Abstract
Purpose: Mild temperature hyperthermia (MTH) increases blood flow and oxygenation in tumours. On the other hand, high-dose-per-fraction irradiation damages blood vessels, decreases blood flow and increases hypoxia in tumours. The radiation-induced hypoxia in tumours activates hypoxia-inducible factor-1α (HIF-1α) and its target genes, such as vascular endothelial growth factor (VEGF), promoting revascularization and recurrence. In the present study, we examined the hypothesis that MTH inhibits radiation-induced upregulation of HIF-1α and its target genes by increasing tumour oxygenation.
Materials and methods: FSaII fibrosarcoma tumours grown subcutaneously in the legs of C3H mice were used. Tumours were irradiated with 15 Gy using a 60Co irradiator or heated at 41 °C for 30 min using an Oncothermia heating unit. Blood perfusion and hypoxia in tumours were assessed with Hoechst 33342 and pimonidazole staining, respectively. Expression levels of HIF-1α and VEGF were determined using immunohistochemical techniques. Apoptosis of tumour cells was quantitated via TUNEL staining and the effects of treatments on tumour growth rate were assessed by measuring tumour diameters.
Results: Irradiation of FSaII tumours with a single dose of 15 Gy led to significantly decreased blood perfusion, increased hypoxia and upregulation of HIF-1α and VEGF. On the other hand, MTH at 41 °C for 30 min increased blood perfusion and tumour oxygenation, thereby suppressing radiation-induced HIF-1α and VEGF in tumours, leading to enhanced apoptosis of tumour cells and tumour growth delay.
Conclusion: MTH enhances the anti-tumour effect of high-dose irradiation, at least partly by inhibiting radiation-induced upregulation of HIF-1α.
Introduction
Hyperthermia is a temperature-dependent clinical regimen used to kill tumour cells and enhances the sensitivity of tumours to radiotherapy and specific chemotherapeutic drugs [Citation1–10]. However, the specific mechanisms underlying heat-induced radiosensitization and chemosensitisation, particularly in vivo, are unclear at present. Application of heat at temperatures higher than 42–43 °C kills tumour cells both directly and indirectly by destroying tumour vasculature [Citation11,Citation12]. On the other hand, mild temperature hyperthermia (MTH) at 39–42 °C causes little direct killing of tumour cells but increases the cytotoxic effects of radiation largely by inhibiting repair of radiation-induced damage. Additionally, MTH increases the response of tumours to ionising radiation by promoting tumour blood perfusion, and thereby, tumour oxygenation [Citation13–18]. Earlier studies indicate that MTH enhances the efficacy of specific anti-cancer drugs by augmenting delivery to tumours via increasing blood perfusion and cellular uptake [Citation7,Citation17]. Furthermore, regional MTH of tumours or whole-body heating at fever-range temperatures has been shown to elevate anti-tumour or systemic immunity [Citation19–23].
Owing to the remarkable technical improvements in tumour imaging and radiation delivery systems in recent years, increasing numbers of cancer patients have been treated with stereotactic body radiation therapy (SBRT) or stereotactic radiation surgery (SRS) that precisely delivers 15–50 Gy of radiation to tumours in 1–5 fractions [Citation24–27]. This type of high-dose hypofractionated irradiation has been shown to induce vascular damage in tumours [Citation28–30], in turn, triggering secondary or indirect tumour cell death [Citation28,Citation31–34]. Importantly, recent studies have reported that high-dose irradiation can also induce activation of various cell survival signals such as HIF-1α and its target genes in tumour cells [Citation35–40].
HIF-1α is the master transcription factor upregulated under conditions of hypoxia that plays a crucial role in the survival and proliferation of tumour and stromal cells through transcription of numerous genes [Citation35–44]. HIF-1α promotes angiogenesis and vasculogenesis by upregulating vascular endothelial growth factor (VEGF) [Citation35,Citation40] and C-X-C motif chemokine 12 (CXCL12) [Citation44], and its activation is believed to play a pivotal role in relapse and metastasis of tumours after various treatments, including radiotherapy.
Activation of HIF-1α is regulated by an O2-independent mechanism, but HIF-1α is degraded rapidly in the presence of oxygen. Under aerobic conditions, HIF-1α is hydroxylated, leading to binding with Von Hippel–Lindau (VHL) protein, which promotes its ubiquitination and proteasome-mediated degradation [Citation45,Citation46].
The purpose of the present study was to elucidate the potential influence of the MTH-induced increase in tumour oxygenation on expression of HIF-1α and VEGF in irradiated tumours. Exposure to 15 Gy irradiation caused profound accumulation of HIF-1α and VEGF in FSaII mouse tumours, which was effectively suppressed by MTH at 41 °C for 30 min applied using Oncothermia. Our data collectively indicate that suppression of HIF-1α is one of the mechanism by which MTH enhances the efficacy of irradiation therapy against tumours, supporting the potential utility of MTH as a means to improve the outcome of SBRT or SRS.
Materials and methods
Tumours
FSaII fibrosarcoma of C3H mice were used. Tumour cells were maintained in vitro in RPMI 1640 supplemented with 10% FBS and 1% antibiotics (5 mg penicillin, 5 mg streptomycin and 10 mg neomycin/ml, Life Technologies, Inc., Carlsbad, CA) under humidified 5% CO2 at 37 °C. Exponentially growing FSaII tumour cells in culture were harvested following trypsin treatment and washed three times with PBS. Next, 2 × 105 viable cells suspended in 0.05 ml PBS were injected subcutaneously (s.c.) into each right hind leg of male C3H mice. Tumour diameters were measured with callipers and volumes were calculated with the formula: V = 0.523 × A × B2, where A represents the longest and B the shortest diameter of tumours, respectively. Tumours that reached 6–7 mm in diameter were used for experiments. All animal experiments were conducted following a protocol approved by the Institutional Animal Care and Use Committee.
Mice
C3H mice (5 weeks old) were purchased from Orient Bio Co. (Seongnam, Korea) and allowed to acclimatise to the new environment for one week before use for experiments. Animal room temperature and relative humidity were maintained at 22 ± 3 °C and 50 ± 20%, respectively. Mice were provided water and food (Purina, St. Louis, MA) ad libitum.
Irradiation
Mice were lightly anaesthetised with tiletamine/zolazepam (Virbac Zoletil™ 50; Virbac Lab., Carros, France) and the tumour-bearing legs were irradiated with a 60Co unit (Thermatron 780, Atomic Energy of Canada) at a dose rate of 1.3 Gy/min.
Heating
Mice were lightly anaesthetised with tiletamine/zolazepam, and tumours were heated using an experimental Oncothermia unit provided by HOSPICARE Co., Ltd. (Seoul, Korea) (LAB-EHY100, OncoTherm, Budapest, Hungary) equipped for capacitive heating of mouse tumours with amplitude-modulated radiofrequency of 13.56 MHz [Citation47]. Details of Oncothermia heating have been described in previous reports [Citation47–49]. Tumour temperatures were measured with an optical thermal probe (Luxtron FOT Lab Kit, LumaSense Technologies, Inc., Santa Clara, CA) inserted into the centre of tumours. Tumours were heated by applying power of 2–3 W, which increased the temperature to 41 °C in 10 min. The temperature was maintained at 41 °C for 30 min, following which the RF power was turned off. Therefore, although the total heating period was 40 min, the duration of heating at 41 °C was 30 min. The temperature of skin over the subcutaneous tumours was measured by placing a thermal probe between the skin and upper electrode coupled to the tumours. A thermal probe was additionally placed between mice and the lower electrical plate on which mice were placed. Tumours used for core temperature measurements were not used for further experiments, since potential tissue damage caused by insertion of thermal probes could affect the results. However, the temperature over the tumours was always measured to ensure that the tumours were properly heated.
Tumour growth study
Tumour-bearing mice were randomly divided into six groups with 7–9 tumours per group as follows: (a) control, (b) irradiation, (c) one heating, (d) three heatings at 2-day intervals (on days 0, 2 and 4), (e) irradiation followed immediately with one heating and (f) irradiation and three heatings at 2-day intervals. We additionally examined the effects of MTH before tumour irradiation to compare the relative effectiveness of heating tumours before and after irradiation.
Analysis of apoptosis
Tumours were randomly divided into four groups with 4–5 tumours per group as follows: (a) control, (b) irradiation, (c) one heating and (d) one heating immediately after irradiation. Apoptosis was assessed following a previously reported method [Citation50,Citation51]. Three days after treatment, tumours were excised and placed in neutral-buffered formalin. After paraffin embedding, tumours were cut into 4 μm sections, mounted on silane-coated slides, deparaffinised and stained with ApopTag peroxidase with an in situ apoptosis detection staining kit (S7100, Merck Millipore, Billerica, MA). Apoptotic cells positive for terminal deoxynucleotidyl transferase-mediated dUTP-biotin nick end labelling (TUNEL) were counted at 400× magnification. TUNEL-positive cells were considered apoptotic only when they exhibited characteristic morphology [Citation50,Citation51].
Immunohistochemical assessment of HIF-1α, VEGF and CA-9
Tumours were randomly divided into four groups with 4–5 tumours per group: (a) control, (b) irradiation, (c) one heating and (d) one heating immediately after irradiation. Three days after treatment, tumours were excised and placed in neutral-buffered formalin, embedded in paraffin and cut into 4 μm sections. Tissue sections were mounted on silane-coated slides, deparaffinised and boiled for 10 min in 0.01 M citrate buffer (pH 6.0) to retrieve antigen. Sections were maintained overnight at 4 °C in solutions containing the appropriate concentrations of antibodies against HIF-1α (sc-10790; Santa Cruz Biotechnology, Dallas, TX), VEGF (ab1316; Abcam, San Francisco, CA) and anti-carbonic anhydrase IX (CA9) (ab15086; Abcam, San Francisco, CA). After washing three times with PBS, sections were incubated with peroxidase reagent and anti-mouse IgG (ImmPRESS™; VECTOR laboratories, Inc., Burlingame, CA) for 20 min. Following three washes with PBS, peroxidase-binding sites were stained with diaminobenzidine (DAB; VECTOR Laboratories, Inc., Burlingame, CA), counterstained with Mayer's haematoxylin, and examined under a light microscope.
Assessment of blood perfusion and tumour hypoxia
Tumours were randomly divided into four groups with 4–5 tumours per group: (a) control, (b) irradiation, (c) one heating and (d) one heating immediately after irradiation. Three days after treatment, tumour-bearing mice were injected intraperitoneally with 75 mg/kg pimonidazole (Chemicon Int, Temecula, CA) in 0.1 ml saline [Citation52]. One hour later, 2 mg/kg Hoechst 33342 (H3570, Thermo Fisher scientific, Waltham, MA) in 0.05 ml of saline was injected intravenously through the tail vein and mice were sacrificed 2 min later. Tumours were excised, immediately frozen, cut into 5 μm sections and treated with anti-pimonidazole antibody (mouse fluorescein isothiocyanate-monoclonal antibody diluted 1:50; Hypoxyprobe, Inc., Burlington, MA). Hypoxic areas indicated by green pimonidazole fluorescence and perfused blood vessels filled with blue Hoechst 33342 fluorescence dye were quantitated via confocal fluorescence microscopy (Olympus FV1000, Melville, NY).
Statistical analysis
All results are expressed as means ± SE. Student’s t-test was used for statistical comparisons. All tests were two-sided, and p values less than 0.05 considered significant.
Results
Tumour temperature
shows an example of changes in temperature of FSaII tumours during heating with the LAB-EHY100 Oncothermia unit. Tumour temperature increased from ∼32 °C before heating to ∼41 °C within 10 min. Tumours were heated for 30 min at 41 ± 0.2 °C and the RF power was turned off. The temperature of skin over the subcutaneous tumour increased and remained at 36 °C during the tumour heating process. The body surface temperature gradually increased from 25 °C to ∼29 °C.
Figure 1. Temperature profile during heating of FSaII tumours with Oncothermia. T1: Intratumour temperature determined with a thermal probe inserted into the middle of tumour, T2: temperature of the skin surface over subcutaneous tumours determined by placing a thermal probe between the tumour and upper heating electrode, T3: temperature of the skin surface determined by placing a thermal probe between the mouse and lower electrical plate on which the mouse was placed.
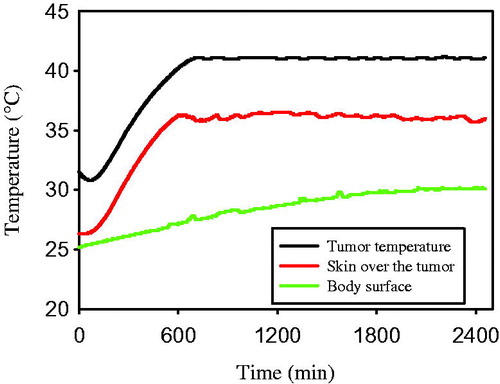
Tumour growth
The effects of various treatments on growth of subcutaneous FSaII tumours in legs of C3H mice are shown in . Treatments were initiated when the tumour volume reached 130–150 mm3. Control tumours progressively increased in size once they reached 400–500 mm3. The growth of tumours treated with a single heating at 41 °C for 30 min was slower than that of control tumours (p < 0.05). Repeated heating three times on days 0, 2 and 4 was slightly more effective than single heating in suppressing tumour growth. Irradiation of tumours with 15 Gy in a single exposure induced marked suppression of tumour growth. Consequently, the volume of untreated control tumours increased 10 times in about 11 days while that of irradiated tumours increased 10 times in 16 days. The growth of tumours irradiated and then immediately heated once was significantly slower than that of tumours treated with irradiation or heating alone (p < 0.05). Heating the tumours three times after irradiation was the most effective in suppressing growth relative to the other treatments, i.e. irradiation alone or irradiation with one heating. A 10-fold increase in volume of tumours treated with irradiation in combination with three heating cycles was observed at 20 days.
Figure 2. Changes in tumour volume after various treatments. Data represent mean volumes of 7–9 tumours ±1 SE. *indicates p < 0.05.
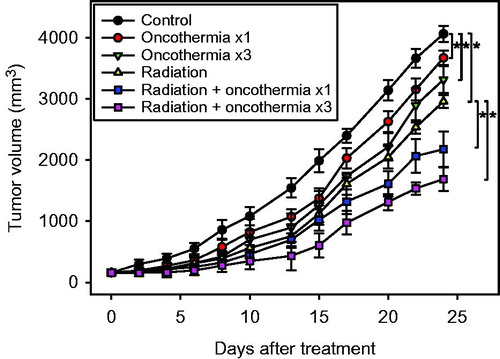
We additionally examined the effects of heating tumours immediately before irradiation on tumour growth. Our data showed that application of heat treatment before irradiation is more effective than that after irradiation () to a small but statistically significant extent (p < 0.05).
Apoptosis
Induction of apoptosis in FSaII tumours at the 3-day time point after various treatments is depicted in . Representative sections showing TUNEL-positive apoptotic cells from each treatment group are presented in and the percentage of apoptotic cells (apoptotic index) in . In control tumours, 5.5 ± 1.6% cells were apoptotic, and irradiation with 15 Gy increased the apoptotic index to 17.0 ± 2.4% (p < 0.05). Three days after heating at 41 °C for 30 min with Oncothermia, the apoptotic index increased to 9.1 ± 1.6%, which was also significantly greater than that in control tumours (p < 0.05). The apoptotic index in tumours irradiated and heated once immediately afterwards was increased up to 25.5 ± 2.7% in 3 days (p < 0.05).
Figure 4. (A) TUNEL-positive apoptotic cells in FSaII tumours three days after various treatments. Arrows indicate apoptotic cells. (B) Apoptotic index (%) three days after treatments. The number of apoptotic cells (total of 1000 nuclei) in 10 fields randomly selected in each of three tissue sections per tumour was obtained. Means of five tumours ±1 SE are shown. *indicates p < 0.05.
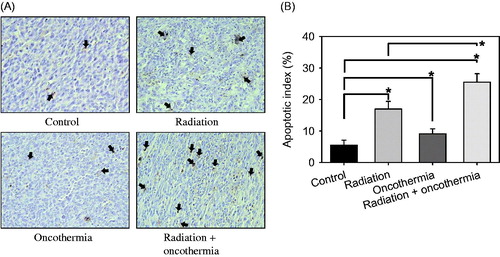
Blood perfusion and hypoxia in tumours
Changes in blood perfusion and hypoxia in FSaII tumours caused by various treatments are shown in . Areas stained with Hoechst 33342 (blue colour) indicate perfused blood vessels and those stained with pimonidazole (green colour) represent hypoxic areas (). The percentages of areas positive for blood perfusion and hypoxia are presented in . Three days after irradiation of tumours with 15 Gy in a single exposure, the size of the Hoechst 33342-positive area declined to almost half that in control tumours, indicating significant disruption of tumour blood perfusion. The size of pimonidazole-positive areas almost doubled in two days after irradiation with 15 Gy, suggesting a marked increase in tumour hypoxia (p < 0.05). Three days after heating at 41 °C for 30 min with Oncothermia, the size of Hoechst 33342-positive areas increased (p < 0.05) and pimonidazole-positive areas decreased (p < 0.05) to a significant extent, supporting the theory that MTH improves tumour oxygenation by increasing blood perfusion. Importantly, heating tumours for 30 min at 41 °C immediately after 15 Gy irradiation induced a slight decrease in the size of Hoechst 33342-positive areas and increase in pimonidazole-positive areas relative to those in control tumours. Our data clearly demonstrate that 15 Gy irradiation impairs blood perfusion and increases hypoxia in tumours whereas heating at 41 °C markedly improves blood perfusion and decreases hypoxia. When tumours were irradiated and heated once, blood perfusion was greater and hypoxia was lower than that in tumours receiving irradiation only.
Figure 5. (A) Immunofluorescence images of hypoxia and blood perfusion in FSaII tumours three days after treatment. Radiation: Tumours were irradiated with 15 Gy. Oncothermia: Tumours were heated once at 41 °C for 30 min. Radiation + Oncothermia: Tumours were irradiated with 15 Gy and immediately heated once at 41 °C for 30 min. Hypoxic areas were labelled with pimonidazole (green) and blood perfusion assessed using Hoechst 33342 (blue). (B) Areas positive for Hoechst 33342 (perfusion) and pimonidazole (hypoxia) in tissue sections were quantified using Image J software. Five fields in each of the three tissue sections per tumour were studied and the % positive area calculated. Means of five tumours ±1 SE are shown. *indicates p < 0.05.
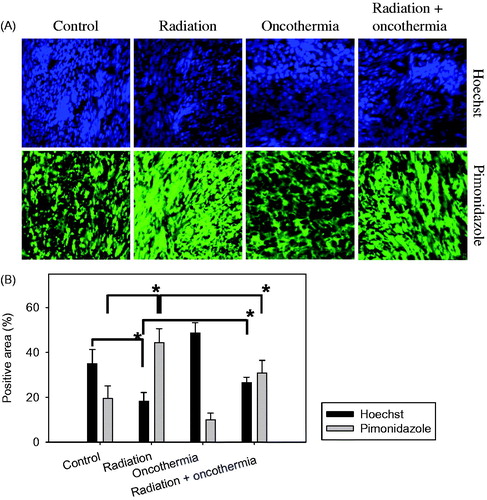
Expression of HIF-1α and target genes
Immunohistological staining for HIF-1α and its target genes, CA9 and VEGF, in FSaII tumours three days after various treatment regimens are shown in . The percentages of areas positive for HIF-1α, CA9 and VEGF are presented in . Irradiation with 15 Gy markedly elevated HIF-1α, VEGF and CA9 expression (p < 0.05). On the other hand, 30 min heating at 41 °C reduced the levels of HIF-1α and VEGF (p < 0.05) while having little effect on expression of CA9. The increases in HIF-1α, CA9 and VEGF expression caused by 15 Gy irradiation were significantly nullified by one cycle of post-irradiation heating at 41 °C for 30 min (p < 0.05) so that HIF-1α and VEGF levels in tumours treated with both irradiation and heating were similar to those in control tumours.
Figure 6. (A) Immunohistological staining for HIF-1α, CA9 and VEGF in FSaII tumours three days after treatment. Radiation: Tumours were irradiated with 15 Gy. Oncothermia: Tumours were heated once at 41 °C for 30 min. Radiation + Oncothermia: Tumours were irradiated with 15 Gy and heated once at 41 °C for 30 min (B). Expression of HIF-1α, VEGF and CA9 was quantified using Image J 1.38× software (Rasband, W.S., Image J, US National Institutes of Health, Bethesda, MD). Ten images were captured for each of the three tissue sections per tumour and % positive areas calculated. Means of 4–6 tumours ±1 SE are shown. *indicates p < 0.05.
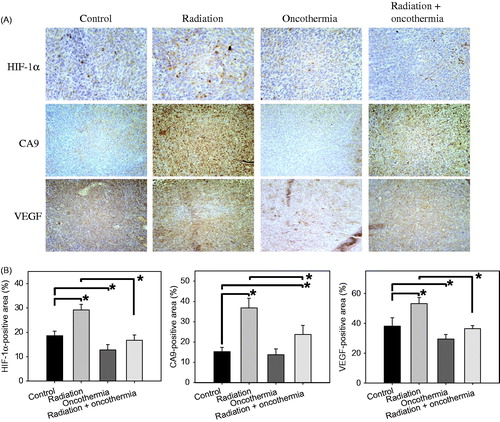
Discussion
MTH applied with Oncothermia enhanced the radiation-induced growth delay of FSaII fibrosarcoma in legs of C3H mice. MTH increased the cytotoxic effect of radiation, as evident from the increase in apoptosis, and suppressed radiation-induced upregulation of HIF-1α and its target genes, such as VEGF. Suppression of HIF-1α and target genes may be one of the mechanisms by which MTH enhances the response of tumours to high-dose irradiation.
A single heating of FSaII tumours at 41 °C for 30 min caused visible tumour growth delay and heating the tumours three times with two-day intervals was slightly more effective than the single heating in suppressing tumour growth (). Administration of the single heating immediately after irradiation significantly enhanced the radiation-induced tumour growth delay and, heating the tumours three times was more effective than the single heating in promoting tumour growth delay. As shown in , apoptosis in FSaII tumours irradiated and immediately heated afterwards was far greater than that in tumours treated with irradiation or heating alone. Notably, apoptosis is only one of the many cell death modes triggered after various treatments, including irradiation and hyperthermia. Thus, it is reasonable to assume that total tumour cell death caused by heating, irradiation or a combination of both is greater than the apoptotic index shown in , and that tumour cell death by other modes, in addition to apoptosis, contribute significantly to tumour growth delay.
The marked decrease in blood perfusion after 15 Gy irradiation in FSaII tumours () is consistent with numerous reports that high-dose hypofractionated irradiation causes severe vascular damage in experimental and human tumours [Citation28–33]. In view of this decline in blood perfusion, it is not surprising that intratumour hypoxia is markedly increased under these conditions (). The aberrant increase in HIF-1α and its target genes, VEGF and CA9, in FSaII tumours observed in response to 15 Gy irradiation () is in agreement with previous reports that high-dose irradiation induces significant upregulation of HIF-1α in various tumours [Citation31,Citation35–40,Citation43]. In the context of radiation-induced upregulation of HIF-1α, studies to date have shown that hypoxic cells in tumours are reoxygenated after irradiation and HIF-1α is activated in response to the reactive oxygens formed [Citation36]. Irradiation stabilises HIF-1α, even in normoxic conditions, through activation of p38 MAPX [Citation39]. While it has been established that enduring elevation of hypoxia due to severe reduction in blood perfusion in FSaII tumours prevents HIF-1α ubiquitination and proteasome-mediated degradation, the mechanisms underlying the upregulation of HIF-1α in FSaII tumours in response to 15 Gy irradiation are currently unclear.
Increased blood perfusion and tumour oxygenation (reduction of hypoxia) in FSaII tumours induced by MTH at 41 °C for 30 min in the present study () are consistent with numerous previous observations that heating tumours to mild temperatures of 39–42 °C leads to significantly elevated tumour blood perfusion and oxygenation [Citation13–18]. Heating at 41 °C for 30 min resulted in a small decline in HIF-1α, CA9 and VEGF expression in FSaII tumours, as shown in . In a previous report [Citation53], HIF-1α expression increased after heating at 43 °C for 1 h, peaked at 6 h and declined to control levels in 24–48 h, while VEGF peaked at 48 h after heating in a mouse tumour model. The investigators concluded that reactive oxygen species (ROS) produced through mediation of NADPH oxidase activate HIF-1 [Citation53]. In a hepatic carcinoma model of rabbit, residual tumour cells that survived radiofrequency ablative heating overexpressed various growth stimulants, including HIF-1α and VEGF [Citation54]. An interesting and potentially important observation in our present study was that MTH only slightly reduced expression of HIF-1α and VEGF but significantly inhibited upregulation of HIF-1α and VEGF caused by 15 Gy irradiation (). Under aerobic conditions or in the presence of oxygen, HIF-1α is hydroxylated and binds VHL protein, which promotes its ubiquitination and proteasome-mediated degradation [Citation45,Citation46]. In view of the findings of the present study, it is highly likely that the increase in intratumour oxygenation caused by MTH triggers degradation of HIF-1α that is upregulated by high-dose irradiation. Considering HIF-1α is the master transcriptional regulator of various pathophysiologic processes, including angiogenesis and vasculogenesis, it would be reasonable to conclude that MTH-induced suppression of HIF-1α and VEGF is responsible, at least in part, for enhancement of tumour growth delay after 15 Gy irradiation ().
As shown in , heating FSaII tumours at 41 °C for 30 min immediately before 15 Gy irradiation was slightly more effective than heating after irradiation in suppressing tumour growth, probably because prior heating increases tumour oxygenation, thereby augmenting the response of tumours to irradiation via enhancing the production of ROS. Evidently, when tumours were heated after irradiation, such an increase in tumour oxygenation was not involved in enhancement of the radiation-induced tumour growth delay (). One possibility is that MTH applied after 15 Gy irradiation promotes radiation-induced cell death by inhibiting repair of sublethal radiation damage, as indicated by the significant increase in apoptosis (). Additionally, it would be reasonable to suggest that inhibition of radiation-induced upregulation of HIF-1α and VEGF plays a role in the suppression of tumours by MTH applied after irradiation. Further studies using tumour cell lines expressing different levels of HIF-1α are warranted to determine the precise role of MTH-induced downregulation of HIF-1α in tumour response to irradiation.
Acknowledgements
This research was supported by the National R&D Program through the Korea Institute of Radiological and Medical Sciences funded by the Ministry of Science, ICT and Future Planning (No. 1711045549/50541–2016), and a research fund from the HOSPICARE Co., Ltd. (Seoul, Korea) (51304–2016).
Disclosure statement
No potential conflict of interest was reported by the authors.
Additional information
Funding
References
- Dewey WC, Hopwood LE, Sapareto SA, Gerweck LE. (1977). Cellular responses to combinations of hyperthermia and radiation. Radiology 123:463–74.
- Sapareto SA, Hopwood LE, Dewey WC. (1978). Combined effects of X-irradiation and hyperthermia on CHO cells for various temperatures and orders of application. Radiat Res 73:221–33.
- Overgaard J, Gonzalez Gonzalez D, Hulshof MC, et al. (1995). Randomised trial of hyperthermia as adjuvant to radiotherapy for recurrent or metastatic malignant melanoma. Lancet 345:540–3.
- Harima Y, Nagata K, Harima K, et al. (2001). A randomized clinical trial of radiation therapy versus thermoradiotherapy in stage IIIB cervical carcinoma. Int J Hyperthermia 17:97–105.
- Vernon CC, Hand JW, Field SB, et al. (1996). Radiotherapy with or without hyperthermia in the treatment of superficial localized breast cancer: results from five randomized controlled trials. Int J Radiat Oncol Biol Phys 35:731–44.
- Sneed PK, Stauffer PR, McDermott MW, et al. (1998). Survival benefit of hyperthermia in a prospective randomized trial of brachytherapy boost +/− hyperthermia for glioblastoma multiforme. Int J Radiat Oncol Biol Phys 40:287–95.
- Wust P, Hildebrandt B, Sreenivasa G, et al. (2002). Hyperthermia in combined treatment of cancer. Lancet Oncol 3:487–97.
- Datta NR, Ordonez SG, Gaipl US, et al. (2015). Local hyperthermia combined with radiotherapy and-/or chemotherapy: recent advances and promises for the future. Cancer Treat Rev 41:742–53.
- Issels RD, Abdel-Rahman S, Wendtner C, et al. (2001). Neoadjuvant chemotherapy combined with regional hyperthermia (RHT) for locally advanced primary or recurrent high-risk adult soft-tissue sarcomas (STS) of adults: long-term results of a phase II study. Eur J Cancer 37:1599–608.
- Falk MH, Issels RD. (2001). Hyperthermia in oncology. Int J Hyperthermia 17:1–18.
- Song CW. (1984). Effect of local hyperthermia on blood flow and microenvironment: a review. Cancer Res 44:4721s–40s.
- Kang MS, Song CW, Levitt SH. (1980). Role of vascular function in response of tumors in vivo to hyperthermia. Cancer Res 40:1130–5.
- Iwata K, Shakil A, Hur WJ, et al. (1996). Tumour pO2 can be increased markedly by mild hyperthermia. Br J Cancer Suppl 27:S217–21.
- Song CW, Park HJ, Lee CK, Griffin R. (2005). Implications of increased tumor blood flow and oxygenation caused by mild temperature hyperthermia in tumor treatment. Int J Hyperthermia 21:761–7.
- Vujaskovic Z, Song CW. (2004). Physiological mechanisms underlying heat-induced radiosensitization. Int J Hyperthermia 20:163–74.
- Song CW, Shakil A, Osborn JL, Iwata K. (2009). Tumour oxygenation is increased by hyperthermia at mild temperatures. Int J Hyperthermia 25:91–5.
- Dewhirst MW, Vujaskovic Z, Jones E, Thrall D. (2005). Re-setting the biologic rationale for thermal therapy. Int J Hyperthermia 21:779–90.
- Brizel DM, Scully SP, Harrelson JM, et al. (1996). Radiation therapy and hyperthermia improve the oxygenation of human soft tissue sarcomas. Cancer Res 56:5347–50.
- Evans SS, Wang WC, Bain MD, et al. (2001). Fever-range hyperthermia dynamically regulates lymphocyte delivery to high endothelial venules. Blood 97:2727–33.
- Frey B, Weiss EM, Rubner Y, et al. (2012). Old and new facts about hyperthermia-induced modulations of the immune system. Int J Hyperthermia 28:528–42.
- Repasky EA. (2013). Progress in development of biomedical applications of heat shock proteins and thermal stress. Int J Hyperthermia 29:359–61.
- Repasky EA, Evans SS, Dewhirst MW. (2013). Temperature matters! And why it should matter to tumor immunologists. Cancer Immunol Res 1:210–16.
- Evans SS, Repasky EA, Fisher DT. (2015). Fever and the thermal regulation of immunity: the immune system feels the heat. Nat Rev Immunol 15:335–49.
- Kavanagh BD. (2014). Stereotactic body radiation therapy as a derivative of stereotactic radiosurgery: clinically independent but with enduring common themes. J Clin Oncol 32:2827–31.
- Timmerman RD, Herman J, Cho LC. (2014). Emergence of stereotactic body radiation therapy and its impact on current and future clinical practice. J Clin Oncol 32:2847–54.
- Nagata Y. (2013). Stereotactic body radiotherapy for early stage lung cancer. Cancer Res Treat 45:155–61.
- Jang WI, Kim MS, Bae SG, et al. (2013). High-dose stereotactic body radiotherapy correlates increased local control and overall survival in patients with inoperable hepatocellular carcinoma. Radiat Oncol 8:250.
- Park HJ, Griffin RJ, Hui S, et al. (2012). Radiation-induced vascular damage in tumors: implication of vascular damage in ablative hypofractionated radiotherapy (SBRT and SRS). Radiat Res 177:311–27.
- Song CW, Kim MS, Cho LC, et al. (2014). Radiobiological basis of SBRT and SRS. Int J Clin Oncol 19:570–8.
- Kim MS, Kim W, Park IH, et al. (2015). Radiobiological mechanisms of stereotactic body radiation therapy and stereotactic radiation surgery. Radiat Oncol J 33:265–75.
- Song CW, Lee YJ, Griffin RJ, et al. (2015). Indirect tumor cell death after high-dose hypofractionated irradiation: implications for stereotactic body radiation therapy and stereotactic radiation surgery. Int J Radiat Oncol Biol Phys 93:166–72.
- Solesvik OV, Rofstad EK, Brustad T. (1984). Vascular changes in a human malignant melanoma xenograft following single-dose irradiation. Radiat Res 98:115–28.
- El Kaffas A, Gilles A, Czarnota GJ. (2013). Dose-dependent response of tumor vasculature to radiation therapy in combination with Sunitinib depicted by three-dimensional high-frequency power Doppler ultrasound. Angiogenesis 16:443–54.
- Maeda A, Chen Y, Bu J, et al. (2017). In vivo imaging reveals significant tumor vascular dysfunction and increased tumor hypoxia-inducible factor-1α expression induced by high single-dose irradiation in a pancreatic tumor model. Int J Radiat Oncol Biol Phys 97:184–94.
- Harada H, Inoue M, Itasaka S, et al. (2012). Cancer cells that survive radiation therapy acquire HIF-1 activity and translocate towards tumour blood vessels. Nat Commun 3:783.
- Moeller BJ, Cao Y, Li CY, Dewhirst MW. (2004). Radiation activates HIF-1 to regulate vascular radiosensitivity in tumors: role of reoxygenation, free radicals, and stress granules. Cancer Cell 5:429–41.
- Mimeault M, Batra SK. (2013). Hypoxia-inducing factors as master regulators of stemness properties and altered metabolism of cancer- and metastasis-initiating cells. J Cell Mol Med 17:30–54.
- Semenza GL. (1998). Hypoxia-inducible factor 1: master regulator of O2 homeostasis. Curr Opin Genet Dev 8:588–94.
- Kim YH, Yoo KC, Cui YH, et al. (2014). Radiation promotes malignant progression of glioma cells through HIF-1alpha stabilization. Cancer Lett 354:132–41.
- Zhang M, Qui Q, Li Z, et al. (2015). HIF-1alpha regulate the response of primary sarcomas to radiation therapy through a cell autonomous mechanisms. Radiat Res 183:594–609.
- Barker HE, Paget JT, Khan AA, Harrington KJ. (2015). The tumour microenvironment after radiotherapy: mechanisms of resistance and recurrence. Nat Rev Cancer 15:409–25.
- Tepeköylü C, Wang FS, Kozaryn R, et al. (2013). Shock wave treatment induces angiogenesis and mobilizes endogenous CD31/CD34-positive endothelial cells in a hindlimb ischemia model: implications for angiogenesis and vasculogenesis. J Thorac Cardiovasc Surg 146:971–8.
- Rankin EB, Giaccia AJ. (2016). Hypoxic control of metastasis. Science 352:175–80.
- Ceradini DJ, Kulkarni AR, Callaghan MJ, et al. (2004). Progenitor cell trafficking is regulated by hypoxic gradients through HIF-1 induction of SDF-1. Nat Med 10:858–64.
- Maxwell PH, Wiesener MS, Chang GW, et al. (1999). The tumour suppressor protein VHL targets hypoxia-inducible factors for oxygen-dependent proteolysis. Nature 399:271–5.
- Ohh M, Park CW, Ivan M, et al. (2000). Ubiquitination of hypoxia-inducible factor requires direct binding to the beta-domain of the von Hippel–Lindau protein. Nat Cell Biol 2:423–7.
- Jeon TW, Yang H, Lee CG, et al. (2016). Electro-hyperthermia up-regulates tumour suppressor septin 4 to induce apoptotic cell death in hepatocellular carcinoma. Int J Hyperthermia 32:648–56.
- Hegyi G, Szasz O, Szasz A. (2009). Oncothermia treatment of cancer: from the laboratory to clinic. Electromagn Biol Med 28:148–65.
- Hegyi G, Szigeti GP, Szasz A. (2013). Hyperthermia versus oncothermia: cellular effects in complementary cancer therapy. Evid Based Complement Alternat Med 2013:672873.
- Kim W, Seong J, An JH, Oh HJ. (2007). Enhancement of tumor radioresponse by wortmannin in C3H/HeJ hepatocarcinoma. J Radiat Res 48:187–95.
- Kim W, Seong J, Oh HJ, et al. (2011). A novel combination treatment of armed oncolytic adenovirus expressing IL-12 and GM-CSF with radiotherapy in murine hepatocarcinoma. J Radiat Res 52:646–54.
- Dings RP, Loren M, Heum H, et al. (2007). Scheduling of radiation with angiogenesis inhibitors anginex and Vastin improves therapeutic outcome via vessel normalization. Clin Cancer Res 13:3395–402.
- Moon EJ, Sonveaux P, Porporato PE, et al. (2010). NADPH oxidase-mediated reactive oxygen species production activates hypoxia-inducible factor-1 (HIF-1) via the ERK pathway after hyperthermia treatment. PNAS 107:20477–82.
- Ke S, Ding XM, Kong J, et al. (2010). Low temperature of radiofrequency ablation at the target sites can facilitate rapid progression of residual hepatic VX2 carcinoma. J Transl Med 8:73.