Abstract
Ultrasound is an attractive modality for non-invasive imaging to monitor temperature of tumorous regions undergoing hyperthermia therapy. Previously, we predicted monotonic changes in backscattered energy (CBE) of ultrasound with temperature for certain sub-wavelength scatterers. We also measured CBE values similar to our predictions in bovine liver, turkey breast muscle, and pork rib muscle in both 1D and 2D in in vitro studies. To corroborate those results in perfused, living tissue, we measured CBE in both normal tissue and in implanted human tumors (HT29 colon cancer line) in 7 nude mice. Images were formed by a phased-array imager with a 7.5 MHz linear probe during homogeneous heating from 37° to 45°C in 0.5°C steps and from body temperature to 43°C during heterogeneous heating. We used cross-correlation as a similarity measure in RF signals to automatically track feature displacement as a function of temperature. Feature displacement was non-uniform with a maximum value of 1 mm across all specimens during homogeneous heating, and 0.2 mm during heterogeneous heating. Envelopes of image regions, compensated for non-rigid motion, were found with the Hilbert transform then smoothed with a 3 × 3 running average filter before forming the backscattered energy at each pixel. Means of both the positive and negative changes in the BE images were evaluated. CBE was monotonic and accumulated to 4–5 dB during homogeneous heating to 45°C and 3–4 dB during heterogenous heating to 43°C. These results are consistent with our previous in vitro measurements and support the use of CBE for temperature estimation in vivo during hyperthermia.
Introduction
A major limitation of thermal therapies for treatment of cancer is the lack of detailed thermal information available to guide and assess the therapy Citation[1]. In clinical procedures, temperatures are measured invasively and therefore only sparse measurements can be made Citation[2–9]. A non-invasive method for rapid volumetric determination of temperature distribution during treatment would facilitate targeted heating of tumors at therapeutic levels in patients receiving hyperthermia treatment, while minimizing damage to adjacent normal tissue.
To meet the capability of present and forthcoming clinical heating technologies, our long-term goal is to develop a non-invasive, low-cost, clinically useful method to measure three-dimensional (3D) temperature distributions to within 0.5°C in 1 cm3 volumes Citation[1], Citation[10]. Temperature imaging methods based on magnetic resonance (MR) imaging appear to satisfy the spatial resolution goal with 1°C accuracy, but may be limited by temporal resolution of tens of seconds Citation[11–15]. Further, MRI may also ultimately be limited by requirements of significant facilities investment and development of MR-compatible heating therapies.
Ultrasound is a non-ionizing, convenient, and inexpensive modality with relatively simple signal processing requirements, making it an attractive option for temperature imaging if robust methods can be developed. We predicted monotonic changes in backscattered ultrasonic energy (CBE) with temperature from tissue inhomogeneities in the form of subwavelength scatterers Citation[16]. Subsequently, we made 1D and 2D measurements of CBE with temperature in abattoir specimens of bovine liver, turkey breast, and pork muscle that were consistent with the predictions of our single-scatterer model Citation[17–19]. To expand our single-scatterer model, we developed methods for simulating images of multiple discrete, temperature-dependent, sub-wavelength scatterers from which we could extract CBE Citation[20]. Predicted, measured, and simulated CBE were all nearly monotonic with temperature, suggesting that CBE could serve as the basis for ultrasonic thermometry.
An important limitation of the CBE method, however, is that CBE is dependent on changes in the apparent location of scattering regions that occur with changes in speed of sound and tissue motion. Our previous measurements of CBE in 1D required segmenting and tracking echoes from individual scattering regions Citation[19]. In 2D we automatically tracked and compensated for motion Citation[17].
In this study we have extended characterization of CBE temperature dependence to an in vivo system to test CBE for thermometry in perfused, living tissue. This study of CBE in living systems, which includes the influence of blood flow and motion due to respiration, is a necessary step towards using this technique for temperature estimation in the clinic. In addition to measuring CBE in normal living murine tissue, we have also characterized CBE in implanted tumors (HT29 colon cancer line) in nude-mouse preparations.
Ultrasonic measurement of temperature
Methods for using ultrasound as a non-invasive thermometer fall into three categories: (1) those based on the measurement of the acoustic attenuation coefficient, (2) those based on echo shifts due to thermal expansion and changes in speed of sound (SOS), and (3) our approach, which exploits changes in backscattered energy (CBE) from a single backscatter view measured with standard equipment. We have recently reviewed the first two methods Citation[21] and will briefly describe the use of CBE here.
Backscattered energy from sub-wavelength scatterers
The choice of CBE as a monotonic temperature-dependent parameter to exploit for temperature imaging was based on the expression developed by Sigelmann and Reid for the average backscattered energy from a small scattering volume containing a random distribution of scatterers in response to insonification by a sinusoidal burst Citation[22]. To eliminate parameters that are not temperature dependent, we normalized that expression to the energy received at reference temperature TR to predict CBE at temperature T Citation[16].As functions of temperature, α(T) is the attenuation within the tissue volume and η(T) is the backscatter coefficient of the tissue volume. Distance x is the path length in the tissue volume. We inferred the temperature dependence of the backscatter coefficient from the scattering cross section of a sub-wavelength scatterer Citation[23], Citation[24]. Neglecting the small change in the wave number (<1.5%) with temperature, we assumed Citation[16],
where ρ and c are the density and speed of sound of the scatterer s and medium m. This expression applies to conditions where the wavelength λ is larger than 2πa, where a is the radius of the scatterer. The effect of thermal expansion was not included because for the hyperthermia range it is expected to cause <2 μm of scatterer motion, which will have no measurable effect on backscattered energy Citation[19], Citation[25].
Using reported attenuation and SOS data, along with inferred backscatter coefficients, we predicted nearly monotonic CBE with temperature at 7.5 MHz from aqueous and lipid scatterers of as much as 5 dB over the temperature range from 37° to 50°C. Changes were both positive and negative with extents of 5 and −3 dB, respectively. shows these predictions over the temperature range used in this study.
Figure 1. Left: CBE predicted over the temperature range employed in this study using Equation 1 for single lipid and aqueous scatterers with thermal properties given in our initial theoretical study Citation[16]. Right: CBE from simulated B-mode images with collections of scatterers in the presence of noise Citation[20].
![Figure 1. Left: CBE predicted over the temperature range employed in this study using Equation 1 for single lipid and aqueous scatterers with thermal properties given in our initial theoretical study Citation[16]. Right: CBE from simulated B-mode images with collections of scatterers in the presence of noise Citation[20].](/cms/asset/c1ccd168-72ee-4640-a1c9-701eadf8006f/ihyt_a_294385_f0001_b.gif)
Backscattered energy from collections of scatterers
To expand our theoretical CBE prediction from a single scatterer to an entire image representing multiple populations of multiple scatterer types, we developed methods for modeling and simulating images of multiple discrete, temperature-dependent, sub-wavelength scatterers Citation[20]. Our physical model for image formation combined a linear model for the radio-frequency (RF) image with a discrete-scatterer model for the tissue medium Citation[26]. The imaging system was characterized by its 3D point-spread function. Image formation was represented as the convolution of the reflectivity of the scatterer distribution and a spatially invariant point-spread function.
Using the same method applied to our experimental images, CBE images were computed from simulated, envelope-detected B-mode images by squaring and averaging with a 3 × 3 moving average filter Citation[17]. shows predictions in the presence of noise for positive and negative CBE from simulated 1 × 1 cm2 B-mode images with 2000 aqueous and lipid scatterers in a 2:1 ratio with the same properties as our single-scatterer model. CBE plots from simulated images with noise closely match our CBE results measured in vitro Citation[17].
Experimental methods
To test the use of CBE as a parameter for temperature estimation in vivo, phased-array images of live nude-mouse specimens were acquired as a function of temperature under two heating conditions: (1) homogeneous heating in a water bath, (2) heterogeneous heating with our small animal hyperthermia ultrasound system (SAHUS) Citation[27], Citation[28].
Imaging during homogeneous heating was done with a Terason 2000 (Teratech Corp., Burlington, MA) laptop-based imaging system using a 7.5-MHz, 128-element array (model 10L5). After upgrading our Terason system, we imaged during heterogeneous heating with a Terason 3000 system using a similar transducer (model 12L5). These 2D images were analysed in the same manner used for our in vitro studies Citation[17].
Measurements during homogeneous heating
Measurements of backscattered energy as a function of temperature with homogeneous heating were made on four live nude mice. One had implanted tumors (HT29 colon cancer line) on its hind quarters. All were anesthetized with ketamine xylazine prior to being secured to a platform as shown in . The platform and the lower section of the mouse were submerged into a degassed, deionized water bath and homogeneously heated in the same method used in our previous in vitro experiments Citation[17]. The water was degassed to reduce release of air bubbles during heating which are strong ultrasound scatterers. The water was deionized to give it properties similar to those of distilled water, for which SOS as a function of temperature is well known.
Figure 2. Configuration for automatic heating and ultrasonic imaging of nude mice. Deionized and degassed water was heated to equilibrium under control of the laptop-based imaging system.
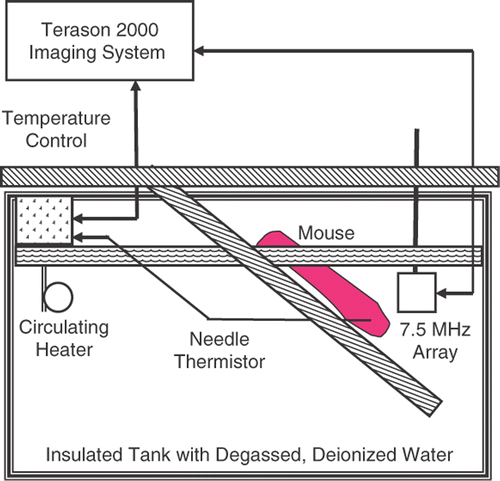
The notebook computer that the Terason 2000 was based upon was also used to regulate tissue heating. Tissue temperature was set by controlling a circulating heater (Thermo Haake, Newington, NH) via a serial link using a custom Matlab (Mathworks, Inc., Natick, MA) routine. Temperature was measured with a needle RTD thermistor (Type HYP-4, Omega Technologies, Stamford, CT) in the limb contralateral to the one imaged.
Images, focused at the center of the mouse leg (at about 2 cm), were taken in 0.5°C increments from 37°C to 45°C over about 30 minutes. We reduced the duration of the experiment and hence the temperature range studied, compared to in vitro experiments, to be sure the mice remained fully anesthetized during the experiment. Temperature from the needle thermistor was reported to the Matlab routine, which turned off the heater and circulator when the desired temperature was reached. Control was then switched to the Terason 2000 to acquire and save an image frame. This process was automated. All keystrokes needed to switch between Matlab and the Terason 2000 program, and to save image files were administered using AutoIT keystroke-emulation software (hiddensoft.com).
Measurements during heterogeneous heating
We measured CBE in images made during heterogeneous heating with our small animal treatment system (SAHUS) Citation[27] in three nude mice with implanted human tumors (HT29 colon cancer line). shows the SAHUS with a 5 MHz high-power acoustic heating element, along with the 7.5 MHz array of our Terason 3000 system. The 128-element, linear array was positioned above the tumor and coupled to the heated leg with acoustic gel. A thermocouple sensor (Type HYP-2, Omega Technologies, Stamford, CT) was placed near the center of the tumor to measure temperature rise from body temperature to 43°C, which took about 6 minutes. At 43°C the heating system was turned off and an image acquired.
Tracking and compensating for apparent and actual motion
As tissue is heated, features in fixed-frame images appear to move because the speed of sound changes, but SOS used by the imaging system is fixed. In addition, of course, there is actual motion due to perfusion, respiration and other sources in the anesthetized preparation.
Estimation of tissue motion is a key step in many applications associated with ultrasound imaging, such as elasticity imaging Citation[29–32], estimation of the velocity of blood flow Citation[33–35], and non-invasive temperature estimation, including our work Citation[18], Citation[36], Citation[37]. These approaches have focused on 2D motion in ultrasound images.
We developed optimization-based, non-rigid motion estimation algorithms in both 2D and 3D. They were implemented in Matlab. The cost function was the normalized cross-correlation of two RF datasets (RF signals available courtesy of Teratech Corp. from both the Terason 2000 and 3000).
Motion compensation in 2D was used for the studies reported here. The motion field in 2D (over a tissue region of interest) was represented as a linear function of 2D motion vectors at 4 reference points (the corners) of each image. The optimization algorithm then used standard gradient-based techniques to find the motion field that locally minimized the cost function evaluated over the entire volume. For a sequence of frames, the motion between consecutive frames was found and accumulated to find the relative motion of all frames to the reference frame.
Backscattered energy in motion-compensated images
Once apparent tissue motion was accounted for, CBE was calculated over the measured temperature range. Envelopes of motion-compensated image regions were found with the Hilbert transform then smoothed with a 3 × 3 running average filter Citation[17]. Values were squared to determine the backscattered energy at each pixel. The backscattered energy image at 37°C was the reference for BE images during homogeneous heating; the body temperature image was the reference during heterogeneous heating.
For some image regions CBE was positive as temperature increased, for others CBE was negative. This behavior was predicted by our model of the CBE for a single scatterer, depending on its density and speed of sound Citation[16]. The means of CBE from pixels with increasing backscattered energy were found, as were the means from pixels with decreasing backscattered energy.
Non-thermal and unwanted thermal effects
We tested our system previously under conditions for which we expected to see no change in backscattered energy. We measured CBE in bovine liver over the time required for an experiment, but without heating, to determine the magnitude of non-thermal effects. CBE values of < ± 0.2 dB were typical of the changes seen over 80 minutes, twice the approximate duration of an experiment. The AIUM 100-mm test object Citation[38] was used to evaluate thermal effects on the Terason 2000 transducer itself Citation[17]. CBE from a wire in the test object over the time of a typical experiment was < ± 0.1 dB at a constant temperature. Apparent motion of a stainless steel wire in the AIUM test object heated from 37°C to 50°C was accounted for by the expected change in SOS in water. In addition the spectra of the radio-frequency echoes did not change significantly over the 37° to 50°C range. These small non-thermal effects have been neglected in the results presented here.
Results
To investigate the effect of temperature on backscattered energy in vivo, we imaged nude mice undergoing both homogeneous and heterogeneous heating in the hyperthermia range. Images were formed by a Terason imager with a 7.5-MHz linear transducer array. shows an image of a nude mouse undergoing homogeneous heating.
Motion of scattering regions
Motion was estimated using optimization techniques described in the Methods. Examples of motion fields during homogeneous heating over 0.5°C steps at 3°C intervals are shown in for the image region included in the figure. The image region contained all of the tissue in the leg and part of the abdomen. Based on these results motion is clearly non-uniform from one temperature to the next. As can also be seen in the figure, the magnitude and direction of the motion at a given site in the tissue also differs depending on which 0.5°C step is considered.
Figure 5. Image region at 37°C and 2D motion fields over 0.5°C steps at 3°C intervals during homogeneous heating estimated using optimization techniques. Image intensity is in dB. Arrow lengths are 50 times the actual motion. The magnitude of the largest motion was about 100 μm. Motion values were found via bilinear interpolation over the image.
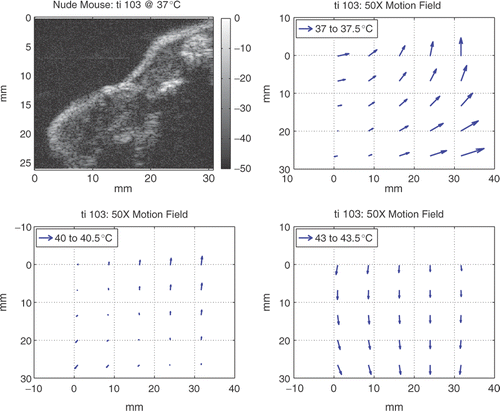
During homogeneous heating, axial motion across all specimens ranged from −0.08 to 0.7 mm with a mean for total axial displacement across all experiments of 0.4 mm. The extent of axial motion in vivo was about 0.2 mm greater than that seen previously during in vitro homogeneous heating Citation[17]. The range of lateral motion across all specimens was from −1.1 to 1.1 mm with a mean total lateral displacement of 0.04 mm. This lateral motion in vivo was about twice that seen in vitro Citation[17]. During heterogeneous heating, axial motion from body temperature to 43°C was <0.16 mm; lateral motion was <0.3 mm.
These findings mean that on average tissue movement was <60 μm per 0.5°C step during homogeneous heating and <160 μm during heterogeneous heating. These small changes are consistent with visual observation of motion and are in a range over which the motion tracking and compensation methods functioned well.
Change in backscattered energy in in vivo images
The energy at each pixel in motion-compensated images was calculated as indicated in the Methods and compared to its value at the reference temperature, which was 37°C for homogeneous heating in a water bath or body temperature for heterogeneous heating with SAHUS Citation[27]. For some image regions CBE was positive, for others it was negative as temperature increased. This kind of change was predicted by our model of the CBE for a single scatterer, depending on its density and speed of sound Citation[16].
CBE during homogeneous heating
CBE from the thigh of each of the four nude-mouse preparations is shown in . The means of CBE from pixels with increasing backscattered energy are plotted along with the means from pixels with decreasing backscattered energy. Both sets of CBE curves (increasing CBE and decreasing CBE) are nearly monotonic with temperature.
Figure 6. CBE measured over the thighs of live nude mice. Means of CBE at pixels with increasing BE and means at pixels with decreasing BE are shown from four experiments.
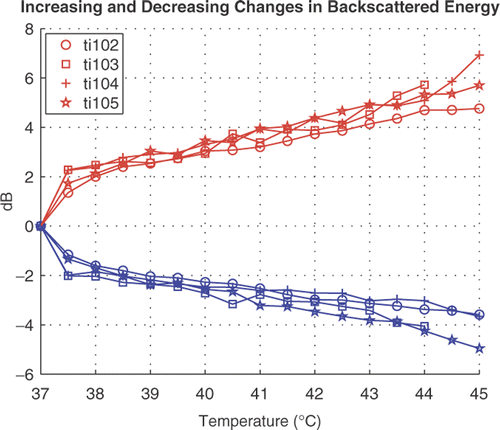
After an initial jump in CBE from 37 to 37.5°C of about 2 dB, the positive CBE undergoes another 3–4 dB increment by 45°C. The initial jump in the negative CBE curves is also nearly 2 dB. The magnitude of the negative CBE from 37.5° to 45°C is 2–3 dB, about 1 dB less than seen in the positive CBE measurements.
The experiment labeled ti102 in was performed on a nude mouse with an implanted HT29 tumor. The CBE results from that experiment came from the tumor region and are similar to the CBE results from normal tissue in the other three nude mice.
CBE during heterogeneous heating
shows an image of an HT29 tumor in the thigh of a nude mouse after heterogeneous heating using our SAHUS appliance pictured in . The circle in the image shows the position and size of the heating transducer. The thermocouple, coming in from the left with its tip near the center of the tumor, measured 43°C. The inset in the image above the thermocouple indicates the region from which the CBE image was taken. CBE at 43°C was relative to body temperature for the mouse. Dark regions in the CBE image indicate a reduction in BE compared to body temperature; light regions indicate an increase in BE.
Figure 7. Upper panel: Terason 3000 image at 7.5 MHz of an HT29 tumor in the leg of a nude mouse heated to 43°C at the thermocouple. The circle shows the position and size of the heating transducer. Lower panel: CBE at 43°C compared to body temperature for the inset region above the thermocouple probe in the conventional image. Tissue volume for the CBE image is 0.075 cm3. Vertical bars show the image intensities in dB.
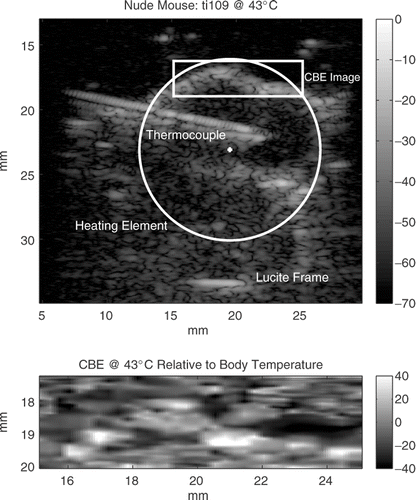
Tissue volume of the CBE images was about 0.075 cm3 (1.0 × 0.25 × 0.3 cm3, elevation beam width is about 3 mm). Positive CBE from body temperature to 43°C in the tumors in the thighs of three nude mice is shown in . Positive CBE values were the means of the pixel values with increasing BE in the inset images, as shown in . CBE at 43°C relative to body temperature was between 3.1 and 3.6 dB with a mean of 3.5 dB in three nude mice over a 0.075 cm3 tissue volume in each mouse. The mean of the corresponding negative CBE values was −3.4 dB, but the individual values varied more. They were −2.0, −4.5, and −3.7 dB. Both our previous in vitro results and our simulations of scatterer collections show that, as expected, the standard deviation of the CBE value at a given temperature is reduced as the size of the image region is increased.
Figure 8. Mean positive CBE at 43°C relative to body temperature in a portion of implanted HT29 tumors in three nude mice after heterogeneous heating in our SAHUS apparatus shown in Citation[27].
![Figure 8. Mean positive CBE at 43°C relative to body temperature in a portion of implanted HT29 tumors in three nude mice after heterogeneous heating in our SAHUS apparatus shown in Figure 3 Citation[27].](/cms/asset/e455ca71-1f82-45d9-8937-9de61966ea1b/ihyt_a_294385_f0008_b.gif)
The in vivo homogeneous heating CBE curves and the extent of CBE during in vivo heterogeneous heating are similar to our in vitro results. Furthermore, our simulations of collections of scatterers account for these observations, including the dependence of CBE on signal-to-noise ratio Citation[20]. These results suggest that CBE, which is monotonic with temperature, can serve as the basis for non-invasive thermal imaging in living systems.
Discussion
Ultrasonic images during previous in vitro homogeneous heating studies showed apparent motion in both the lateral and axial directions of up to 0.5 mm Citation[17]. This motion was estimated over small image regions, assuming rigid motion, by maximizing the cross correlation in RF images at adjacent temperatures to track feature displacement. After compensation for apparent axial and lateral movement of image features during heating, image regions showed CBE with temperature that was clearly distinct from changes due to movement.
Here we used a non-rigid optimization technique that allowed us to estimate motion over larger image regions of interest than previously as a single operation. The ability to apply a non-rigid deformation to images of large tissue volumes is an important step toward clinical application of ultrasonic temperature imaging based on CBE. This is an important development because we can now find CBE over tissue volumes that match our 1 cm3 spatial resolution goal for thermal imaging in a single operation.
As expected, motion seen in vivo was greater than that found in vitro due to the presence of actual as well as apparent motion. Less axial motion was seen in the in vivo experiments, however, because effects of SOS changes in the water path were reduced. The water path was shorter in the homogeneous heating studies; there was no water path in the heterogeneous heating experiments.
Motion compensation was done in 2D. We have conducted preliminary in vitro experiments in 3D by taking seven 2D images separated by 0.6 mm in elevation at each temperature. The motion encountered in elevation was much smaller than the beam width in elevation, so that the percentage of scatterers that move in or out of the beam in elevation is smaller than that in the lateral direction. We plan to corroborate the results presented here by evaluating CBE in 3D using our 3D non-rigid motion compensation algorithms. Nevertheless, based on the present results and the expectation of a small effect on CBE of motion in elevation, we expect CBE to support temperature estimation in 3D.
After motion compensation, positive and negative CBE excursions tracked nearly monotonically with temperature in the 37° to 45°C range during homogeneous heating. The CBE plots in closely match our previous in vitro results Citation[17], which means that perfusion and other effects of a living system did not alter the CBE dependence on temperature seen in vitro.
In vivo measurements presented here were also similar to our previous single-scatterer predictions and CBE calculated from simulated images, as seen in . That figure shows the effect of noise on CBE. We showed in simulations of collections of scatterers that the size of the jump in CBE from the 37°C to 37.5°C is inversely related to the signal-to-noise ratio Citation[20]. Noise also accounts for the minor variations in CBE as temperature increases after the initial jump, as well. Both of these effects are illustrated in .
Future work includes estimation of CBE in vitro in 3D and the use of CBE calculated from simulated images to establish the effects of both rigid and non-rigid motion on CBE by statistically analysing the dependence of CBE on temperature in 3D in the presence of noise. Even so, the results from this study already support the use of backscattered ultrasonic energy as a modality for non-invasive thermometry and are consistent with theoretical expectations and previous experimental findings.
Conclusions
The change in backscattered energy in motion-compensated images from a phased-array system was measured in vivo in nude mice during both homogeneous and heterogeneous heating. Cross-correlation was used as a similarity measure for automatic non-rigid tracking of feature displacement as a function of temperature. Maximum feature displacement across the specimen was about 1 mm during homogenous heating in 15 0.5°C steps. The typical displacement (<60 μm) from temperature to temperature was easily handled using the cross-correlation approach. CBE in motion-compensated images was nearly monotonic with a maximum change of 4–5 dB. Displacements during heterogeneous heating were <160 µm with about a 3.5 dB change from body temperature to 43°C. Results from this study are consistent with our theoretical predictions, simulations from collections of scatterers, and our previous findings in vitro in 2D.
Knowledge gained from these studies is an important part of the translation of CBE thermometry to a clinical setting that will enhance our ability to deal with similar circumstances in human patients. In vivo success of CBE temperature estimation based on 3D non-rigid tracking and compensation for real and apparent motion of image features could serve as the foundation for the eventual generation of 3D temperature maps in soft tissue in a non-invasive, convenient, and low-cost way in clinical hyperthermia and other biomedical applications.
Acknowledgements
This work was supported in part by NIH grants R21-CA90531 and R01-CA107558 from the National Cancer Institute and by the Wilkinson Trust at Washington University in St Louis. We are grateful for the cooperation of Dr Buck Rogers in providing the murine specimens and to Mr Jesse Parry for his assistance in conducting the nude-mouse experiments. We also wish to thank Mr Yuzheng Guo for his help with the motion analyses.
Declaration of interest: The authors report no conflicts of interest. The authors alone are responsible for the content and writing of the paper.
References
- Moros E, Corry P, Orton C. Point/counterpoint: Thermoradiotherapy is underutilized for the treatment of cancer. Med Phys 2007; 34(1)1–4
- Dewhirst M, Prosnitz L, Thrall D, Prescott D, Cleff S, Charles C, Macfall J, Rosner G, Samulski T, Gillette E, et al. Hyperthermic treatment of malignant diseases: Current status and a view toward the future. Semin Oncol 1997; 24: 616–625
- Myerson R, Moros E, Roti-Roti J. Principles and Practice of Radiation Oncology 3rd ed, C Perez, L Brady. Lippincott-Raven, Philadelphia 1998; 637–683, ch 24 Hyperthermia In
- Myerson R, Straube W, Moros E, Emami B, Lee H, Perez C, Taylor M. Simultaneous superficial hyperthermia and external radiotherapy: Report of thermal dosimetry and tolerance to treatment. Int J Hyperthermia 1999; 15: 251–266
- Dewhirst M, Sim D, Sapareto S, Coner W. Importance of minimum tumor temperature in determining early and long-term responses of spontaneous canine and feline tumors to heat and radiation. Cancer Res 1984; 44: 43–50
- Myerson R, Perez C, Emami B, Straube W, Kuske R, Leybovich L, Gerichten JV. Tumor control in long-term survivors following superficial hyperthermia. Int J Radiat Oncol Biol Phys 1990; 18: 1123–1129
- Leopold K, Dewhirst M, Samulski T, Dodge R, George S, Blivin J, Progsnitz L, Oleson J. Relationships among tumor temperature, treatment time, and histopathological outcome using preoperative hyperthermia with radiation in soft tissue sarcomas. Int J Radiat Oncol Biol Phys 1992; 22: 989–998
- Hand J, Machin D, Vernon C, Whaley J. Analysis of thermal parameters obtained during phase III trials of hyperthermia as an adjunct to radiotherapy in the treatment of breast carcinoma. Int J of Hyperthermia 1997; 13: 343–364
- Dewhirst M, Sneed P. Those in gene therapy should pay closer attention to lessons from hyperthermia. Int J Radiat Oncol Biol Phys 2003; 57: 597–599, author reply:599–600
- Sun Z, Ying H. A multi-gate time-of-flight technique for estimation of temperature distribution in heated tissue: theory and computer simulation. Ultrasonics 1999; 37: 107–122
- Carter D, MacFall J, Wan SCSX, Prescott D, Charles H, Samulski T. Magnetic resonance thermometry during hyperthermia for human high-grade sarcoma. Int J Radiat Oncol Biol Phys 1998; 40: 815–822
- Nadobny J, Wlodarczyk W, Westhoff L, Gellermann J, Felix R, Wust P. A clinical watercoated antenna applicator for MR-controlled deep-body hyperthermia: A comparison of calculated and measured 3-D temperature data sets. IEEE Trans Biomed Eng 2005; 52(3)505–519
- de Senneville B, Quesson B, Moonen C. Magnetic resonance temperature imaging. Int J Hyperthermia 2005; 21: 515–531
- Gellermann J, Wlodarczyk W, Feussner A, Fahling H, Nadobny J, Hildebrandt B, Felix R, Wust P. Methods and potentials of magnetic resonance imaging for monitoring radiofrequency hyperthermia in a hybrid system. Int J Hyperthermia 2005; 21: 497–513
- Gellermann J, Wlodarczyk W, Ganter H, Nadobny J, Fahling H, Seebass M, Felix R, Wust P. A practical approach to thermography in a hyperthermia/magnetic resonance hybrid system: Validation in a heterogeneous phantom. Int J Radiat Oncol Biol Phys 2005; 61(1)267–277
- Straube W, Arthur R. Theoretical estimation of the temperature dependence of backscattered ultrasonic power for noninvasive thermometry. Ultrasound Med Biol 1994; 20: 915–922
- Arthur R, Trobaugh J, Straube W, Moros E. Temperature dependence of ultrasonic backscattered energy in motion-compensated images. IEEE Trans UFFC 2005; 52: 1644–1652
- Arthur R, Trobaugh J, Straube W, Moros E, Sangkatumvong S. Temperature dependence of ultrasonic backscattered energy in images compensated for tissue motion. Proceedings of the 2003 International IEEE Ultrasonics Symposium No. 03CH37476C. IEEE Press, New York 2003; 990–993
- Arthur R, Straube W, Starman J, Moros E. Noninvasive temperature estimation based on the energy of backscattered ultrasound. Med Phys 2003; 30: 1021–1029
- Trobaugh J, Arthur R, Straube W, Moros E. A simulation model for ultrasonic temperature imaging using change in backscattered energy. J Ultrasound Med Biol 2008; 34: 289–298
- Arthur R, Trobaugh J, Straube W, Moros E. Noninvasive estimation of hyperthermia temperatures with ultrasound. Int J Hyperthermia 2005; 21: 589–600
- Sigelmann R, Reid J. Analysis and measurements of ultrasonic backscattering from an ensemble of scatterers excited by sine wave bursts. J Acoust Soc Am 1973; 53: 1351–1355
- Morse P, Ingard K. Theoretical Acoustics. McGraw-Hill, New York 1968; 427
- Shung K, Smith M, Tsui B. Principles of Medical Imaging. Academic Press, San Diego, CA 1992; 90–99
- Seip R, Ebbini E. Noninvasive estimation of tissue temperature response to heating fields using diagnostic ultrasound. IEEE Trans Biomed Eng 1995; 42: 828–839
- Trobaugh J, Arthur R. A discrete-scatterer model for ultrasonic images of rough surfaces. IEEE Trans UFFC 2000; 47(6)1520–1529
- Singh A, Moros E, Novak P, Straube W, Zeug A, Locke J, Myerson R. Micropet-compatible, small animal hyperthermia ultrasound system (SAHUS) for sustainable, collimated and controlled hyperthermia of subcutaneously implanted tumours. Int J Hyperthermia 2004; 20(1)32–44
- Locke J, Zeug A, Thompson D, Allan J, Mazzarella K, Novak P, Hanson D, Singh A, Moros E, Pandita T. Localized versus regional hyperthermia: Comparison of xenotransplants treated with a small animal ultrasound system and waterbath limb immersion. Int J Hyperthermia 2005; 21: 271–281
- O’Donnell M, Skovoroda A, Shapo B, Emelianov S. Internal displacement and strain imaging using ultrasonic speckle tracking. IEEE Trans UFFC 1994; 41(3)314–325
- Lubinski M, Emelianov S, O’Donnell M. Speckle tracking methods for ultrasonic elasticity imaging using short-time correlation. IEEE Trans UFFC 1999; 46: 82–96
- Xunchang C, Zohdy M, Emelianov S, O’Donnell M. Lateral speckle tracking using synthetic lateral phase. IEEE Trans UFFC 2004; 51(5)540–550
- Vogt M, Ermert H. Development and evaluation of a high-frequency ultrasound-based system for in vivo strain imaging of the skin. IEEE Trans UFFC 2005; 52(3)375–385
- Forster S, Embree P, O’Brien W, Jr. Flow velocity profile via time-domain correlation: Error analysis and computer simulation. IEEE Trans UFFC 1990; 37(2)164–175
- Bohs L, Trahey G. A novel method for angle independent ultrasonic imaging of blood flow and tissue motion. IEEE Trans Biomed Eng 1991; 38(3)280–286
- Lai X, Torp H. Interpolation methods for time-delay estimation using cross-correlation method for blood velocity measurement. IEEE Trans UFFC 1999; 46(2)277–290
- Hein I, O’Brien W, Jr. Current time-domain methods for assessing tissue motion by analysis from reflected ultrasound echoes. IEEE Trans UFFC 1993; 40(2)84–102
- Ebbini E. Phase-coupled two-dimensional speckle tracking algorithm. IEEE Trans UFFC 2006; 53(5)972–990
- Erikson K, Carson P, Stewart H. Field evaluation of the AIUM standard 100 mm test object. Ultrasound Med 1976; 2: 445–451