Abstract
Purpose: Thermoradiotherapy is an effective treatment for locally advanced cervical cancer. However, the optimal time interval between radiotherapy and hyperthermia, resulting in the highest therapeutic gain, remains unclear. This study aims to evaluate the effect of time interval on the therapeutic gain using biological treatment planning.
Methods: Radiotherapy and hyperthermia treatment plans were created for 15 cervical cancer patients. Biological modeling was used to calculate the equivalent radiation dose, that is, the radiation dose that results in the same biological effect as the thermoradiotherapy treatment, for different time intervals ranging from 0–4 h. Subsequently, the thermal enhancement ratio (TER, i.e. the ratio of the dose for the thermoradiotherapy and the radiotherapy-only plan) was calculated for the gross tumor volume (GTV) and the organs at risk (OARs: bladder, rectum, bowel), for each time interval. Finally, the therapeutic gain factor (TGF, i.e. TERGTV/TEROAR) was calculated for each OAR.
Results: The median TERGTV ranged from 1.05 to 1.16 for 4 h and 0 h time interval, respectively. Similarly, for bladder, rectum and bowel, TEROARs ranged from 1–1.03, 1–1.04 and 1–1.03, respectively. Radiosensitization in the OARs was much less than in the GTV, because temperatures were lower, fractionation sensitivity was higher (lower α/β) and direct cytotoxicity was assumed negligible in normal tissue. TGFs for the three OARs were similar, and were highest (around 1.12) at 0 h time interval.
Conclusion: This planning study indicates that the largest therapeutic gain for thermoradiotherapy in cervical cancer patients can be obtained when hyperthermia is delivered immediately before or after radiotherapy.
Introduction
Cervical cancer is the fourth most common cancer in women worldwide, with 528 000 new cases and 266 000 deaths in 2012 [Citation1]. Clinical studies have shown that thermoradiotherapy, that is, radiotherapy combined with hyperthermia, is an effective treatment for advanced stage cervical cancer [Citation2–4]. With comparable clinical outcomes, thermoradiotherapy provides a good alternative for the standard treatment with chemoradiotherapy for patients with a contraindication for chemotherapy [Citation2–5].
A clinical thermoradiotherapy treatment for advanced cervical cancer consists of daily fractionated external beam radiotherapy, once or twice a week preceded or followed by hyperthermia [Citation2]. During hyperthermia the tumor is heated to 40–43 °C for one hour, using a locoregional heating device. These temperatures are known to radiosensitize the tumor through several mechanisms, such as suppression of DNA damage repair [Citation6,Citation7] and increased oxygenation [Citation8–11]. Also, hyperthermia may cause direct cytotoxicity [Citation12–15].
The effect of hyperthermia is known to depend on the temperature distribution and the time interval between radiotherapy and hyperthermia [Citation16–18]. Clinically applied time intervals between radiotherapy and hyperthermia in the treatment of cervical cancer vary substantially: a recent retrospective study showed that the majority of time intervals at the AMC were between 1–1.5 h [Citation17], while in the Dutch Deep Hyperthermia trial time intervals ranged from 30 min to 4 h [Citation3]. This raises the question which time interval provides the highest therapeutic gain.
In his seminal paper, Overgaard showed through in vivo experiments that the thermal enhancement ratio, a measure of the degree of radiosensitization, is highest when hyperthermia and radiotherapy are delivered simultaneously [Citation16]. However, the same study showed that this was the case for both normal and tumor tissue and thus predicted no therapeutic gain for such a (nearly) simultaneous treatment delivery schedule. For longer time intervals, a positive therapeutic gain could be obtained (with an optimum at 4 h), because the radiosensitization in normal tissue was shown to disappear more rapidly with an increasing time interval. Along with practical considerations, these findings have most likely inspired the development of the current clinical schedules in which time intervals of one to several hours are common.
The 4-h time interval found to be optimal in Overgaard’s experiments was based on the assumption that tumor and normal tissues are heated to the same temperature levels. However, clinical temperature distributions are heterogeneous and are known to depend in a complex way on the patient geometry [Citation19,Citation20]. Consequently, the radiosensitizing effect may vary significantly between the tumor and the organs at risk (bladder, rectum and bowel) and this may affect which time interval results in optimal therapeutic gain. Derivation of an optimal time interval requires a planning study that models radiosensitization, taking into account the heterogeneous radiation dose and temperature distributions. Radiosensitization can be modelled using an extended version of the LQ-model with temperature-dependent radiosensitivity parameters (α and β) [Citation18,Citation21–23]. This can be used to calculate the equivalent radiation dose (EQDRT), the dose needed in a radiotherapy-only treatment to obtain the same biological effect as the combined radiotherapy and hyperthermia treatment [Citation24]. Recently, we developed software that allows calculation of equivalent radiation dose distributions in patients at the voxel level, based on planned radiation dose and temperature distributions [Citation24], enabling us to investigate the effect of different time intervals between radiotherapy and hyperthermia.
The aim of this planning study is to predict what time interval between radiotherapy and hyperthermia provides the highest therapeutic gain for cervical cancer patients, taking into account clinically realistic (i.e. heterogeneous) temperature distributions. This is achieved by evaluating the effect of time interval between radiotherapy and hyperthermia on several DVH indices and on the thermal enhancement ratio for the GTV and organs at risk.
Materials and methods
This planning study included 15 advanced stage cervical cancer patients who received thermoradiotherapy at the AMC between 2009 and 2016. For these patients, equivalent radiation dose distributions were calculated for the thermoradiotherapy treatment plans for different time intervals between radiotherapy and hyperthermia (0 h, 0.5 h, 1 h, 1.5 h, 2.5 h and 4 h) using the following procedure described in Kok et al. [Citation23].
First, radiation dose and temperature distributions were calculated based on the respective treatment plans for radiotherapy and hyperthermia (). Then, the radiotherapy and hyperthermia planning CTs were matched using deformable image registration and the radiation dose distribution was warped to the frame of reference of the hyperthermia CT. Finally, the equivalent dose distribution was calculated [Citation25].
Figure 1. Workflow for equivalent radiation dose calculation of a combined treatment with radiotherapy (RT) and hyperthermia (HT). Reproduced from Crezee et al. [Citation23] with permission.
![Figure 1. Workflow for equivalent radiation dose calculation of a combined treatment with radiotherapy (RT) and hyperthermia (HT). Reproduced from Crezee et al. [Citation23] with permission.](/cms/asset/8fddf43a-2c08-4641-8ee3-b2f02a7f3334/ihyt_a_1468930_f0001_c.jpg)
Hyperthermia treatment planning
The hyperthermia treatment planning CT was acquired in supine treatment position on a water bolus and mattresses. Although all original hyperthermia treatment plans were made for treatment with the 70 MHz AMC-4 system [Citation26], the plans varied in resolution and optimization strategy Therefore, new treatment plans were created for this study according to a single, uniform protocol. Based on the planning CT, a segmented anatomy was created at a resolution of 2.5 × 2.5 × 2.5 mm3 with the dielectric and thermal tissue properties as described in [Citation25]. Treatment planning was performed using the non-commercial software package Plan2Heat, developed at the AMC [Citation27]. Treatment plans were created and optimized for the highest possible T90 within the GTV (i.e. the temperature received in at least 90% of the tumor volume), while constraining normal tissue temperatures to 45 °C.
Radiotherapy treatment planning
Radiation treatment consisted of 28 × 1.8 Gy or 23 × 2 Gy external beam radiotherapy for patients with and without paraortal lymph node involvement, respectively, followed by a 24 Gy brachytherapy boost (PDR). A 3 D conformal technique and, since 2014, a VMAT technique has been used for external beam radiotherapy. Both techniques might yield different dose distributions, particularly in the organs at risk. Therefore, new VMAT plans were created for this study for the eight patients treated before 2014. In one patient, the PTV in the cranial-caudal direction was too large to cover with a VMAT plan, and the clinically delivered IMRT plan was used. Four patients were planned and treated in prone position on a belly board. This practice changed in 2009 [Citation28] and the other 11 patients were planned and treated in supine position. At the AMC, hyperthermia is given during the same period as external beam radiotherapy. The enhancement of the brachytherapy dose by hyperthermia is negligible since there is a gap of several days between external beam radiotherapy and the brachytherapy boost. Thus, the brachytherapy dose is not taken into account in the analyses.
Image registration
Deformable image registration was performed using the Velocity registration software (Varian Medical Systems, Palo Alto) to account for anatomical differences between the hyperthermia and radiotherapy planning CTs. The structures GTV, bladder, rectum and bowel should be available on both the radiation and the hyperthermia planning CT scan, delineated according to the same guidelines. Available clinical structure sets were extended and completed for this purpose. A structure-guided deformable image registration was used, aiming to match both the delineations of corresponding structures and the Hounsfield units. Matching and equivalent dose calculation were performed separately for each structure (GTV, bladder, rectum and bowel), to increase the quality of the deformable image registration.
Biological modeling
The X-Term software package, which has been designed for biological evaluation of thermoradiotherapy treatment plans, was used to calculate the equivalent radiation dose distribution (EQDRT) [Citation24]. This software package allows to import clinical radiotherapy and hyperthermia treatment plans in order to calculate the heterogeneous 3 D EQDRT for a user-specified thermoradiotherapy treatment schedule. This EQDRT is also summarized as a dose volume histogram for evaluation. For a more detailed description of the software and validation, the reader is referred to a previous publication [Citation24]. For normal tissue, calculation of EQDRT was based on a previously proposed extension of the LQ-model, in which the radiosensitivity parameters (α and β) depend on the temperature achieved during hyperthermia and the time interval between radiotherapy and hyperthermia [Citation24]. The expression for equivalent radiation dose based on this extended LQ-model is
(1)
with D (Gy) the total (physical) radiation dose, dref (Gy) the fraction size of the reference treatment, G the protraction factor from the generalised LQ-model, α37 (Gy−1) and β37 (Gy−2) the radiation sensitivity parameters without hyperthermia, and α(T, tint) and β(T, tint) the radiation sensitivity parameters with hyperthermia at temperature T and a time interval between radiotherapy and hyperthermia tint (h). Throughout this study, dref = 2 Gy, so that all calculated EQDRT values are comparable to the conventional EQD2. The radiation sensitivity parameters with hyperthermia are given by
(2)
(3)
where α41 = α(41 °C, 0), β41 = β(41 °C, 0) and μ (h−1) is the rate at which the radiosensitizing effect of hyperthermia disappears. Note that these expressions are symmetric in tint = 0 h, that is, the radiosensitization is the same regardless of whether hyperthermia is given before or after radiotherapy. This was based on the symmetry observed in in vitro survival of cervical cancer cells for radiotherapy before and after hyperthermia [Citation18].
The direct cytotoxic effect of hyperthermia in tumor tissue was accounted for by a term based on the Arrhenius relationship [Citation18]. Thus, the expression for equivalent radiation dose becomes
(4)
with ΔS (cal/°C/mole) the entropy of inactivation and ΔH (cal/mole) the inactivation energy of the critical rate-limiting molecules that cause hyperthermic cytotoxicity.
Model parameters
Equivalent dose calculations require parameters describing the radiosensitivity (α37 and α37/β37), radiosensitization (α41/α37, β41/β37, μ) and sensitivity to direct hyperthermic cytotoxicity (ΔS and ΔH) for each tissue. For tumors, these parameters were based on cell survival data after thermoradiotherapy in cervical cancer cell lines [Citation18]. Parameters derived for the SiHa cell line were used (α37 = 0.386 Gy−1, α37/β37 = 17.9 Gy, α41/α37 = 1.73, β41/β37 = 0.41, ΔS = 392.08 cal/°C/mole and ΔH = 147 907.8 cal/mole). As recommended in [Citation18], the rate at which the radiosensitizing effect of hyperthermia disappears (μ) was set to μ = 0.5 h−1, to better match the behavior of in vivo models and clinical data [Citation16,Citation17].
Accurate parameter values are not available for normal tissue but literature suggests a level of radiosensitization similar to tumors when radiotherapy and hyperthermia are given immediately after each other [Citation16]. Moreover, the same data show that the radiosensitizing effect of hyperthermia disappears more quickly in normal tissue. Hence, for normal tissue, similar radiosensitization parameters were assumed (α41/α37 = 1.73, β41/β37 = 0.41), but a faster rate at which hyperthermic radiosensitization disappears was used (μ = 1 h−1, based on an exponential fit to the data in from Overgaard [Citation16]). Additionally, for the ratio α37/β37, a conventional value for normal tissue of 3 Gy was assumed [Citation29].
Analysis of thermoradiotherapy plans
For the radiotherapy treatment plan, the DVH indices V30Gy, V45Gy, Dmean and D2cm3 were calculated for the organs at risk (bladder, rectum and bowel) and the D98%, D2%, Dmean and equivalent uniform dose (EUD, [Citation30]) were calculated for the GTV. For the hyperthermia treatment plan, the temperature-volume indices T10, T50 and T90 representing the temperature achieved in at least 10%, 50% and 90% of the volume of the structure of interest were calculated for GTV, bladder, rectum and bowel [Citation31] to characterize the temperatures achieved in each structure of interest. To characterize the biological effect of the combined treatment with radiotherapy and hyperthermia, the DVH indices were calculated based on the equivalent radiation dose for the thermoradiotherapy plan. The DVH indices for the thermoradiotherapy plans were then normalized using the corresponding DVH indices from the radiotherapy-only plans. For volume-related indices (V30Gy, V45Gy), the difference between the DVH indices for the thermoradiotherapy (RT + HT) plan and the radiotherapy-only (RT-only) plan was calculated (e.g. ΔV45Gy = V45Gy,RT+HT-V45Gy,RT-only). For dose-related indices, the ratio of the DVH indices for the thermoradiotherapy and the radiotherapy-only plan (also known as the thermal enhancement ratio, or TER), was calculated (e.g. TER(Dmean) = Dmean,RT+HT/Dmean,RT-only). Such normalization corrects for any variation between patients in the radiotherapy-only DVH indices and thus shows more clearly the effect of time interval between radiotherapy and hyperthermia.
Finally, the therapeutic gain factor (TGF) was calculated, defined as the ratio of the thermal enhancement ratio for tumor tissue to the thermal enhancement ratio for an organ at risk (OAR), that is, TGF = TERGTV/TEROAR. The therapeutic gain factor was calculated for each of the three organs at risk with the thermal enhancement ratio based on the mean dose (e.g. TGFbladder = TER(Dmean,GTV)/TER(Dmean,bladder)). A therapeutic gain factor larger than 1 indicates that the effect of hyperthermia is larger in the GTV than in the organ at risk, and thus that hyperthermia increases the size of the therapeutic window. In general, a higher therapeutic gain factor is better.
Any biological model is limited by the statistical accuracy of its parameters, which can be represented by a 95% confidence interval for the model parameters and translated into a confidence interval for EQDRT, as described earlier [Citation18]. To verify that this parameter uncertainty did not substantially affect the results of our analysis, a reasonable range of biological parameters was simulated and the confidence intervals on TER(Dmean) (for the GTV and organs at risk), ΔV45Gy (for the organs at risk) and TER(D98%) (for the GTV) at different time intervals were evaluated for a single patient.
Results
The hyperthermia treatment plans show an adequate focusing of heat to the tumor. With a locoregional hyperthermia technique, heating surrounding normal tissue is inevitable, but as a result of optimization temperatures in the organs at risk were typically lower than in the tumor. For example, the median T50 was 40.7 °C for the GTV, compared with 40.1 °C, 39.7 °C and 38.8 °C for the bladder, rectum and bowel, respectively. shows the T90, T50 and T10 for both GTV and OARs.
Figure 2. Temperature-volume indices for each of the structures of interest, as calculated from the hyperthermia treatment plans of 15 patients.
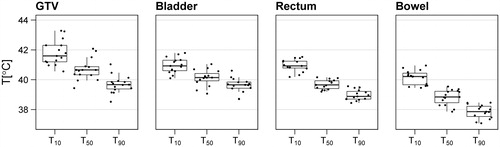
The radiation treatment plans show a good tumor coverage (), with a D98% higher than 95% of the prescribed dose in all patients. For the organs at risk, D2cm3 is close to the prescribed dose in most patients, due to their proximity to the GTV. Median dose is somewhat lower in the bowel, since this structure generally extends well above the GTV in the cranial direction. A large spread is visible in the V45Gy between patients, especially in the bladder and rectum, which is why normalization to the radiotherapy-only DVH indices is needed to evaluate the effect of time interval between radiotherapy and hyperthermia in thermoradiotherapy plans.
Figure 3. DVH indices for each of the structures of interest, as calculated from the radiation treatment plans of 15 patients.
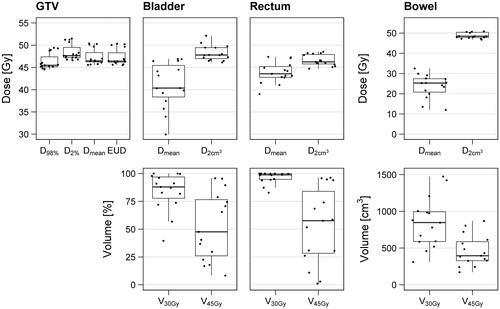
Treatment with a 0-h time interval shows the largest radiosensitizing effect in both the GTV and organs at risk (). The median thermal enhancement ratio for the GTV ranged from 1.05 to 1.16 for 4-h and 0-h time interval, respectively. Similarly, thermal enhancement ratio for bladder ranged from 1 to 1.03, for rectum from 1 to 1.04 and for bowel from 1 to 1.03. As such, the thermal enhancement is much larger in the GTV than in the organs at risk. Moreover, the effect of hyperthermia disappears more rapidly in the organs at risk and has mostly disappeared after approximately 1.5 h. Within the GTV, some enhancement by hyperthermia is still visible after 4 h.
Figure 4. The ratios (TER(D98%) and TER(Dmean)) and difference (ΔV45Gy) between the thermoradiotherapy treatment plans and corresponding radiation-only treatment plans of 15 patients, as a function of the time interval between radiation and hyperthermia treatment. Similar figures for the ΔV30Gy and D2cm3 (organs at risk) and the D2% and EUD (GTV) can be found in Supplementary Figure A1.
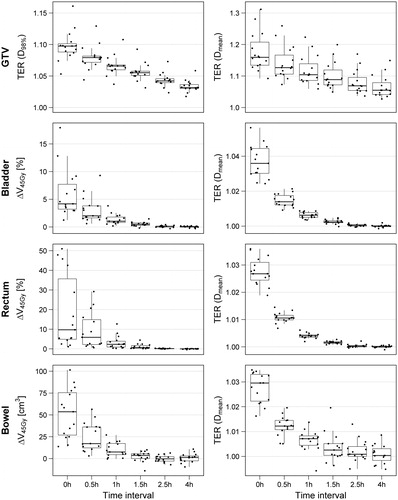
The therapeutic gain factor is mainly determined by the thermal enhancement ratio for the GTV, since the thermal enhancement ratio for all organs at risk is much closer to 1. Thus, the therapeutic gain factor shows a similar dependency on time interval as the therapeutic enhancement ratio for the GTV, and a time interval of 0 h yields the highest therapeutic gain (median TGF ≈ 1.12 for all three organs at risk, ).
Figure 5. Therapeutic gain factors for thermoradiotherapy compared to radiation alone of 15 patients, as a function of the time interval between radiation and hyperthermia treatment.
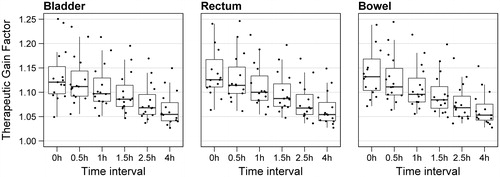
Finally, we verified that our analyses were not substantially affected by the uncertainty in radiobiological parameters (Supplementary Figure B1). In particular, we verified that the therapeutic gain remains highest at 0-h time interval for all plausible ranges of the radiobiological parameters.
Discussion
The results of this planning study show that the highest therapeutic gain can be obtained when the time interval between hyperthermia and radiotherapy is as short as possible. This may be counterintuitive, since the radiosensitizing effect of hyperthermia is largest for both the normal and tumor tissue when time intervals are very short. The anticipated higher normal tissue damage at shorter intervals, as predicted in preclinical studies [Citation16], may in the clinical setting be much more moderate because of lower temperatures in the organs at risk, the tumor selectiveness of direct cytotoxicity and a lower α/β ratio for late normal tissue complications.
A rough calculation shows how the combination of these three factors explain the difference between the predicted thermal enhancement ratio for the GTV on the one hand, and the thermal enhancement ratios for the organs at risk on the other hand. For a schedule of 23 × 2 Gy daily radiation treatment + weekly hyperthermia, the thermal enhancement ratio for the GTV (assuming a typical temperature of 41 °C and 0-h time interval) would be 1.177 (see Supplementary Appendix C). Subsequently lowering the temperature to 40 °C, omitting the direct cytotoxicity term from the equivalent radiation dose calculation and lowering the α37/β37 value to 3 Gy results in thermal enhancement ratios of 1.111, 1.089 and 1.024 respectively. The latter is comparable to the thermal enhancement ratios for the organs at risk.
The therapeutic gain factor varies considerably between patients. The variation in therapeutic gain factor is mainly caused by variation in thermal enhancement ratio for the GTV, since the thermal enhancement ratios for the organs at risk only vary by 1–2%. For a 0-h time interval, the thermal enhancement ratio for the GTV ranges from approximately 1.1–1.3. Calculation of thermal enhancement ratio for the GTV at 40 and 42 °C (the approximate range of T50) with a 0-h time interval shows that TERGTV(40 °C) = 1.111 and TERGTV(42 °C) = 1.277 (see Supplementary Appendix C). Thus, the variation in the thermal enhancement ratio for the GTV can be attributed to the variation in planned tumor temperatures. The large variation of the thermal enhancement ratio for the GTV with temperature stresses the importance of quality control, while the large variation between individuals shows that biological treatment planning may be a valuable tool in assessing the expected benefit of hyperthermia in individual patients.
The thermal enhancement ratio for the GTV predicted in our study is relatively low compared with values derived from clinical data. Assuming an increase in local control of approximately 2%/Gy [Citation32], the 20% increase in 3-year local control by hyperthermia reported in the Dutch Deep Hyperthermia Trial (DDHT) [Citation33] would correspond to a difference in equivalent radiation dose of 10 Gy, or a thermal enhancement ratio for the GTV of approximately 1.2. However, our planning study predicts that the thermal enhancement ratio for the GTV ranges from 1.05 to 1.1, for the 1–4 h time intervals between radiotherapy and hyperthermia employed in the Dutch Deep Hyperthermia Trial. A possible explanation for this difference is that the α41/α37 and β41/β37 values in this study were derived from cell survival assays. These assays can model the DNA repair suppressing capabilities of hyperthermia, but cannot model the increased perfusion and reoxygenation occurring during clinical hyperthermia – mechanisms that may be important for radiosensitization, particularly for (hypoxic) tumors. Thus, we expect the in vivo values of α41/α37 and β41/β37 for the tumor to be higher than what was derived in vitro. Using higher values of α41/α37 and β41/β37 for the GTV would result in a higher thermal enhancement ratio for the GTV, which would be more in line with the more favorable clinical results from the Dutch Deep Hyperthermia Trial. The therapeutic gain factor would also be higher when reoxygenation effects are included as the thermal enhancement ratio in the nonhypoxic normal tissues would not be altered. Incorporating perfusion and reoxygenation mechanisms in biological modelling requires the derivation of radiobiological parameters from in vivo experiments and is subject of further research [Citation34].
In addition to the changes in the ratios α41/α37 and β41/β37, modeling a hypoxic tumor would also require a lower value of α37. Rewriting EquationEquation (4)(4) , it can be shown that only the term accounting for direct cytotoxicity depends on α37, the other terms only depend on the ratios α37/β37, α41/α37 and β41/β37:
(5)
Direct toxicity, however, does not depend on the time interval. Thus, changing the value of α37 does not substantially affect the optimal time interval. Despite the uncertainty in the various biological parameters, our conclusion regarding the optimal time interval seems robust, since the therapeutic gain remains highest at 0 h time interval for all plausible ranges of the radiobiological parameters (Supplementary Appendix B).
LQ-parameters valid under hyperthermic conditions are very important for biological modelling and the present study is the first to include very detailed data for tumor tissue from cell survival assays describing radiosensitization as a function of temperature and time interval [Citation18]. For normal tissue, parameters were based on more limited data from animal experiments, which only varied one variable (either temperature or time interval) at a time [Citation16]. A future in vivo study in which EQDRT is measured at different combinations of radiation dose, temperature and time interval (similar to the in vitro data in [Citation18]) for both tumor and normal tissue is essential to provide LQ-parameters for tumor tissue in which not only DNA repair suppression, but also other mechanisms of hyperthermia (such as increased perfusion and reoxygenation) are accounted for, potentially making these parameters more representative for the clinical situation. Additionally, such a study would allow LQ-parameters for normal tissues to be more formally derived through a regression analysis.
A recent, retrospective clinical study showed that a shorter time interval between radiotherapy and hyperthermia (1 h compared to 1.5 h) resulted in lower in-field recurrence rates in advanced stage cervical cancer patients [Citation17]. A significant difference (37%) was observed, but the uncertainty in this difference was large due to the small size of the study cohort. No evidence was found for any difference in toxicity, although the low number of toxicities limited statistical power. The current planning study corroborates these results and suggests that an even stronger therapeutic benefit for thermoradiotherapy treatments may be obtained for very short (<30 min) time intervals. Such a strategy may work, as another study showed promising results in the treatment of large, preirradiated breast cancer recurrences with hyperthermia immediately prior to the RT fractions [Citation35]. Despite the low dose of 5 × 4 Gy, a CR of 61% was achieved in 73 patients with macroscopic disease, while only grade 1 toxicities were found. Thus, response rates are comparable to results obtained with conventional treatment schemes that apply higher doses (8 × 4 Gy/12 × 3 Gy) with longer time intervals [Citation36,Citation37]. While an exact number cannot be estimated, the combined results of these studies [Citation17,Citation35–37] suggest that a substantial increase in tumor control may be expected when very short time intervals are used between radiotherapy and hyperthermia in the treatment of advanced stage cervical cancer. In vivo experiments in which hyperthermia immediately precedes radiotherapy may confirm whether such a strategy could also be safe and effective for cervical cancer. As our study showed that the temperature and the α/β value are determining factors for the efficacy of such a treatment, treatment schedules with clinically realistic fraction sizes (typically 2 Gy) and temperatures (i.e. 40–42 °C for tumors, and 39–41 °C for organs at risk) should be used in these in vivo experiments. If such a schedule is proven to be safe, this may provide an opportunity to further improve the clinical outcome of advanced stage cervical cancer patients treated with thermoradiotherapy.
Conclusion
This planning study indicates that the largest therapeutic gain for thermoradiotherapy in cervical cancer patients can be obtained when hyperthermia is delivered immediately before or after radiotherapy. To further support these results future research should focus on hyperthermic enhancement of radiosensitivity in normal tissue and include other relevant mechanisms of hyperthermia in biological modelling, such as reoxygenation.
IHYT_1468930Supplemental_File.pdf
Download PDF (496.5 KB)Disclosure statement
No potential conflict of interest was reported by the authors.
Additional information
Funding
References
- Ferlay J, Soerjomataram I, Dikshit R, et al. Cancer incidence and mortality worldwide: Sources, methods and major patterns in GLOBOCAN 2012. Int J Cancer. 2015;136:E359–E386.
- Lutgens L, van der Zee J, Pijls-Johannesma M, et al. Combined use of hyperthermia and radiation therapy for treating locally advanced cervical carcinoma. Cochrane Database Syst Rev. 2010;CD006377.
- Lutgens LCHW, Koper PCM, Jobsen JJ, et al. Radiation therapy combined with hyperthermia versus cisplatin for locally advanced cervical cancer: Results of the randomized RADCHOC trial. Radiother Oncol. 2016;120:378–382.
- Datta NR, Rogers S, Klingbiel D, et al. Hyperthermia and radiotherapy with or without chemotherapy in locally advanced cervical cancer: a systematic review with conventional and network meta-analyses. Int J Hyperthermia. 2016;32:809–821.
- Eifel PJ, Winter K, Morris M, et al. Pelvic irradiation with concurrent chemotherapy versus pelvic and para-aortic irradiation for high-risk cervical cancer: an update of radiation therapy oncology group trial (RTOG) 90-01. J Clin Oncol. 2004;22:872–880.
- Kampinga HH, Dikomey E. Hyperthermic radiosensitization: mode of action and clinical relevance. Int J Radiat Biol. 2001;77:399–408.
- Oei AL, Vriend LEM, Crezee J, et al. Effects of hyperthermia on DNA repair pathways: one treatment to inhibit them all. Radiat Oncol. 2015;10:165.
- Winslow TB, Eranki A, Ullas S, et al. A pilot study of the effects of mild systemic heating on human head and neck tumour xenografts: analysis of tumour perfusion, interstitial fluid pressure, hypoxia and efficacy of radiation therapy. Int J Hyperthermia. 2015;31:693–701.
- Vujaskovic Z, Song CW. Physiological mechanisms underlying heat-induced radiosensitization. Int J Hyperthermia. 2004;20:163–174.
- Sun X, Xing L, Ling CC, et al. The effect of mild temperature hyperthermia on tumour hypoxia and blood perfusion: relevance for radiotherapy, vascular targeting and imaging. Int J Hyperthermia. 2010;26:224–231.
- Brizel DM, Scully SP, Harrelson JM, et al. Radiation therapy and hyperthermia improve the oxygenation of human soft tissue sarcomas. Cancer Res. 1996;56:5347–5350.
- Horsman MR, Overgaard J. Hyperthermia: a potent enhancer of radiotherapy. Clin Oncol. 2007;19:418–426.
- Overgaard J. The heat is (still) on-the past and future of hyperthermic radiation oncology. Radiother Oncol. 2013;109:185–187.
- Dewey WC, Hopwood LE, Sapareto SA, et al. Cellular responses to combinations of hyperthermia and radiation. Radiology. 1977;123:463–474.
- Streffer C. Molecular and cellular mechanisms of hyperthermia. In: Seegenschmiedt MH, Fessenden P, Vernon CC, editors. Thermoradiotherapy thermochemistry. Berlin: Springer-Verlag; 1995. p. 47–74.
- Overgaard J. Simultaneous and sequential hyperthermia and radiation treatment of an experimental tumor and its surrounding normal tissue in vivo. Int J Radiat Oncol Biol Phys. 1980;6:1507–1517.
- van Leeuwen CM, Oei AL, Chin KWTK, et al. A short time interval between radiotherapy and hyperthermia reduces in-field recurrence and mortality in women with advanced cervical cancer. Radiat Oncol. 2017;12:75.
- van Leeuwen CM, Oei AL, Ten Cate R, et al. Measurement and analysis of the impact of time-interval, temperature and radiation dose on tumour cell survival and its application in thermoradiotherapy plan evaluation. Int J Hyperthermia. 2017;34:30–38.
- Sreenivasa G, Gellermann J, Rau B, et al. Clinical use of the hyperthermia treatment planning system HyperPlan to predict effectiveness and toxicity. Int J Radiat Oncol Biol Phys. 2003;55:407–419.
- van Haaren PMA, Hulshof MCCM, Kok HP, et al. Relation between body size and temperatures during locoregional hyperthermia of oesophageal cancer patients. Int J Hyperthermia. 2008;24:663–674.
- Franken NAP, Oei AL, Kok HP, et al. Cell survival and radiosensitisation: modulation of the linear and quadratic parameters of the LQ model (Review). Int J Oncol. 2013;42:1501–1515.
- Myerson RJ, Roti Roti JL, Moros EG, et al. Modelling heat-induced radiosensitization: clinical implications. Int J Hyperth. 2004;20:201–212.
- Kok HP, Crezee J, Franken NAP, et al. Quantifying the combined effect of radiation therapy and hyperthermia in terms of equivalent dose distributions. Int J Radiat Oncol Biol Phys. 2014;88:739–745.
- van Leeuwen CM, Crezee J, Oei AL, et al. 3D radiobiological evaluation of combined radiotherapy and hyperthermia treatments. Int J Hyperth. 2017;33:160–169.
- Crezee J, van Leeuwen CM, Oei AL, et al. Biological modelling of the radiation dose escalation effect of regional hyperthermia in cervical cancer. Radiat Oncol. 2016;11:14.
- van Dijk JD, Schneider C, van Os R, et al. Results of deep body hyperthermia with large waveguide radiators. Adv Exp Med Biol. 1990;267:315–319.
- Kok HP, Kotte ANTJ, Crezee J. Planning, optimisation and evaluation of hyperthermia treatments. Int J Hyperthermia. 2017;33:593–607.
- Heijkoop ST, Westerveld H, Bijker N, et al. Optimal patient positioning (prone versus supine) for VMAT in gynecologic cancer: a dosimetric study on the effect of different margins. Int J Radiat Oncol Biol Phys. 2016;96:432–439.
- Bentzen SM, Joiner MC. The linear-quadratic approach in clinical practice. In: Joiner MC, van der Kogel A, editors. Basic clinical radiobiology. 4th ed. London: Hodder Arnold; 2009. p. 120–134.
- Niemierko A. Reporting and analyzing dose distributions: a concept of equivalent uniform dose. Med Phys. 1997;24:103–110.
- Bruggmoser G, Bauchowitz S, Canters R, et al. Guideline for the clinical application, documentation and analysis of clinical studies for regional deep hyperthermia: quality management in regional deep hyperthermia. Strahlenther Onkol. 2012;188: 198–211.
- Okunieff P, Morgan D, Niemierko A, et al. Radiation dose-response of human tumors. Int J Radiat Oncol. 1995;32:1227–1237.
- van der Zee J, González D, van Rhoon GC, et al. Comparison of radiotherapy alone with radiotherapy plus hyperthermia in locally advanced pelvic tumours: a prospective, randomised, multicentre trial. Lancet. 2000;355:1119–1125.
- Crezee H, van Leeuwen CM, Oei AL, et al. Thermoradiotherapy planning: integration in routine clinical practice. Int J Hyperth. 2016;32:41–49.
- Notter M, Piazena H, Vaupel P. Hypofractionated re-irradiation of large-sized recurrent breast cancer with thermography-controlled, contact-free water-filtered infra-red-A hyperthermia: a retrospective study of 73 patients. Int J Hyperthermia. 2016;33:227–236.
- Oldenborg S, Griesdoorn V, van Os R, et al. Reirradiation and hyperthermia for irresectable locoregional recurrent breast cancer in previously irradiated area: size matters. Radiother Oncol. 2015;117:223–228.
- Linthorst M, Baaijens M, Wiggenraad R, et al. Local control rate after the combination of re-irradiation and hyperthermia for irresectable recurrent breast cancer: results in 248 patients. Radiother Oncol. 2015;117:217–222.