bank, owing largely to the difficulties associated with their crystallization to obtain X-ray structures. Nuclear magnetic resonance (NMR) spectroscopy, however, has been widely used to obtain high-resolution structures of MPs in nanodiscs. Solid-state NMR has been used for structural studies of the nanodisc MSP belt (Li, Kijac, Sligar, & Rienstra, Citation2006) and cytochrome P450 3A4 in nanodiscs (Kijac, Li, Sligar, & Rienstra, Citation2007). More recently, larger 30 nm diameter variants of nanodiscs, termed macrodiscs, have been developed as magnetically alignable media for oriented sample solid-state NMR (Park et al., Citation2011). Solution-state NMR-based studies, in contrast to solid-state NMR, can be limited by the size and slow tumbling rates of large particles. In this regard, nanodiscs are attractive systems for solution NMR due to their size and sufficiently rapid isotropic reorientation. Hence, nanodisc reconstitutions have been used for two-dimensional solution NMR-based studies on the structures and functional dynamics of many MPs, including the voltage-sensing domain of the potassium channel KvAP (Shenkarev et al., Citation2010), the mitochondrial voltage-dependent anion channels VDAC-1 (Raschle et al., Citation2009) and VDAC-2 (Yu, Raschle, Hiller, & Wagner, Citation2012), and the membrane-spanning segment of the CD4 receptor (Glück et al., Citation2009).
In an effort to further improve the quality of NMR spectra of nanodisc-reconstituted samples, recent work has been aimed toward engineering smaller bilayers by systematic reduction of the MSP belt size, resulting in discs with shorter rotational correlation times. The truncation of MSP1D1 by selective removal of internal helices allowed for the reconstitution of bR and the β-barrel OmpX into nanodiscs as small as 6.3 nm in diameter, making them accessible to multidimensional NMR analysis and higher resolution structure determination (Hagn, Etzkorn, Raschle, & Wagner, Citation2013). In a related study, C-terminal truncations of MSP down to seven helical repeats rendered improved 15N TROSY-HSQC spectral quality of integrin aIIB incorporated into nanodiscs, particularly for transmembrane residues (Puthenveetil & Vinogradova, Citation2013). Interestingly, both studies identified lower size limits for nanodiscs: below a certain MSP length threshold, discs lacked stability or began to form heterogeneous samples containing larger particles.
As lipid bilayer mimetics, nanodiscs also offer an excellent system for NMR-based analyses of the lipid-interactive regions of peptides and proteins. This is illustrated by two recent studies on proteins associated with cellular signaling. Using a combination of chemical shift perturbation, transferred cross-saturation, and paramagnetic relaxation enhancement measurements to observe protein-bilayer contacts on the NMR timescale, Ingaki and co-workers assayed the interaction of the FYVE domain of early endosome antigen 1 in the presence of phosphoinositide (PI)-containing nanodiscs (Yokogawa et al., Citation2012). This work defined a structural model for the membrane orientation and mode of interaction between FYVE domain residues and the inositol head groups, providing insight into the nature of bilayer interactions among PI-effector proteins. Using similar NMR techniques, a related study analyzed bilayer-interactive regions of Rheb, a membrane-tethered Ras-type GTPase (Mazhab-Jafari et al., Citation2013). Coupled with molecular dynamics simulations, this work revealed interactions of the GTPase domain with the bilayer, which occurred by two distinct nucleodite-dependent conformers, thus providing clues to the mechanism of Rheb-mediated signal regulation.
Recent advances in other structural approaches to investigate MPs have also employed nanoscale bilayers. Pulsed EPR double electron–electron resonance (DEER) spectroscopy provides distance measurements between paramagnetic centers up to 80 Å apart. BR and KCNE1 reconstituted into SMALPs/Lipodisqs® revealed high-quality DEER-detected distance distributions between selectively incorporated spin labels (Orwick-Rydmark et al., Citation2012; Sahu et al., Citation2013), forecasting the applicability of this technique to other MPs in obtaining distance constrains for modeling, and in determining structural dynamics and ligand associations. Further, advances in the implementation and analysis of small-angle X-ray and neutron scattering (SAXS and SANS) experiments show great promise for future work in obtaining low (~10 Å)-resolution structures of nanodisc-incorporated MPs (Kynde et al., Citation2014; Maric et al., Citation2014).
Protein–protein and protein–ligand interactions
Nanodiscs provide the environment for MPs to assume the proper conformation for interaction with their native cognate-binding partners. One class of MPs widely used in nanodisc-based analyses of protein interactions includes the seven transmembrane-segment G-protein coupled receptors (GPCRs), which respond to external stimuli to activate G proteins and initiate signal cascades. Using nanodisc reconstitutions, the protein associations of rhodopsin, a class A GPCR of vertebrate rod photoreceptor cells, have been elucidated by several studies. Photon absorption triggers the isomerization of the 11-cis chromophore of rhodopsin to the all-trans form, thereby inducing a conformational change to metarhodopsin II (Rh*), which activates the heterotrimeric G-protein transducin. Utilizing the ability to reconstitute rhodopsin into nanodiscs with controlled stoichiometry, studies have shown that monomeric Rh* is able to activate transducin in a stable 1:1 complex (Bayburt, Leitz, Xie, Oprian, & Sligar, Citation2007; D’Antona, Xie, Sligar, & Oprian, Citation2014). Similar studies demonstrated that monomeric rhodopsin is phosphorylated by rhodopsin kinase GRK1 and binds arrestin-1 in a 1:1 M ratio. (Bayburt et al., Citation2011; Vishnivetskiy et al., Citation2013). Hence, by allowing experimental control over MP assembly state, nanodisc-based techniques can give insights into difficult and controversial questions regarding the oligomeric states of functionally active MPs such as GPCRs.
Membrane-level interactive networks involving multicomponent systems can be experimentally intractable in native bilayers. In this regard, nanodiscs provide useful reductionist systems to address protein interactions, as illustrated by recent studies that couple nanodisc reconstitutions with fluorescence spectroscopy techniques. For example, as a model for the clamped vesicle-bilayer junction, the Rothman group used nanodiscs joined by SNARE components in trans and employed Förster resonance energy transfer (FRET)-based analyses to study the mechanism by which calcium-activated synaptotagmin inserts into the bilayer and completes the assembly of the prefusion SNARE complex (Krishnakumar et al., Citation2013). In other studies, the Driessen group used combinations of FRET and fluorescence correlation spectroscopy (FCS) to analyze the protein transport activity and conformational dynamics of the SecYEG translocon (Taufik, Kedrov, Exterkate, & Driessen, Citation2013) and ribosome binding of the YidC insertase (Kedrov et al., Citation2013), demonstrating that single copies of SecYEG or YidC are active as monomers in nanodiscs. Notably, the size of nanodisc-bound MPs can be advantageous when using certain fluorescence approaches to measure protein interactions. FCS, for example, can be used to analyze binding interactions of fluorescent-labeled proteins by an autocorrelation function, which depends on the diffusion coefficients of the bound vs. unbound species. As demonstrated by a recent study (Ly et al., Citation2014), FCS-based binding assays are facilitated using nanodisc-bound samples because the size of the MP-nanodisc complex provides the necessary differential in diffusion times between free and bound fluorescent-binding partners.
Nanodisc technology is also expanding opportunities to identify and isolate the soluble interacting partners of MPs using advances in mass spectrometry (MS)-based quantitative proteomics. In many such assays, MPs in nanodiscs bound by epitope-tagged MSP and anchored to a solid support are useful for co-immunoprecipitation and identification of specific binding partners from complex biological mixtures. Importantly, reconstitution of “bait” MPs into nanodiscs circumvents detergent solubilization, which can destabilize their interactions with potential “prey” proteins. By this approach, the soluble protein interactome of several nanodisc-bound bacterial envelope complexes (the SecYEG channel, the YidC insertase and the maltose transporter MalFGK2) was identified from SILAC-labeled whole cell extracts (Zhang et al., Citation2012). Similar strategies can be used to identify soluble interaction partners of other types of nanodisc-immobilized biomolecules as well. For instance, using nanodiscs containing the intestinal glycolipid ganglioside GM1, the heat-labile enterotoxin B was identified as a specific binding partner from lysates of pathogenic Escherichia coli (Borch, Roepstorff, & Moller-Jensen, Citation2011). Recent progress using native electrospray MS to analyze intact nanodisc complexes in the gas phase highlight the possibility of future studies that focus on subunit identity, stoichiometry, and interactions of nanodisc-bound MPs that are fully assembled (Marty et al., Citation2012; Marty, Zhang, Cui, Gross, & Sligar, Citation2014).
Finally, nanodiscs are excellent platforms for investigating MP-ligand interactions, particularly in cases where ligand binding is perturbed by the presence of detergents or where the catalytic site could be inaccessible when the MP is incorporated into lipid vesicles. For example, one recent study explored the conformational dynamics of nanodisc-bound gamma-glutamyl carboxylase using hydrogen–deuterium exchange mass spectrometry, revealing localized changes in solvent exposure upon binding propeptide substrate (Parker et al., Citation2014). Other studies highlight the use of nanodisc systems in measuring equilibrium ligand binding. A recently developed application combined nanodisc reconstitutions with the scintillation proximity assay, wherein scintillant-loaded beads emit light in the presence of proximal radioligand (within a distance of ~8 μm). Nanodiscs containing the amino acid transporter LeuT were immobilized on scintillation beads followed by titrations of tritiated substrates ([3H]Ala and [3H]Leu) to obtain Kd and Bmax values (Nasr & Singh, Citation2014). Notably, Bmax values and [3H]Ala affinity were both higher in nanodisc-bound samples than detergent-solubilized samples, demonstrating the greater binding activity of the transporter in a lipid bilayer, which is particularly critical for measuring weak interactions. In another recent study, bilayer-sensitive binding was also observed for the bacterial outer membrane β-barrel transporter FhuA, which binds and transports siderophore-complexed iron in a manner dependent on interaction with the inner MP TonB (Mills, Le, Coulton, & Duong, Citation2014). This work revealed a 10-fold decrease in equilibrium affinity of nanodisc-bound FhuA for TonB (Kd = 200 nM) relative to previous measurements of FhuA in detergent micelles (Kd = 20 nM); however, when reconstituted in nanodiscs, the transporter displayed a strong physiologically relevant siderophore-dependent enhancement of TonB binding unlike the case in detergent in which siderophore had little effect. These studies emphasize the importance of performing MP-ligand analyses in a membrane-like environment. They also point to future studies using nanoscale bilayers in which specific features of the bilayer (e.g. lipid composition) can be selectively modulated to determine their effects on ligand interactions.
Lipid-dependent MP activity
Lipids are well known to be important modulators of MP function (Andersen & Koeppe, Citation2007; Cross, Murray, & Watts, Citation2013; Lee, Citation2004). A key advantage of nanodiscs is that they can be synthesized with an experimentally defined lipid content, using either synthetic lipids or those derived from natural sources, to study lipid-dependent protein activity. A compelling illustration of the link between bilayer lipids and enzymatic activity comes from nanodisc reconstitutions of MsbA, an ABC transporter from E. coli that translocates lipid A across the inner membrane in an ATP-dependent manner (Kawai, Caaveiro, Abe, Katagiri, & Tsumoto, Citation2011). This study revealed that the nature of the lipids used for reconstitution (headgroup identities and acyl chain lengths) had a strong effect on ATP binding and hydrolysis rates, suggesting a long-distance functional coupling between the membrane-spanning regions of the transporter and the ATP binding module, which is likely to be distal from the bilayer. Another study addressed the influence of the lipid environment on the photocyle of the light-driven proton pump proteorhodopsin (Ranaghan, Schwall, Alder, & Birge, Citation2011). Photoactive monomers reconstituted into nanodiscs and subjected to time-resolved spectral analysis revealed an acceleration of M and N photocycle intermediates in bilayers of longer, unsaturated lipids (POPC) relative to those containing shorter, saturated lipids (DMPC), possibly due to hydrophobic mismatch.
Nanodiscs have also been extensively used to study the lipid-dependent activity of membrane-bound oxidoreductases. For example, mitochondrial succinate:quinone oxidoreductase reconstituted into nanodiscs of different lipid composition revealed a critical role for the anionic phospholipid cardiolipin in promoting holoenzyme stability and redox activity (Schwall, Greenwood, & Alder, Citation2012). Another complex of the electron transport chain, cytochrome c oxidoreductase, was reconstituted into nanodiscs and subjected to microsecond resolution measurements of proton-coupled electron transfer, revealing an accelerated rate of proton uptake in comparison to detergent-solubilized enzymes (Näsvik Öjemyr, von Ballmoos, Gennis, Sligar, & Brzezinski, Citation2012). Several studies have also examined electron transfer mechanisms of the diflavin cytochrome P450 reductase (CPR) and its native redox partner CYP3A4, a drug-metabolizing member of the cytochrome P450 superfamily. These monotopic MPs of the endoplasmic reticulum mediate the transfer of electrons from NADPH to organic substrates. Using spectroelectrochemical titrations, Sligar and co-workers analyzed the redox potentials of microsomal CYP3A4 and CPR reconstituted into nanodiscs of different lipid composition, finding that membrane insertion shifted the midpoint potentials of the FAD/FMN (CPR) and heme (CYP3A4) to significantly more positive values in a manner that was influenced by the presence of anionic phospholipids (Das, Grinkova, & Sligar, Citation2007; Das & Sligar, Citation2009). These studies demonstrated that the electrostatic field of the bilayer is critical in tuning the redox potentials of these enzymes to make electron transfer thermodynamically feasible. This effect may underpin the recently reported increase in CYP3A4 coupling when reconstituted in the presence of anionic phospholipids (Grinkova, Denisov, McLean, & Sligar, Citation2013). As another experimental approach, neutron reflectometry was employed to monitor redox-dependent structural transitions of nanodisc-bound CPR aligned at the water–silica interface, suggesting that NADPH reduction promoted a compact conformation as a potential mechanism to prevent off-pathway electron transfer (Wadsäter et al., Citation2012). Technical advances in monitoring individual enzyme turnover events of nanodisc-bound CPR (Laursen et al., Citation2014) forecast future single molecule strategies using nanodics to explore discrete functional states of such oxidoreductases and other MPs.
Taken together, these studies demonstrate that nanodiscs are excellent platforms for precisely determining the effects of different lipids on MP structure and function. They also serve to emphasize the point that lipid composition is a critical component in the experimental design of such model membrane-based analyses.
Conclusions
Nanoscale bilayers represent the next frontier in the study of MP structure and function. The solubility, stability, and monodispersity of these model membranes coupled with their facile synthesis make them widely applicable to solution-based studies of MPs that have a broad range of structural complexities. This point is confirmed by the recent studies on MP structure, protein–protein interactions, and protein–lipid interactions that have been covered in this short review. Nanodiscs, having been in development for over a decade, have the proven ability to functionally reconstitute MPs in a controllable oligomeric state and with a user-defined lipid composition. The SMALP/Lipodisq® system as a means of detergent-free MP reconstitution is, by contrast, a relatively nascent technology whose full range of applications has yet to be explored. In this regard, future modifications of the SMA copolymer (size, pendant group stoichiometry and functionalization with molecular tags) will likely be useful in the development of novel experimental applications. In addition to their utility in basic research, these nanoscale bilayers have vast potential for applied technologies, including the development of biocompatible systems for hydrophobic drug delivery and diagnostic applications (Murakami, Citation2012; Ng, Lovell, Vedadi, Hajian, & Zheng, Citation2013; Numata et al., Citation2013), nanoelectronic devices (Goldsmith et al., Citation2011; Ham et al., Citation2010), and multiplexed sensor arrays to detect protein interactions (Sloan, Marty, Sligar, & Bailey, Citation2013). Given the commercial availability of all components required for the synthesis of nanodiscs and SMAPLs/Lipodisqs®, we are sure to see these systems applied to an ever-expanding repertoire of MPs by many independent groups in the coming years.
Note added in proof
A recent publication (Gulati et al., Citation2014), has documented the use of SMA copolymers for the detergent-free extraction of different ABC transporters (the largest being 190 kDa and comprising 17 transmembrane helices) from the membranes of a range of eukaryotic expression systems.
Acknowledgments
We thank the Alder research group for many helpful discussions in preparing this review and acknowledge the relevant studies from many labs that could not be included due to space limitations.
Funding
This work was supported by the National Science Foundation to NA under Grants MCB-1024908 and MCB-1330695.
References
- Andersen, O. S., & Koeppe, R. E., 2nd. (2007). Bilayer thickness and membrane protein function: An energetic perspective. Annual Review of Biophysics and Biomolecular Structure, 36, 107–130.10.1146/annurev.biophys.36.040306.132643
- Bayburt, T. H., Grinkova, Y. V., & Sligar, S. G. (2002). Self-assembly of discoidal phospholipid bilayer nanoparticles with membrane scaffold proteins. Nano Letters, 2, 853–856.10.1021/nl025623 k
- Bayburt, T. H., Leitz, A. J., Xie, G., Oprian, D. D., & Sligar, S. G. (2007). Transducin activation by nanoscale lipid bilayers containing one and two rhodopsins. Journal of Biological Chemistry, 282, 14875–14881.10.1074/jbc.M701433200
- Bayburt, T. H., & Sligar, S. G. (2003). Self-assembly of single integral membrane proteins into soluble nanoscale phospholipid bilayers. Protein Science: A Publication of the Protein Society, 12, 2476–2481.
- Bayburt, T. H., Vishnivetskiy, S. A., McLean, M. A., Morizumi, T., Huang, C. C., Tesmer, J. J., … Gurevich, V. V. (2011). Monomeric rhodopsin is sufficient for normal rhodopsin kinase (GRK1) phosphorylation and arrestin-1 binding. Journal of Biological Chemistry, 286, 1420–1428.10.1074/jbc.M110.151043
- Borch, J., Roepstorff, P., & Moller-Jensen, J. (2011). Nanodisc-based co-immunoprecipitation for mass spectrometric identification of membrane-interacting proteins. Molecular & Cellular Proteomics: MCP, 10, 006775.
- Cappuccio, J. A., Blanchette, C. D., Sulchek, T. A., Arroyo, E. S., Kralj, J. M., Hinz, A. K., … Coleman, M. A. (2008). Cell-free co-expression of functional membrane proteins and apolipoprotein, forming soluble nanolipoprotein particles. Molecular & Cellular Proteomics: MCP, 7, 2246–2253.
- Cross, T. A., Murray, D. T., & Watts, A. (2013). Helical membrane protein conformations and their environment. European Biophysics Journal, 42, 731–755.10.1007/s00249-013-0925-x
- D’Antona, A. M., Xie, G., Sligar, S. G., & Oprian, D. D. (2014). Assembly of an activated rhodopsin–transducin complex in nanoscale lipid bilayers. Biochemistry, 53, 127–134.10.1021/bi4012995
- Das, A., Grinkova, Y. V., & Sligar, S. G. (2007). Redox potential control by drug binding to cytochrome P450 3A4. Journal of the American Chemical Society, 129, 13778–13779.10.1021/ja074864x
- Das, A., & Sligar, S. G. (2009). Modulation of the cytochrome P450 reductase redox potential by the phospholipid bilayer. Biochemistry, 48, 12104–12112.10.1021/bi9011435
- Denisov, I. G., Grinkova, Y. V., Lazarides, A. A., & Sligar, S. G. (2004). Directed self-assembly of monodisperse phospholipid bilayer Nanodiscs with controlled size. Journal of the American Chemical Society, 126, 3477–3487.10.1021/ja0393574
- Denisov, I. G., McLean, M. A., Shaw, A. W., Grinkova, Y. V., & Sligar, S. G. (2005). Thermotropic phase transition in soluble nanoscale lipid bilayers. The Journal of Physical Chemistry B, 109, 15580–15588.10.1021/jp051385 g
- Glück, J. M., Wittlich, M., Feuerstein, S., Hoffmann, S., Willbold, D., & Koenig, B. W. (2009). Integral membrane proteins in nanodiscs can be studied by solution NMR spectroscopy. Journal of the American Chemical Society, 131, 12060–12061.10.1021/ja904897p
- Goldsmith, B. R., Mitala, J. J., Josue, J., Castro, A., Lerner, M. B., Bayburt, T. H., … Johnson, A. T. (2011). Biomimetic chemical sensors using nanoelectronic readout of olfactory receptor proteins. ACS Nano, 5, 5408–5416.10.1021/nn200489j
- Grinkova, Y. V., Denisov, I. G., McLean, M. A., & Sligar, S. G. (2013). Oxidase uncoupling in heme monooxygenases: human cytochrome P450 CYP3A4 in Nanodiscs. Biochemical and Biophysical Research Communications, 430, 1223–1227.10.1016/j.bbrc.2012.12.072
- Gulati, S., Jamshad, M., Knowles, T. J., Morrison, K. A., Downing, R., Cant, N., … Rothnie, A. J. (2014). Detergent free purification of ABC transporters. The Biochemical Journal Retrieved from http://www.ncbi.nlm.nih.gov/pubmed/24758594
- Hagn, F., Etzkorn, M., Raschle, T., & Wagner, G. (2013). Optimized phospholipid bilayer nanodiscs facilitate high-resolution structure determination of membrane proteins. Journal of the American Chemical Society, 135, 1919–1925.10.1021/ja310901f
- Ham, M. H., Choi, J. H., Boghossian, A. A., Jeng, E. S., Graff, R. A., Heller, D. A., … Strano, M. S. (2010). Photoelectrochemical complexes for solar energy conversion that chemically and autonomously regenerate. Nature Chemistry, 2, 929–936.10.1038/nchem.822
- Junge, F., Haberstock, S., Roos, C., Stefer, S., Proverbio, D., Dötsch, V., & Bernhard, F. (2011). Advances in cell-free protein synthesis for the functional and structural analysis of membrane proteins. New Biotechnology, 28, 262–271.10.1016/j.nbt.2010.07.002
- Katzen, F., Fletcher, J. E., Yang, J. P., Kang, D., Peterson, T. C., Cappuccio, J. A., … Kudlicki, W. (2008). Insertion of membrane proteins into discoidal membranes using a cell-free protein expression approach. Journal of Proteome Research, 7, 3535–3542.10.1021/pr800265f
- Kawai, T., Caaveiro, J. M., Abe, R., Katagiri, T., & Tsumoto, K. (2011). Catalytic activity of MsbA reconstituted in nanodisc particles is modulated by remote interactions with the bilayer. FEBS Letters, 585, 3533–3537.10.1016/j.febslet.2011.10.015
- Kedrov, A., Sustarsic, M., de Keyzer, J., Caumanns, J. J., Wu, Z. C., & Driessen, A. J. (2013). Elucidating the native architecture of the YidC: Ribosome complex. Journal of Molecular Biology, 425, 4112–4124.10.1016/j.jmb.2013.07.042
- Kijac, A. Z., Li, Y., Sligar, S. G., & Rienstra, C. M. (2007). Magic-angle spinning solid-state NMR spectroscopy of nanodisc-embedded human CYP3A4. Biochemistry, 46, 13696–13703.10.1021/bi701411g
- Knowles, T. J., Finka, R., Smith, C., Lin, Y. P., Dafforn, T., & Overduin, M. (2009). Membrane proteins solubilized intact in lipid containing nanoparticles bounded by styrene maleic acid copolymer. Journal of the American Chemical Society, 131, 7484–7485.10.1021/ja810046q
- Krishnakumar, S. S., Kümmel, D., Jones, S. J., Radoff, D. T., Reinisch, K. M., & Rothman, J. E. (2013). Conformational dynamics of calcium-triggered activation of fusion by synaptotagmin. Biophysical Journal, 105, 2507–2516.10.1016/j.bpj.2013.10.029
- Kynde, S. A., Skar-Gislinge, N., Pedersen, M. C., Midtgaard, S. R., Simonsen, J. B., Schweins, R., … Arleth, L. (2014). Small-angle scattering gives direct structural information about a membrane protein inside a lipid environment. Acta Crystallographica Section D Biological Crystallography, 70, 371–383.10.1107/S1399004713028344
- Laursen, T., Singha, A., Rantzau, N., Tutkus, M., Borch, J., Hedegård, P., … Hatzakis, N. S. (2014). Single molecule activity measurements of cytochrome p450 oxidoreductase reveal the existence of two discrete functional States. ACS Chemical Biology, 9, 630–634.10.1021/cb400708v
- Lee, A. G. (2004). How lipids affect the activities of integral membrane proteins. Biochimica et Biophysica Acta (BBA) – Biomembranes, 1666, 62–87.10.1016/j.bbamem.2004.05.012
- Li, Y., Kijac, A. Z., Sligar, S. G., & Rienstra, C. M. (2006). Structural analysis of nanoscale self-assembled discoidal lipid bilayers by solid-state NMR spectroscopy. Biophysical Journal, 91, 3819–3828.10.1529/biophysj.106.087072
- Long, A. R., O’Brien, C. C., & Alder, N. N. (2012). The cell-free integration of a polytopic mitochondrial membrane protein into liposomes occurs cotranslationally and in a lipid-dependent manner. PloS One, 7, e46332. doi:10.1371/journal.pone.0046332 [Epub 2012 Sep 25].
- Long, A. R., O’Brien, C. C., Malhotra, K., Schwall, C. T., Albert, A. D., Watts, A., & Alder, N. N. (2013). A detergent-free strategy for the reconstitution of active enzyme complexes from native biological membranes into nanoscale discs. BMC Biotechnology, 13, 41. Retrieved from http://www.biomedcentral.com/1472-6750/13/4110.1186/1472-6750-13-41
- Ly, S., Bourguet, F., Fischer, N. O., Lau, E. Y., Coleman, M. A., & Laurence, T. A. (2014). Quantifying interactions of a membrane protein embedded in a lipid nanodisc using fluorescence correlation spectroscopy. Biophysical Journal, 106, L05–L08.10.1016/j.bpj.2013.12.014
- Lyukmanova, E. N., Shenkarev, Z. O., Khabibullina, N. F., Kopeina, G. S., Shulepko, M. A., Paramonov, A. S., … Kirpichnikov, M. P. (2012). Lipid–protein nanodiscs for cell-free production of integral membrane proteins in a soluble and folded state: Comparison with detergent micelles, bicelles and liposomes. Biochimica et Biophysica Acta (BBA) – Biomembranes, 1818, 349–358.10.1016/j.bbamem.2011.10.020
- Maric, S., Skar-Gislinge, N., Midtgaard, S., Thygesen, M. B., Schiller, J., Frielinghaus, H., … Arleth, L. (2014). Stealth carriers for low-resolution structure determination of membrane proteins in solution. Acta Crystallographica Section D Biological Crystallography, 70, 317–328.10.1107/S1399004713027466
- Marsh, D. (2007). Lateral pressure profile, spontaneous curvature frustration, and the incorporation and conformation of proteins in membranes. Biophysical Journal, 93, 3884–3899.10.1529/biophysj.107.107938
- Marty, M. T., Zhang, H., Cui, W., Blankenship, R. E., Gross, M. L., & Sligar, S. G. (2012). Native mass spectrometry characterization of intact nanodisc lipoprotein complexes. Analytical Chemistry, 84, 8957–8960.
- Marty, M. T., Zhang, H., Cui, W., Gross, M. L., & Sligar, S. G. (2014). Interpretation and deconvolution of nanodisc native mass spectra. Journal of the American Society for Mass Spectrometry, 25, 269–277.10.1007/s13361-013-0782-y
- Mazhab-Jafari, M. T., Marshall, C. B., Stathopulos, P. B., Kobashigawa, Y., Stambolic, V., Kay, L. E., … Ikura, M. (2013). Membrane-dependent modulation of the mTOR activator Rheb: NMR observations of a GTPase tethered to a lipid-bilayer nanodisc. Journal of the American Chemical Society, 135, 3367–3370.10.1021/ja312508w
- Mills, A., Le, H. T., Coulton, J. W., & Duong, F. (2014). FhuA interactions in a detergent-free nanodisc environment. Biochimica et Biophysica Acta (BBA) – Biomembranes, 1838, 364–371.10.1016/j.bbamem.2013.09.022
- Murakami, T. (2012). Phospholipid nanodisc engineering for drug delivery systems. Biotechnology Journal, 7, 762–767.10.1002/biot.v7.6
- Nakano, M., Fukuda, M., Kudo, T., Miyazaki, M., Wada, Y., Matsuzaki, N., … Handa, T. (2009). Static and dynamic properties of phospholipid bilayer nanodiscs. Journal of the American Chemical Society, 131, 8308–8312.10.1021/ja9017013
- Nasr, M. L., & Singh, S. K. (2014). Radioligand binding to nanodisc-reconstituted membrane transporters assessed by the scintillation proximity assay. Biochemistry, 53, 4–6.10.1021/bi401412e
- Näsvik Öjemyr, L., von Ballmoos, C., Gennis, R. B., Sligar, S. G., & Brzezinski, P. (2012). Reconstitution of respiratory oxidases in membrane nanodiscs for investigation of proton-coupled electron transfer. FEBS Letters, 586, 640–645.10.1016/j.febslet.2011.12.023
- Ng, K. K., Lovell, J. F., Vedadi, A., Hajian, T., & Zheng, G. (2013). Self-assembled porphyrin nanodiscs with structure-dependent activation for phototherapy and photodiagnostic applications. ACS Nano, 7, 3484–3490.10.1021/nn400418y
- Numata, M., Grinkova, Y. V., Mitchell, J. R., Chu, H. W., Sligar, S. G., & Voelker, D. R. (2013). Nanodiscs as a therapeutic delivery agent: Inhibition of respiratory syncytial virus infection in the lung. International Journal of Nanomedicine, 8, 1417–1427.
- Nymeyer, H., & Zhou, H. X. (2008). A method to determine dielectric constants in nonhomogeneous systems: application to biological membranes. Biophysical Journal, 94, 1185–1193.10.1529/biophysj.107.117770
- Orwick, M. C., Judge, P. J., Procek, J., Lindholm, L., Graziadei, A., Engel, A., … Watts, A. (2012). Detergent-free formation and physicochemical characterization of nanosized lipid–polymer complexes: Lipodisq. Angewandte Chemie International Edition, 51, 4653–4657.10.1002/anie.201201355
- Orwick-Rydmark, M., Lovett, J. E., Graziadei, A., Lindholm, L., Hicks, M. R., & Watts, A. (2012). Detergent-free incorporation of a seven-transmembrane receptor protein into nanosized bilayer Lipodisq particles for functional and biophysical studies. Nano Letters, 12, 4687–4692.10.1021/nl3020395
- Park, S. H., Berkamp, S., Cook, G. A., Chan, M. K., Viadiu, H., & Opella, S. J. (2011). Nanodiscs versus macrodiscs for NMR of membrane proteins. Biochemistry, 50, 8983–8985.10.1021/bi201289c
- Parker, C. H., Morgan, C. R., Rand, K. D., Engen, J. R., Jorgenson, J. W., & Stafford, D. W. (2014). A conformational investigation of propeptide binding to the integral membrane protein γ-glutamyl carboxylase using nanodisc hydrogen exchange mass spectrometry. Biochemistry, 53, 1511–1520.10.1021/bi401536m
- Proverbio, D., Roos, C., Beyermann, M., Orbán, E., Dötsch, V., & Bernhard, F. (2013). Functional properties of cell-free expressed human endothelin A and endothelin B receptors in artificial membrane environments. Biochimica et Biophysica Acta (BBA) – Biomembranes, 1828, 2182–2192.10.1016/j.bbamem.2013.05.031
- Puthenveetil, R., & Vinogradova, O. (2013). Optimization of the design and preparation of nanoscale phospholipid bilayers for its application to solution NMR. Proteins: Structure, Function, and Bioinformatics, 81, 1222–1231.10.1002/prot.v81.7
- Ranaghan, M. J., Schwall, C. T., Alder, N. N., & Birge, R. R. (2011). Green proteorhodopsin reconstituted into nanoscale phospholipid bilayers (nanodiscs) as photoactive monomers. Journal of the American Chemical Society, 133, 18318–18327.10.1021/ja2070957
- Raschle, T., Hiller, S., Yu, T. Y., Rice, A. J., Walz, T., & Wagner, G. (2009). Structural and functional characterization of the integral membrane protein VDAC-1 in lipid bilayer nanodiscs. Journal of the American Chemical Society, 131, 17777–17779.10.1021/ja907918r
- Roos, C., Zocher, M., Müller, D., Münch, D., Schneider, T., Sahl, H. G., … Bernhard, F. (2012). Characterization of co-translationally formed nanodisc complexes with small multidrug transporters, proteorhodopsin and with the E. coli MraY translocase. Biochimica et Biophysica Acta (BBA) – Biomembranes, 1818, 3098–3106.10.1016/j.bbamem.2012.08.007
- Sahu, I. D., McCarrick, R. M., Troxel, K. R., Zhang, R., Smith, H. J., Dunagan, M. M., … Lorigan, G. A. (2013). DEER EPR measurements for membrane protein structures via bifunctional spin labels and lipodisq nanoparticles. Biochemistry, 52, 6627–6632.10.1021/bi4009984
- Schuler, M. A., Denisov, I. G., & Sligar, S. G. (2013). Nanodiscs as a new tool to examine lipid-protein interactions. Methods in Molecular Biology, 974, 415–433.10.1007/978-1-62703-275-9
- Schwall, C. T., Greenwood, V. L., & Alder, N. N. (2012). The stability and activity of respiratory Complex II is cardiolipin-dependent. Biochimica et Biophysica Acta (BBA) – Bioenergetics, 1817, 1588–1596.10.1016/j.bbabio.2012.04.015
- Seddon, A. M., Curnow, P., & Booth, P. J. (2004). Membrane proteins, lipids and detergents: not just a soap opera. Biochimica et Biophysica Acta (BBA) – Biomembranes, 1666, 105–117.10.1016/j.bbamem.2004.04.011
- Seelig, A., & Seelig, J. (1974). Dynamic structure of fatty acyl chains in a phospholipid bilayer measured by deuterium magnetic resonance. Biochemistry, 13, 4839–4845.10.1021/bi00720a024
- Shenkarev, Z. O., Lyukmanova, E. N., Paramonov, A. S., Shingarova, L. N., Chupin, V. V., Kirpichnikov, M. P., Blommers, M. J., & Arseniev, A. S. (2010). Lipid−protein nanodiscs as reference medium in detergent screening for high-resolution NMR studies of integral membrane proteins. Journal of the American Chemical Society, 132, 5628–5629.10.1021/ja9097498
- Shenkarev, Z. O., Lyukmanova, E. N., Butenko, I. O., Petrovskaya, L. E., Paramonov, A. S., Shulepko, M. A., … Arseniev, A. S. (2013). Lipid–protein nanodiscs promote in vitro folding of transmembrane domains of multi-helical and multimeric membrane proteins. Biochimica et Biophysica Acta (BBA) – Biomembranes, 1828, 776–784.10.1016/j.bbamem.2012.11.005
- Sloan, C. D., Marty, M. T., Sligar, S. G., & Bailey, R. C. (2013). Interfacing lipid bilayer nanodiscs and silicon photonic sensor arrays for multiplexed protein–lipid and protein–membrane protein interaction screening. Analytical Chemistry, 85, 2970–2976.10.1021/ac3037359
- Taufik, I., Kedrov, A., Exterkate, M., & Driessen, A. J. M. (2013). Monitoring the activity of single translocons. Journal of Molecular Biology, 425, 4145.10.1016/j.jmb.2013.08.012
- Vishnivetskiy, S. A., Ostermaier, M. K., Singhal, A., Panneels, V., Homan, K. T., Glukhova, A., … Gurevich, V. V. (2013). Constitutively active rhodopsin mutants causing night blindness are effectively phosphorylated by GRKs but differ in arrestin-1 binding. Cellular Signalling, 25, 2155–2162.10.1016/j.cellsig.2013.07.009
- Wadsäter, M., Laursen, T., Singha, A., Hatzakis, N. S., Stamou, D., Barker, R., … Cardenas, M. (2012). Monitoring shifts in the conformation equilibrium of the membrane protein cytochrome P450 reductase (POR) in nanodiscs. Journal of Biological Chemistry, 287, 34596–34603.10.1074/jbc.M112.400085
- Wallin, E., & von Heijne, G. (1998). Genome-wide analysis of integral membrane proteins from eubacterial, archaean, and eukaryotic organisms. Protein Science: A Publication of the Protein Society, 7, 1029–1038.
- White, S. H., Ladokhin, A. S., Jayasinghe, S., & Hristova, K. (2001). How membranes shape protein structure. Journal of Biological Chemistry, 276, 32395–32398.10.1074/jbc.R100008200
- Yang, J. P., Cirico, T., Katzen, F., Peterson, T. C., & Kudlicki, W. (2011). Cell-free synthesis of a functional G protein-coupled receptor complexed with nanometer scale bilayer discs. BMC Biotechnology, 11, 57.10.1186/1472-6750-11-57
- Yokogawa, M., Kobashigawa, Y., Yoshida, N., Ogura, K., Harada, K., & Inagaki, F. (2012). NMR analyses of the interaction between the FYVE domain of early endosome antigen 1 (EEA1) and phosphoinositide embedded in a lipid bilayer. Journal of Biological Chemistry, 287, 34936–34945.10.1074/jbc.M112.398255
- Yu, T. Y., Raschle, T., Hiller, S., & Wagner, G. (2012). Solution NMR spectroscopic characterization of human VDAC-2 in detergent micelles and lipid bilayer nanodiscs. Biochimica et Biophysica Acta (BBA) – Biomembranes, 1818, 1562–1569.10.1016/j.bbamem.2011.11.012
- Zhang, X. X., Chan, C. S., Bao, H., Fang, Y., Foster, L. J., & Duong, F. (2012). Nanodiscs and SILAC-based mass spectrometry to identify a membrane protein interactome. Journal of Proteome Research, 11, 1454–1459.10.1021/pr200846y
- Zocher, M., Roos, C., Wegmann, S., Bosshart, P. D., Dötsch, V., Bernhard, F., & Müller, D. J. (2012). Single-molecule force spectroscopy from nanodiscs: An assay to quantify folding, stability, and interactions of native membrane proteins. ACS Nano, 6, 961–971.10.1021/nn204624p