Abstract
Cellular metabolic reprogramming is an important feature of malignant tumors. Metabolic reprogramming causes changes in the levels or types of specific metabolites inside and outside the cell, which affects tumorigenesis and progression by influencing gene expression, the cellular state, and the tumor microenvironment. During tumorigenesis, a series of changes in the glucose metabolism, fatty acid metabolism, amino acid metabolism, and cholesterol metabolism of tumor cells occur, which are involved in the process of cellular carcinogenesis and constitute part of the underlying mechanisms of tumor formation. Hyperthermia, as one of the main therapeutic tools for malignant tumors, has obvious effects on tumor cell metabolism. In this paper, we will combine the latest research progress in the field of cellular metabolic reprogramming and focus on the current experimental research and clinical treatment of hyperthermia in cellular metabolic reprogramming to discuss the feasibility of cellular metabolic reprogramming-related mechanisms guiding hyperthermia in malignant tumor treatment, so as to provide more ideas for hyperthermia to treat malignant tumors through the direction of cellular metabolic reprogramming.
1. Introduction
Hyperthermia (HT) refers to a treatment by applying different physical factors (radiofrequency, microwave, ultrasound, laser, etc.) to raise the temperature of tumor tissues and/or the whole body, using high temperature and its secondary effects to treat tumors. Currently, hyperthermia has become the fifth major therapy in tumor treatment after surgery, radiotherapy, chemotherapy and immunotherapy, and is a new and effective means, which is called ‘green therapy for tumors’ [Citation1]. Hyperthermia takes advantage of the characteristic that tumor cells are less tolerant to high temperature than normal cells, increasing the temperature inside the tumor to 39–45 °C to induce apoptosis and inhibit cellular DNA repair; promoting the delivery and dispersion of chemotherapeutic drugs in the tumor to enhance the effect of chemotherapy; improving blood flow to rectify hypoxia, increasing oxygen free radicals and sensitizing radiotherapy [Citation2]. Hyperthermia can destroy and kill cancer cells with minimal damage to normal tissues, which is an effective, feasible, and safe physical therapy [Citation3]. Depending on the scope of application, hyperthermia can be divided into local hyperthermia and whole body hyperthermia (WBH) [Citation4]. In clinical practice, local hyperthermia raises the intra-tumor temperature to 39–45 °C or higher by external energy, including ultrasound (US), radiofrequency, and microwaves [Citation5]. WBH is a treatment method that uses heat and its secondary effects to selectively kill cancer and control the widespread metastasis of cancer cells by artificially raising the body temperature, which is characterized by raising not only the temperature at the cancer site but also the whole body temperature to the same temperature. Within a certain temperature range (<42 °C), WBH can improve the immunity of the body and inhibit the metastasis of tumor [Citation6]. WBH is usually achieved by increasing the ambient temperature, which has a lower temperature (39.5–40.5 °C) and a longer duration (1–2 h) compared to local hyperthermia [Citation7].
Metabolic reprogramming is one of the ten characteristics of tumor cells and has been a hot research area in recent years [Citation8]. To fulfill the bioenergetic and biosynthetic requirements for cell growth, proliferation, immune escape and metastasis, as well as to adapt to the tumor microenvironment, cancer cells undergo altered energy metabolic patterns [Citation9]. The metabolic reprogramming of glucose, cholesterol, fatty acids and amino acids in tumor cells is involved in all stages of tumor development, and the differences between them and normal cells reveal the underlying mechanisms of tumor formation [Citation10, Citation11]. As early as the 1980s and 1990s, there have been initial studies on the relationship between hyperthermia and metabolic changes in tumor cells [Citation12, Citation13], but most of the past studies were limited to the changes in the quality of cellular metabolites before and after the effects of hyperthermia, and there were few studies on the mechanisms of metabolic changes induced by hyperthermia. With the refinement of the theory of cellular metabolic reprogramming and the promotion of hyperthermia in clinical applications, we believe that hyperthermia and cellular metabolism seem to have more possibilities.
2. Metabolic reprogramming and tumor progression
In tumor progression, a series of metabolic or structural changes occur within the tumor cells to meet their ‘tumorization’ process. The difference between tumor cells and normal cells means that the original metabolic approach can no longer meet their specific growth requirements and tumor cells must make adaptive metabolic changes. Metabolic reprogramming is one of the most remarkable features of tumors [Citation14] and plays a huge role in tumor cell progression [Citation15] ().
Figure 1. The most notable metabolic modalities of tumor cells include mainly glucose metabolic reprogramming, lipid metabolic reprogramming and amino acid metabolic reprogramming. They play an important role in tumor progression and cover a wide range of aspects including tumor proliferation, distant metastasis, death resistance, immune evasion and treatment tolerance.
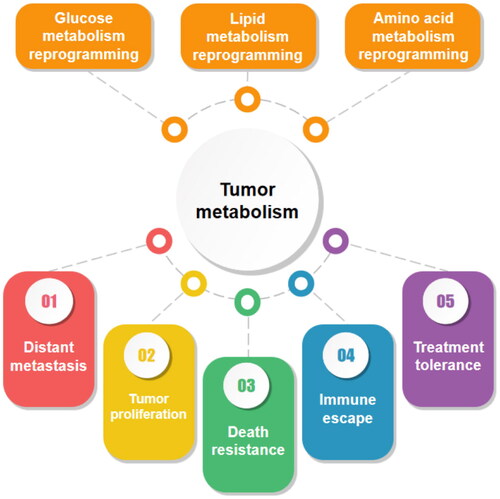
Unlike normal cells, tumor cells still prefer to undergo glycolysis even in the presence of oxygen, a phenomenon known as the Warburg effect [Citation16]. The discovery of the Warburg effect has opened new doors for the study of tumor metabolism. It was found that aerobic glycolysis could not only meet the normal energy requirements of tumors, but also promote tumor growth by affecting other metabolic modalities [Citation17]. The treatment resistance [Citation18], metastasis promotion [Citation19], and immune evasion [Citation20] induced by aerobic glycolysis are crucial for tumor development.
In addition to glucose, lipid metabolic reprogramming is extremely important in tumor progression. Lipids are widely distributed in various membrane structures and are key components of all membranes [Citation21]. In addition to their role as structural components, lipids can also act as second messengers to transmit signals within the cell and are an important source of energy when nutrients are limited [Citation22]. There is growing evidence that lipid metabolism is substantially reorganized in cancer. Upregulation of lipid uptake [Citation23], increased storage [Citation24], and increased lipid synthesis [Citation25] have been demonstrated in a variety of cancers. The metabolic changes in a range of lipids such as fatty acids, cholesterol, triglycerides, and sphingolipids create favorable conditions for adaptive tumor growth.
Amino acids are carbon and nitrogen sources for biosynthetic processes and are widely involved in the synthesis of macromolecules such as proteins, nucleic acids, and lipids [Citation26]. Abnormal alterations in amino acid metabolism are also closely related to tumor development and tumor immunity [Citation27]. Through the energy supply by glutamine oxidation[Citation28], the maintenance of redox balance by serine/glycine [Citation29], and the regulatory role of proline in cellular autophagy and apoptosis [Citation30], these metabolic properties have important roles in tumor cell proliferation, migration, invasion, and immunity [Citation31, Citation32]. To meet the survival and proliferation needs of tumor cells, the metabolism of several amino acids is in a dysregulated state. Abnormal accumulation of amino acids and imbalance in the expression of metabolism-related enzymes provide additional evidence for tumor growth regulation mechanisms.
Over the years, as further research on tumor metabolism has been conducted, there is no doubt that there is a close relationship between metabolic reprogramming of tumors and tumor progression, although there are more explanations and controversies about it. This has greatly helped us to understand the factors of tumor progression and provided more ideas for the exploration of tumor therapeutic tools.
3. Antitumor therapeutic effect of metabolic reprogramming
In addition to providing energy, structural substances, and signaling molecules to meet the growth needs of tumor cells, metabolic reprogramming also plays an important role in antitumor therapy [Citation33] ().
Figure 2. Thermotherapy affects other therapeutic modalities such as chemotherapy, radiotherapy, immunotherapy, sonodynamic therapy and photodynamic therapy by influencing the critical metabolic modalities or metabolites involved in the reprogramming of glucose, lipid and amino acid metabolism.
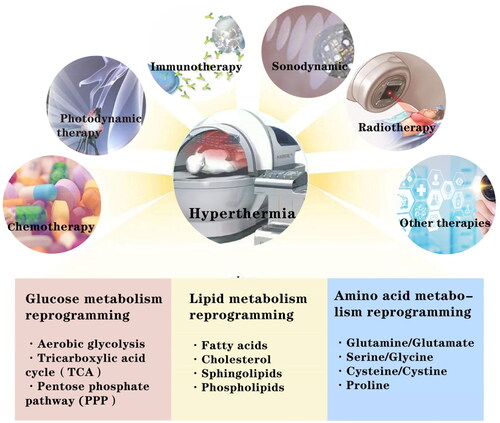
Metabolic reprogramming can interfere with antitumor immunity [Citation34, Citation35]. Aerobic glycolysis is a key means for tumor cells to evade immune surveillance. On one hand, tumor cells can interfere with CD8+ T cell activation and infiltration into tumor tissue by promoting programmed cell death 1 ligand 1 (PD-L1) expression [Citation36], and on the other hand, extracellular acidification induced by glycolysis can also protect tumor cells from macrophage-mediated phagocytosis [Citation37]. Moreover, it is not only the metabolic reprogramming of tumor cells that plays a role. In the tumor microenvironment, responsive T cells exhibit disordered lipid metabolism accompanied by senescence induction [Citation38], while inhibition of natural killer cell (NK cell) glucose metabolism due to lipid peroxidation also plays a key role in antitumor immunity [Citation39]. Metabolic changes in tumor cells and immune cells together shape the appropriate immune microenvironment for tumor growth.
Metabolic reprogramming can induce tumor therapy resistance [Citation40]. Glycolysis [Citation41, Citation42], lipid metabolism [Citation43, Citation44], amino acids [Citation45] and mitochondrial [Citation46] metabolic reprogramming are closely associated with the development of tumor treatment tolerance. By providing the necessary support for energy supply, biosynthesis and maintenance of redox state homeostasis, the more complex growth requirements of tumor cells under therapeutic conditions are met. Despite the different metabolic reprogramming changes in different tumor cells, they play similar roles in antitumor therapy. The development of tumor drug resistance is a major challenge that hinders the effectiveness of chemotherapy. Metabolic reprogramming-induced activation of damage repair, enhancement of autophagy, and suppression of the immune microenvironment play a considerable role in promoting the development of drug resistance [Citation47]. It has been shown that ceramide kinase-mediated reprogramming of sphingolipid metabolism is involved in the development of tamoxifen resistance in estrogen receptor-positive breast cancer patients [Citation48], while the enhanced glycolytic process is a key factor in flavopiridol (FP) resistance in prostate cancer [Citation49], and targeting cellular metabolism is an effective means of improving tumor chemotherapy sensitivity. In addition, the development of radiotherapy resistance is accompanied by metabolic reprogramming. High glucose flux-induced cardiolipin accumulation inhibits cytochrome C-mediated apoptosis [Citation50], and serine/glycine metabolism maintains redox homeostasis in the tumor microenvironment [Citation51], and these metabolic modalities have a significant attenuating effect on radiation damage.
Therefore, the search for therapeutics that can target metabolic reprogramming is of great importance.
4. The effect of hyperthermia on cellular metabolic reprogramming
4.1. The influence of hyperthermia on glucose metabolism
The glucose metabolism of tumor cells can be divided into three ways: (1) aerobically glycolytic (the Warburg effect characterizing proliferating cancer cells), (2) anaerobically glycolytic because of hypoxia, and (3) Oxidative Phosphorylation [Citation52, Citation53]. These three modes of glucose metabolism do not exist in isolation, but work together in tumor cells. However, the metabolic mode that play a key role are not the same in different types of tumor cells. The complexity of glucose metabolic reprogramming in tumor cells means that a single theory cannot reasonably adequately explain the mechanism of hyperthermia’s effect on glucose metabolism. As a type of pyruvate kinase (PK), PKM is the rate-limiting enzyme in glycolysis and catalyzes the generation of pyruvate from phosphoenolated pyruvate [Citation54]. It was reported that nude mice bearing orthotopic MCF-7 tumors were treated with photothermal therapy combined with a guanidine-rich, spherical helical polypeptide to elevate the local tumor temperature, and when the temperature increased to 39.6 °C for 5 min, PKM2 began to show a decreasing trend and decreased significantly with the increase in temperature. It indicates that under the influence of high temperature, the glycolytic rate-limiting enzyme PKM can be degraded, and energy produced by tumor glycolysis is reduced [Citation55]. Hyperthermia significantly alters multiple components of glycolysis and mitochondrial function in the vast majority of tumor cell lines [Citation56]. Studies have shown that hyperthermia at 43 °C for 1h can increase the level of plasma lactate in mice and also directly damage mitochondria or activate hypoxia-inducible factor-1 (HIF-1) and its downstream targets, vascular endothelial growth factor (VEGF) and pyruvate dehydrogenase kinase 1 (PDK1) in tumors, resulting in decreased mitochondrial function and reduced cellular oxygen consumption rate [Citation57]. And PDK1 can inactivate pyruvate dehydrogenase (PDH) to inhibit the tricarboxylic acid cycle, which is accompanied by an increase in the level of the lactate cycle and promotes the switch of heated tumors to anaerobic metabolism [Citation58]. Hyperthermia inactivates glycolytic enzymes and reduces the rate of energy metabolism, so that it is no longer able to meet the various specific demands of tumor cell development. Tumor development is highly dependent on glucose supply and is mostly accompanied by high expression of glucose transporters (GLUT) [Citation59]. It has been shown that the level of GLUT-1 in isolated bovine aortic endothelial cells is reduced under the condition of hyperthermia at 42 °C for 2h, resulting in impaired uptake of glucose [Citation60]. It is important to note that the therapeutic effect of hyperthermia is related to time and temperature. Sublethal heat stress can trigger a stronger Warburg effect in tumor cells, which not only fails to have a therapeutic effect, but also contributes to the development of heat resistance in cancer cells as well as tumor recurrence and invasion [Citation61].
Similarly, reducing tumor heat resistance by modulating glycolysis is one of the effective ways to improve the efficacy of hyperthermia. Tumor cells aberrantly overexpress heat shock proteins (HSPs) to resist heat damage, leading to insufficient apoptosis and tumor recurrence [Citation62]. Pyruvate kinase M2 (PKM2), an isoform of PKM, is highly expressed in a variety of tumors. In addition to its role as the pyruvate kinase in glycolysis in tetrameric form, PKM2 is also capable of translocating from the cytoplasm to the nucleus and regulating gene expression as the dimeric protein kinase in cooperation with various transcription factors, playing a key role in the proliferation, invasion and metastasis of tumor cells [Citation63]. For example, PKM2 can promote tumor angiogenesis by regulating HIF-1α through NF-κB activation [Citation64]; PKM2 can directly bind to histone H3 and phosphorylate histone H3 upon EGF receptor activation, which is instrumental in EGF-induced expression of cyclin D1 and c-Myc, tumor cell proliferation, cell-cycle progression, and tumorigenesis [Citation65]; Recently, it has been reported that ectosomal PKM2 accelerates the differentiation of monocytes into macrophages through SUMO modification, which leads to the release of cytokines and chemokines and promotes tumor process [Citation66]. The mechanism by which PKM2 promotes tumor progression is still being explored, but there is no doubt that PKM2 can be used as a target for tumor therapy. In addition, silencing PKM2 reduces energy supply and thus downregulates the expression of HSP, which is able to overcome the heat resistance of tumor cells [Citation55]. Glucose oxidase (GOx) also has a similar effect by mediating tumor starvation to inhibit ATP production to achieve down-regulation of HSP expression and reduce cellular heat resistance [Citation67]. In recent years, the rapid development of glycolysis-inhibiting targeted drugs and nanomaterials [Citation55, Citation68, Citation69] has generated new ideas for the application of hyperthermia in tumor therapy, and a large number of research results have demonstrated the promising potential of combining hyperthermia with glucose metabolism reprogramming.
4.2. The influence of hyperthermia on lipid metabolism
Unlike the function of glucose as a simple energy supplier, the role of lipids in cell growth is more complex, and the effect of hyperthermia on the reprogramming of lipid metabolism is evident at all levels. In the past, it has been suggested that hyperthermia generates reactive oxygen radicals in the cell membrane or cytoplasm of cancer cells, leading to intracellular polyunsaturated fatty acid (PUFA) peroxidation as an important source of antitumor activity [Citation70]. As research progresses, the interaction of ferroptosis, reactive oxygen species and lipid peroxidation, as well as changes in lipid metabolism-related enzymes in hyperthermia, provide a more complete complement to the anti-tumor mechanism of hyperthermia. Not long ago, Ma’s research team discovered and confirmed the role and molecular mechanism that local hyperthermia at 41 °C for 2h can activate beige fat thermogenesis through heat shock factor 1 (HSF1)- Hnrnpa2b1 (A2b1) transcriptional axis to reduce obesity and improve metabolic disorders [Citation71]. This provided new ideas for the clinical application of hyperthermia to regulate lipid metabolism and also provided a stronger theoretical support for the ability of hyperthermia to regulate lipid metabolism.
Fatty acid conversion, synthesis, uptake, and oxidative metabolism-related proteins and enzymes are the most common targets of hyperthermia to affect the reprogramming of lipid metabolism. As a vehicle for cellular lipid transport, apolipoproteins are capable of targeted transport of lipids such as cholesterol and triglycerides, and changes in their function and quantity directly affect cell viability [Citation72]. Hyperthermia has been shown to significantly increase very low density lipoprotein (VLDL) synthesis and downregulate high density lipoprotein (HDL) synthesis. The synthesis of VLDL was increased more than fourfold in Walker 256 tumor-bearing rats exposed to hyperthermia stimulation compared to controls, and the increased survival of tumor-bearing rats after a single session of hyperthermia was associated with the increase in VLDL and the decrease in HDL [Citation73]. The degree of phosphorylation of acetyl CoA carboxylase (ACC), a key enzyme for fatty acid synthesis, is highly correlated with tumor progression and metastasis, and elevated ACC phosphorylation can increase the risk of tumor metastasis [Citation74]. It was shown that the degree of ACC phosphorylation (P-ACC) decreases during hyperthermia at 43 °C for 10 min, and the catalytic product malonyl CoA increases, which manifests in an inhibitory effect on carnitine palmitoyl transferase 1 A (CPT1A), a defect in fatty acid oxidation (FAO), and an increase in apoptosis. Conversely, the therapeutic effect of hyperthermia can be significantly improved by altering the action of metabolic enzymes. The classical inhibitors of FAO, etomoxir and ranolazine, can increase the heat sensitivity of tumor cells by competitively inhibiting CPT1A, which in turn improves the killing effect of heat-stressed cells [Citation75]. The relationship between hyperthermia and lipid metabolism is complex and promising to be studied, but currently few relevant experiments have been conducted. Confirming the effect of hyperthermia on lipid metabolism-related enzymes is the most direct breakthrough, and verifying the role of specific lipid types in it is also one of the feasible research directions.
4.3. The influence of hyperthermia on amino acid metabolism
Although studies related to the effect of hyperthermia on amino acid metabolism have been performed long ago, most of them were limited to the exploration of phenotypic validation and were relatively superficial. Hyperthermia at 44 °C for 33 min promotes the activation or relocation of existing amino acid transport proteins and a significant increase in amino acid uptake [Citation76], and the high glutamine concentration in tumor cells promotes high expression of HSPs, which have an integral role in the development of heat resistance in tumor cells [Citation77]. Glutathione is able to affect tumor cells through multiple death pathways, including necrosis, apoptosis, autophagy, and ferroptosis [Citation78]. An ex vivo experimental study showed that GSH levels in Hela cells exhibited a significant increase after 40 °C treatment for 1h, and the increased expression of Prx2, a key transcription factor for oxidative stress, played a key role in this process [Citation79]. Currently, most of the research on the role of amino acids in hyperthermia has focused on amino acid polymer-related nanocarriers [Citation80, Citation81], which have good performance in tumor-targeted drug delivery systems by utilizing the good biocompatibility and heat sensitivity of amino acid polymers. Moreover, the use of amino acids or amino acid metabolism-related substances as pointers to guide tumor photothermal precision therapy has become a new possibility [Citation82]. Even so, there is still a large gap in the study of hyperthermia combined with amino acid metabolism in tumor treatment, and in view of the complexity of amino acid species, the interactions between different types of amino acids, and the high difficulty of detecting related indicators, the study of hyperthermia and amino acid metabolism has great limitations. The development of new application tools is the key to the application of amino acid metabolism to hyperthermia and is the most feasible research direction in the future.
4.4. The influence of hyperthermia on mitochondrial function
Mitochondria are the site of final oxidation of sugars, lipids and amino acids to release energy and are the center of intracellular metabolism of various energy substances [Citation83], providing 80% of the energy required for cell growth [Citation84]. A common view is that mitochondrial dysfunction is a hallmark of cancer development [Citation85], and most of the cancer progression is accompanied by mitochondrial dysfunction. On the other hand, the finding of the Warburg effect seems to prove that mitochondria are irrelevant for rapid tumor cell proliferation, but in fact, mitochondrial biogenesis and quality control are usually upregulated in cancer [Citation86]. The results of the hyperthermia efficacy and mitochondrial function assays suggest that hyperthermia kills tumor cells by causing mitochondrial dysfunction.
Mitochondria, as the major consumers of oxygen, consume approximately 98% of the total body oxygen [Citation87]. Mitochondria are important sites for oxidative phosphorylation of glucose in tumor cells. When mitochondrial dysfunction occurs, oxidative phosphorylation (OXPHOS) capacity is altered [Citation88]. In isolated mitochondrial heat treatment experiments, oxidative phosphorylation of isolated mitochondria was progressively impaired by hyperthermic perfusion at 42–52 °C. Significant inhibition of respiratory chain components was observed after 42 °C perfusion, and irreversible uncoupling was evident after perfusion at 46 °C and above. After perfusion at 50–52 °C, the energy supply function of mitochondria was completely impaired and mitochondria became energy consumers [Citation89]. Irreversible disruption of mitochondrial function leads to significant cell death.
Mitochondrial membrane potential is directly related to mitochondrial function, and a decrease in mitochondrial membrane potential is considered an early marker of apoptosis [Citation90].
It was found that mitochondrial membrane potential was downregulated and oxygen consumption rate (OCR) was reduced by approximately 50% in cancer cells treated with HT (46 °C water bath for 5 min) compared to untreated pancreatic cancer cells (p < 0.001) [Citation91]. In addition, hyperthermia (43 °C, 3h) is able to depolarize the mitochondrial membrane potential and release cytochrome C into the cytoplasm [Citation92], inducing the activation of the mitochondrial apoptotic pathway, and mitochondrial dysfunction plays a key role in the cell death process.
4.5. The influence of hyperthermia on metabolism inducing cell death
Currently, hyperthermia has been more intensively studied in inhibiting tumor cell proliferation [Citation93] as well as in promoting apoptosis [Citation94]. With the depth of research, the relationship between hyperthermia and apoptosis as well as necrosis is well understood; however, the role of cellular metabolism in it deserves to be explored further. The links between metabolism, hyperthermia, and cell death are further elaborated below.
4.5.1. The disruption of membrane structure and function
Hyperthermia usually leads directly to cell necrosis, which is related to the damage of the cell membrane and cytoskeleton by heat [Citation95]. Cell membranes are in liquid crystal phase at room temperature, and hyperthermia at 42 °C for 1 h can activate acidic sphingomyelinase and generate large amounts of ceramide (Cer) [Citation96], while elevated Cer levels can displace Chol from membrane and form large, Cer-enriched membrane platforms ‘Cer-rafts’ [Citation97]. This transformation between lipids destabilizes the tumor cell membrane structure, loses its original function, and is much less tolerant to damage from inside and outside the cell. In addition, changes in the fatty acid and cholesterol content of the cell membrane alter the fluidity and permeability of the cell membrane [Citation98], leading to changes in the intracellular environment and impeding the function of transmembrane transport proteins and cell surface receptors. Tumor cell membranes have a higher fatty acid content than normal cells [Citation99], and the cell membranes are more fluid and more susceptible to heat. On the one hand, hyperthermia can also activate phospholipase [Citation100], which reduces lipids containing polyunsaturated fatty acids (PUFA) in cell membranes. On the other hand, the increase in membrane fluidity induced by hyperthermia favors the formation of type 2 purinergic receptor 7 (P2X7) pores and promotes cell death [Citation101]. The interaction between hyperthermia and tumor cell membrane properties results in one greater than the sum of its parts. And there is great scope for future research in hyperthermia programmes targeting cell membranes.
In addition, lipid rafts are functional cholesterol-rich micro-regions in cell membranes that play an important role in signal transduction and substance transport [Citation102]. It was found that hyperthermia (40, 41 or 42 °C for 1h) can interfere with the integrity of lipid rafts by affecting the reorganization of membrane cholesterol [Citation103], causing them to lose their normal function and subsequently be cleared.
4.5.2. ROS-mediated cell death
As a by-product of the metabolic process, heat can induce a sharp increase in reactive oxygen species (ROS) [Citation104], while the excessive accumulation of ROS will lead to the disruption of intracellular redox homeostasis and occurring oxidative stress [Citation105]. On the one hand, under the effect of hyperthermia, a large accumulation of intracellular ROS, heat and ROS together denature proteins, causing cells to undergo unfolded protein reactions, and denatured proteins cannot be degraded in a timely and effective manner, resulting in cell death [Citation106]. On the other hand, enhanced mitochondrial reactive oxygen species production during heat stress leads to nonspecific modifications of lipids, proteins, and nucleic acids, resulting in bioenergetic dysfunction [Citation106]. Moreover, as an autophagy activating factor [Citation107], ROS can mediate the phagocytosis and killing of phagocytes to promote cell death [Citation108]. In addition to this, ROS-mediated mitochondrial apoptosis [Citation109] and ferroptosis [Citation110] under the effect of hyperthermia have also been demonstrated.
In addition to the above pathways, hyperthermia can affect tumor cell death through other pathways. A study by Prescott et al. using radiotherapy combined with hyperthermia to treat canines with soft tissue sarcomas demonstrated that hyperthermia could affect adenosine triphosphate (ATP)/phosphomonoester (PME). ATP/PME decreased with increasing Cum min T ≥ 41.5 °C (cumulative minutes at ≥41.5 °C in two hyperthermia treatments) in the range of 100–500 min, and the results demonstrated that the reduction in ATP/PME predicted for a greater degree of tumor necrosis [Citation111]. The way in which hyperthermia induces cell death has been progressively documented theoretically. However, it has been demonstrated that moderate hyperthermia heating during thermal ablation increases the activity of tumor cells and fully activates them, thus secreting pro-tumorigenic factors and inducing proliferation [Citation112], so that incomplete thermal ablation of tumors promotes tumorigenesis [Citation113]. The dual nature of the thermal ablation effect should be fully considered in future studies ().
Table 1. Changes in each metabolite or metabolic process under the influence of hyperthermia alone.
5. Hyperthermia influences other therapies by modulating tumor metabolism
5.1. Hyperthermia combined with chemotherapy
The therapeutic effect of hyperthermia combined with chemotherapy has been confirmed by a large number of clinical cases [Citation114, Citation115], especially the mature application of peritoneal heat perfusion technology, which opens a new horizon for chemo-hyperthermia [Citation116]. Hyperthermia enhances drug-induced DNA adduct formation and single-strand breaks, as well as inhibiting DNA repair [Citation5, Citation117]. Numerous clinical trials have shown that the overall cytotoxic effect of various chemotherapeutic agents is enhanced with increasing tumor temperature [Citation118]. The traditional view analyses the mechanism of action of hyperthermia from the DNA perspective, but this does not fully explain the various clinical effects of chemo-hyperthermia. Therefore, we tried to explore the promotional effect of hyperthermia on chemotherapy from a metabolic perspective. The principles by which hyperthermia combined with chemotherapy affects tumor metabolism can be broadly divided into two kinds: one is that hyperthermia can amplify or attenuate the metabolic regulatory effects of the chemotherapeutic drugs, and the other is the metabolic stress changes made by the tumor cells under the combined effect of both.
Lonidamine (LND) is able to alter the energy metabolism of tumor cells, mainly involving the inhibition of glycolysis, monocarboxylate transporter (MCT), mitochondrial pyruvate carrier (MPC), respiratory chain complex I/II, mitochondrial permeability transition (PT) pore and hexokinase II (HK-II) [Citation119]. It has a weak antitumor effect by itself, and when combined with hyperthermia, heating causes tumor cells to form cohesive mitochondria in vivo, which can enhance its anticancer activity [Citation120]. Another study in Dunning R3327G rats with prostate cancer showed that LND significantly reduced heat dose without compromising anticancer efficacy and avoided HT-induced complications [Citation121]. A similar chemotherapeutic agent, nonivamide, could reduce lipid accumulation [Citation122] and inhibit free fatty acid uptake [Citation123]. Cells exposed to combination therapy (hyperthermia at 44 °C for 15 min and nonivamide) exhibited greater reductions in MMP, more ROS production, and over-activation of the mitochondrial apoptotic pathway [Citation124].
As the center of metabolism, mitochondria are one of the targets of many chemotherapeutic drugs [Citation125]. In the experiment by Shahbaz et al. the combination of hyperthermia (44 °C, 12 min) and the flavonoid compound baicalin (BCN) significantly increases the human U937 mitochondrial free calcium compared to BCN alone [Citation126]. On the one hand, it has long been recognized that heat can affect the distribution of plasma membrane proteins and alter the permeability of the plasma membrane, leading to a sudden increase in Ca2+ [Citation127], and the increase in mitochondrial free calcium may be related to the dysfunction of the mitochondrial Ca2+ uniporter (MCU). On the other hand, high temperature leads to a large increase (3–5 times) in intracellular free calcium, which is due to the release of Ca2+ stored in the endoplasmic reticulum and the influx of Ca2+ from the extracellular medium into the cytoplasm during heating [Citation128, Citation129]. Calcium signaling is critical for cell fate by affecting processes of mitochondrial interconnectivity, such as energy production and apoptosis [Citation130]. When intracellular Ca2+ signals are elevated, calcium-binding proteins bind to Ca2+ and activate inositol-1, 4, 5-triphosphate receptor (IP3R) and ryanodine receptor (RyR) channels, resulting in the release of large amounts of Ca2+ from the endoplasmic reticulum into the cytoplasm, which in turn activates a series of calcium-dependent degradative enzymes, leading to the destruction of cell membranes or organelle membranes, and ultimately leading to the development of apoptosis [Citation131]. In addition, increased ROS induced by mitochondrial Ca2+ overload plays a major role in apoptosis of tumor cells [Citation132]. The imbalance of Ca2+ means the loss of its normal function and increased necrosis of the tumor cells. It should be noted, however, that this process is also present in normal cells adjacent to the cancer. How to specifically adjust the calcium ion concentration of tumor cells without affecting the surrounding normal cells is the key to the study. Because of different cell types, metabolic changes in tumors can be different even with the same treatment. The combination of hyperthermia (43 °C, 1h) and cisplatin can downregulate glutamate dehydrogenase (GDH) activity in ovarian adenocarcinoma OVCAR-3 cells, inhibit mitochondrial respiration rate and increase apoptosis [Citation133], whereas the same treatment has no significant effect in human AGS (a human gastric adenocarcinoma cell-line) and T3M4 (a human pancreatic cancer cell-line) cancer cell lines [Citation134].
In addition, the metabolic changes induced by the combination of hyperthermia and chemotherapeutic agents are not always in the direction of tumor suppression. Tumor cells treated with the combination of hyperthermia (42 °C water bath for 15 or 30 min) and adriamycin have higher levels of ROS than cells treated with hyperthermia or adriamycin alone, but the levels of tricarboxylic acid cycle (TAC) are higher in hyperthermia-treated cells, and high TAC levels can help tumor cells maintain high redox homeostasis and thus survive under heat stress [Citation135].
Therefore, when combining hyperthermia with chemotherapeutic drugs, it is important to understand that different cancer types will respond differently to the same drugs. Not only the amplified killing effect but also the resistance effect that may be induced should be taken into account. Clinicians should fully integrate clinical experience and experimental results when formulating chemotherapy regimens for patients, and weigh the pros and cons of combining chemotherapy with thermotherapy before implementation.
5.2. Hyperthermia combined with radiotherapy
There is also a certain causality between the metabolic modality of tumor cells and the effect of radiotherapy [Citation136, Citation137]. Although it has been demonstrated that radiation can cause membrane lipid peroxidation, leading to dysregulation of membrane lipid ratios [Citation138], it is still unclear whether radiotherapy has any effect on other metabolic modalities of tumor cells. However, it is feasible to enhance the sensitivity of tumor cells to radiotherapy by modulating metabolism. It was found that targeting CPT1A-mediated fatty acid oxidation could sensitize nasopharyngeal carcinoma to radiotherapy [Citation139]. The elevation of fatty acid synthase (FASN) may lead to radiation tolerance in pancreatic cancer [Citation140]; in addition to lipids, the increased degree of glycolysis also plays a key role in promoting radiation tolerance [Citation141], and using lactate metabolism to enhance radiosensitivity is also one of the feasible approaches [Citation142].
Hyperthermia, as the optimal sensitizer for radiation therapy, alters the cellular metabolism, which might be one of its principles for increasing the radiosensitivity of tumor cells. Hypoxia is a key factor affecting radiotherapy [Citation143]. Studies have shown that the efficacy of radiotherapy is dependent on partial pressure of oxygen (pO2) [Citation144]。The O2 enhancement ratio (The ratio of radiation dose required to achieve the same biological effect under aerobic and anaerobic conditions, OER) is generally reported to be 2.7 ∼ 3, Usually when pO2 is below 5 mmHg, tumor cells tend to have a higher radioresistance [Citation145]. And in a hypoxic environment, when cells are exposed to radiation, less ROS are produced, which also reduces the efficacy of radiotherapy [Citation146]. Hyperthermia is able to improve the effect of radiotherapy by increasing the oxygenation status of the tumor microenvironment [Citation147, Citation148]. In addition to improving the vascular oxygen supply capacity [Citation149], hyperthermia can inhibit the activity of metabolic enzymes PKM2 and CPT1A by interfering with the conversion and synthesis of oxygen-consuming metabolic substrates such as fatty acids and glutamine, thereby reducing oxygen consumption, which is also one of the ways to improve the oxygenation status of the tumor microenvironment by hyperthermia. In addition, the effect of radiotherapy is influenced by lactic acid, and studies have shown that cancer cells can exhibit additional radioresistance at lower pH [Citation150]. Results from a study group showed that lactate concentrations are positively correlated with radioresistance [Citation151]. Lactate has antioxidant properties [Citation152], while radiotherapy can induce oxidative stress in target cells and generate large amounts of ROS causing cellular damage. Therefore, the accumulation of antioxidants (e.g. lactate) may induce or enhance the radioresistance of tumor cells [Citation153]. Moreover, lactate can reprogram the antitumor T-cell response to an immunosuppressive state by activating the HIF-1a signaling pathway in cells, inducing a suppressive immune microenvironment and favoring the development of radioresistance [Citation154]. Mild hyperthermia (39.5–42 °C) can increase the pH of the tumor microenvironment, creating a more sensitive environment for radiotherapy to improve its efficacy. The mechanism may be due to the fact that hydrogen ions produced during glycolysis cannot be removed in some tumor regions because of insufficient blood and lymphatic drainage, while hyperthermia can increase tumor blood flow, remove hydrogen ions accumulated in tumor regions, effectively raising the pH of the tumor microenvironment [Citation5]. In addition, hyperthermia can interfere with the aerobic glycolysis of tumor cells [Citation55], thus reducing lactate production. In addition to adjusting cellular metabolism to create favorable conditions for radiotherapy, hyperthermia is also able to amplify the therapeutic effect of radiotherapy by inducing a large production of the metabolic by-product ROS, which is synergistic with radiotherapy-induced apoptosis [Citation155].
The role of cellular metabolism in combined hyperthermia and radiotherapy appears to be less significant than in the synergistic treatment with hyperthermia and chemotherapy. However, in view of the fact that the current research is only limited to the changes in metabolite quantity and the study of more in-depth molecular mechanisms is still very superficial, the future focus can be on the cellular metabolism mechanism of hyperthermia as a radiosensitizer, and it is also a feasible direction to judge the treatment effect and patient prognosis by the changes in metabolic index.
5.3. Hyperthermia combined with other therapies
In addition to the combination with chemotherapy and radiotherapy, other therapies, such as immunotherapy [Citation156], sonodynamic therapy, and photodynamic therapy [Citation157], can also have a good synergistic effect with hyperthermia.
Photodynamic therapy, as a newly developed tumor treatment in recent years [Citation158], also has a unique role in tumor metabolism. It is able to target mitochondria [Citation159] and induce non-enzymatic lipid peroxidation to drive a ferroptosis-like cell death pathway [Citation160]. In a past study, by examining metabolic parameters in mice after combined hyperthermia (localized 43 °C hyperthermia for 1h) and photodynamic therapy, it was found that lactate levels increased significantly and ATP concentrations decreased after the combined treatment [Citation161]. Photothermal therapy promotes the activation of the mitochondrial apoptotic pathway by altering the mitochondrial membrane potential, leading to respiratory chain dysfunction and inducing a significant production of reactive oxygen species [Citation162]. In addition, an in vitro mouse breast cancer cell study demonstrated that hyperthermia (42 °C, 1h) can also regulate the expression of heme carrier protein-1 (HCP-1) and ATP-binding cassette sub-family G member 2 (ABCG2) by generating high levels of ROS to enhance photodynamic therapy [Citation163]. These evidences suggest that hyperthermia can improve photodynamic therapy by modulating tumor metabolism, but the more specific metabolic modalities need to be investigated further.
Although the combination of hyperthermia and immunotherapy has been widely developed in clinical practice, and the theory that hyperthermia can enhance the effectiveness of immunotherapy by activating the immune capacity of the body is well established [Citation164], there are no relevant studies on whether this activation is related to cellular metabolism. Similarly, studies have shown that focused ultrasound (FUS) can alter cell membranes and lead to a decrease in metabolic activity [Citation165]. When FUS was combined with hyperthermia (45 °C, 30 min), tumor cells exhibited lower metabolic activity and significantly lower survival rates compared to hyperthermia alone [Citation166]. Although this could suggest a link between sonodynamic therapy and hyperthermia in metabolism, it is not clear whether hyperthermia can improve the effect of sonodynamic therapy by altering cellular metabolism. Whether photodynamic therapy, sonodynamic therapy, or even hyperthermia, these approaches are not very mature so far and still need to be studied in more depth. Revisiting the combined effect of these therapies from the perspective of metabolism will help to fully understand the changes in various tumor treatments to further understand the tumor killing mechanism, which is of great guidance for further expanding the tumor treatment methods.
6. Conclusion and prospect
Metabolic reprogramming of glucose, cholesterol, fatty acids, and amino acids plays an extremely important role in the development of tumors, and sorting out the different metabolic changes provides a more adequate theoretical guide to clarify the mechanisms of tumorigenesis. However, despite the long-standing experimental evidence on the relationship between hyperthermia and cellular energy metabolism, the specific mechanisms and extent of the effects of changes in the amounts or properties of these metabolic substances on tumorigenesis or progression have not been fully elucidated and still need further validation and exploration in the future. In addition, due to the diversity of metabolic substances inside and outside cells and the complexity of their mechanisms of action, it is difficult to block tumorigenesis or progression to a large extent by metabolic regulation of a specific substance, which is one of the reasons why there are few cases of combined application of metabolic reprogramming to clinical treatment for tumors. Therefore, future experiments on the effects of metabolic reprogramming on tumors should focus on exploring the key factors affecting tumorigenesis and progression, so as to make the treatment more targeted and promote its further application in the clinic. Furthermore, existing research indicates that tumor metabolic reprogramming is closely linked to the development of resistance to chemotherapy and radiotherapy; thus, the regulation of specific substances to reduce tumor cell resistance to radiotherapy and chemotherapy is one of the promising directions for future clinical translation.
Currently, we have made some progress in the research related to the effect of hyperthermia on the metabolism and, thus, the treatment of tumors. By detecting plasma levels of metabolic substances, such as lactate and lipoproteins, in tumor patients after hyperthermia, it is possible to indirectly reflect the metabolic levels of tumor cells in vivo. Compared with in vitro experiments, it has more limitations, but it is able to maintain consistency in the overall therapeutic trend. As an excellent adjuvant therapy, at therapeutic temperatures (39–44 °C), it not only provides a more appropriate environment for radiotherapy, chemotherapy and immunotherapy, but also amplifies the effect of other therapies by interfering with tumor metabolism. When the temperature is too high (>44 °C), or even reaches the ablation temperature, hyperthermia is able to roughly destroy the metabolic function of tumor cells, and the killing effect on the tumor is direct. However, this also faces a key problem, how to control the hyperthermia to act precisely on tumor cells without interfering with normal cells? Whole-body and localized hyperthermia, high-temperature and low-temperature hyperthermia, individual hyperthermia and combined treatment, these are the problems that need to be solved for the broader application of hyperthermia.
Hyperthermia can widely affect the metabolism of multiple substances to treat tumors, avoiding the drawback that drugs can only regulate the metabolism of a single substance or a class of substances and cannot maximize the therapeutic effect. However, according to the current experimental results, the efficacy of hyperthermia on tumors is dual in a certain temperature range. Under certain temperatures, hyperthermia can effectively kill tumor cells, but sublethal heat stress not only fails to have a therapeutic effect but also contributes to the formation of heat resistance in cancer cells and tumor recurrence and invasion under certain circumstances. Moreover, due to the wide variety of intracellular substances, the degree of metabolic effects of hyperthermia on different substances at the same temperature varies, and even the effects on the same substance vary within different types of tumor cells. In addition, tumorigenesis is accompanied by the metabolic reprogramming of multiple substances, and regulating a single reprogramming node may not have a good effect. Therefore, the combination of multiple therapeutic modalities and targeted implementation of therapeutic regimens are needed to achieve more efficient therapeutic effects. Moreover, the effect of hyperthermia combined with other therapies on metabolic regulation is not unidirectional, and changes in metabolic levels after treatment can in turn improve the efficacy of the corresponding therapeutic modalities by altering the resistance of tumor cells to treatment, and so forth. Therefore, the artificial adjustment of metabolic patterns to improve the effectiveness of clinical treatment deserves more in-depth study, which is of great significance to optimize and expand the existing therapeutic tools.
Disclosure statement
No potential conflict of interest was reported by the author(s).
Data availability statements
Data availability is not applicable to this article as no new data were created or analyzed in this study.
Additional information
Funding
Reference
- Xiao S, Wu Z, Zhang K. Chinese expert consensus on tumor thermotherapy. J Pract Oncol. 2020;35(1):1–10. doi:10.13267/j.cnki.syzlzz.2020.01.001.
- Datta NR, Ordóñez SG, Gaipl US, et al. Local hyperthermia combined with radiotherapy and-/or chemotherapy: recent advances and promises for the future. Cancer Treat Rev. 2015;41(9):742–753. doi:10.1016/j.ctrv.2015.05.009.
- van der Zee J. Heating the patient: a promising approach? Ann Oncol. 2002;13(8):1173–1184. doi:10.1093/annonc/mdf280.
- Takahashi I, Emi Y, Hasuda S, et al. Clinical application of hyperthermia combined with anticancer drugs for the treatment of solid tumors. Surgery. 2002;131(1 Suppl):S78–S84. doi:10.1067/msy.2002.119308.
- Dunne M, Regenold M, Allen C. Hyperthermia can alter tumor physiology and improve chemo- and radio-therapy efficacy. Adv Drug Deliv Rev. 2020;163–164:98–124. doi:10.1016/j.addr.2020.07.007.
- Takemoto M, Kuroda M, Urano M, et al. The effect of various chemotherapeutic agents given with mild hyperthermia on different types of tumours. Int J Hyperthermia. 2003;19(2):193–203. doi:10.1080/0265673021000035235.
- Ostberg JR, Repasky EA. Comparison of the effects of two different whole body hyperthermia protocols on the distribution of murine leukocyte populations. Int J Hyperthermia. 2000;16(1):29–43. doi:10.1080/026567300285402.
- Hanahan D, Weinberg RA. Hallmarks of cancer: the next generation. Cell. 2011;144(5):646–674. doi:10.1016/j.cell.2011.02.013.
- Biswas SK. Metabolic reprogramming of immune cells in cancer progression. Immunity. 2015;43(3):435–449. doi:10.1016/j.immuni.2015.09.001.
- Kim J, DeBerardinis RJ. Mechanisms and implications of metabolic heterogeneity in cancer. Cell Metab. 2019;30(3):434–446. doi:10.1016/j.cmet.2019.08.013.
- Boroughs LK, DeBerardinis RJ. Metabolic pathways promoting cancer cell survival and growth. Nat Cell Biol. 2015;17(4):351–359. doi:10.1038/ncb3124.
- Streffer C. Metabolic changes during and after hyperthermia. Int J Hyperthermia. 1985;1(4):305–319. doi:10.3109/02656738509029295.
- Streffer C. Aspects of metabolic change after hyperthermia. Recent Results Cancer Res. 1988;107:7–16. doi:10.1007/978-3-642-83260-4_2.
- Hanahan D. Hallmarks of cancer: new dimensions. Cancer Discov. 2022;12(1):31–46. doi:10.1158/2159-8290.CD-21-1059.
- Schiliro C, Firestein BL. Mechanisms of metabolic reprogramming in cancer cells supporting enhanced growth and proliferation [published correction appears in cells. Cells. 2021;10(5):1056. Published 2021 Apr 29. doi:10.3390/cells10051056.
- Bose S, Zhang C, Le A. Glucose metabolism in cancer: the Warburg effect and Beyond. Adv Exp Med Biol. 2021;1311:3–15. doi:10.1007/978-3-030-65768-0_1.
- Vaupel P, Multhoff G. Revisiting the Warburg effect: historical dogma versus current understanding. J Physiol. 2021;599(6):1745–1757. doi:10.1113/JP278810.
- Marcucci F, Rumio C. Glycolysis-induced drug resistance in tumors-A response to danger signals? Neoplasia. 2021;23(2):234–245. doi:10.1016/j.neo.2020.12.009.
- Lu J. The Warburg metabolism fuels tumor metastasis. Cancer Metastasis Rev. 2019;38(1-2):157–164. doi:10.1007/s10555-019-09794-5.
- Ganapathy-Kanniappan S. Linking tumor glycolysis and immune evasion in cancer: emerging concepts and therapeutic opportunities. Biochim Biophys Acta Rev Cancer. 2017;1868(1):212–220. doi:10.1016/j.bbcan.2017.04.002.
- Holthuis JC, Menon AK. Lipid landscapes and pipelines in membrane homeostasis. Nature. 2014;510(7503):48–57. doi:10.1038/nature13474.
- Efeyan A, Comb WC, Sabatini DM. Nutrient-sensing mechanisms and pathways. Nature. 2015;517(7534):302–310. doi:10.1038/nature14190.
- Zhao J, Zhi Z, Wang C, et al. Exogenous lipids promote the growth of breast cancer cells via CD36. Oncol Rep. 2017;38(4):2105–2115. doi:10.3892/or.2017.5864.
- Geng F, Guo D. Lipid droplets, potential biomarker and metabolic target in glioblastoma. Intern Med Rev (Wash D C). 2017;3(5):10–18103. /imrv3i5443 doi:10.18103/imr.v3i5.443.
- Röhrig F, Schulze A. The multifaceted roles of fatty acid synthesis in cancer. Nat Rev Cancer. 2016;16(11):732–749. doi:10.1038/nrc.2016.89.
- Chandel NS. Amino acid metabolism. Cold Spring Harb Perspect Biol. 2021;13(4):a040584. Published 2021 Apr1. doi:10.1101/cshperspect.a040584.
- Lieu EL, Nguyen T, Rhyne S, et al. Amino acids in cancer. Exp Mol Med. 2020;52(1):15–30. doi:10.1038/s12276-020-0375-3.
- Yoo HC, Yu YC, Sung Y, et al. Glutamine reliance in cell metabolism. Exp Mol Med. 2020;52(9):1496–1516. doi:10.1038/s12276-020-00504-.
- Li AM, Ye J. Reprogramming of serine, glycine and one-carbon metabolism in cancer. Biochim Biophys Acta Mol Basis Dis. 2020;1866(10):165841. doi:10.1016/j.bbadis.2020.165841.
- Phang JM. Proline metabolism in cell regulation and cancer biology: recent advances and hypotheses. Antioxid Redox Signal. 2019;30(4):635–649. doi:10.1089/ars.2017.7350.
- Wang W, Zou W. Amino acids and their transporters in T cell immunity and cancer therapy. Mol Cell. 2020;80(3):384–395. doi:10.1016/j.molcel.2020.09.006.
- Vettore L, Westbrook RL, Tennant DA. New aspects of amino acid metabolism in cancer. Br J Cancer. 2020;122(2):150–156. doi:10.1038/s41416-019-0620-5.
- Yoshida GJ. Metabolic reprogramming: the emerging concept and associated therapeutic strategies. J Exp Clin Cancer Res. 2015;34(1):111. Published 2015 Oct6. doi:10.1186/s13046-015-0221-y.
- Xia L, Oyang L, Lin J, et al. The cancer metabolic reprogramming and immune response. Mol Cancer. 2021;20(1):28. Published 2021 Feb5. doi:10.1186/s12943-021-01316-8.
- Lian X, Yang K, Li R, et al. Immunometabolic rewiring in tumorigenesis and anti-tumor immunotherapy. Mol Cancer. 2022;21(1):27. Published 2022 Jan 21. doi:10.1186/s12943-021-01486-5.
- Guo D, Tong Y, Jiang X, et al. Aerobic glycolysis promotes tumor immune evasion by hexokinase2-mediated phosphorylation of IκBα. Cell Metab. 2022;34(9):1312–1324.e6. doi:10.1016/j.cmet.2022.08.002.
- Chen J, Cao X, Li B, et al. Warburg effect is a cancer immune evasion mechanism against macrophage immunosurveillance. Front Immunol. 2020;11:621757. Published 2021 Feb2. doi:10.3389/fimmu.2020.621757.
- Liu X, Hartman CL, Li L, et al. Reprogramming lipid metabolism prevents effector T cell senescence and enhances tumor immunotherapy. Sci Transl Med. 2021;13(587):eaaz6314. doi:10.1126/scitranslmed.aaz6314.
- Poznanski SM, Singh K, Ritchie TM, et al. Metabolic flexibility determines human NK cell functional fate in the tumor microenvironment. Cell Metab. 2021;33(6):1205–1220.e5. doi:10.1016/j.cmet.2021.03.023.
- Morandi A, Indraccolo S. Linking metabolic reprogramming to therapy resistance in cancer. Biochim Biophys Acta Rev Cancer. 2017;1868(1):1–6. doi:10.1016/j.bbcan.2016.12.004.
- Peng J, Cui Y, Xu S, et al. Altered glycolysis results in drug-resistant in clinical tumor therapy. Oncol Lett. 2021;21(5):369. doi:10.3892/ol.2021.12630.
- Ma L, Zong X. Metabolic symbiosis in chemoresistance: refocusing the role of aerobic glycolysis. Front Oncol. 2020;10:5. Published 2020 Jan 24. doi:10.3389/fonc.2020.00005.
- Cao Y. Adipocyte and lipid metabolism in cancer drug resistance. J Clin Invest. 2019;129(8):3006–3017. Published 2019 Jul 2. doi:10.1172/JCI127201.
- Bacci M, Lorito N, Smiriglia A, et al. Fat and furious: lipid metabolism in antitumoral therapy response and resistance. Trends Cancer. 2021;7(3):198–213. doi:10.1016/j.trecan.2020.10.004.
- Pranzini E, Pardella E, Paoli P, et al. Metabolic reprogramming in anticancer drug resistance: a focus on amino acids. Trends Cancer. 2021;7(8):682–699. doi:10.1016/j.trecan.2021.02.004.
- Pan Y, Cao M, Liu J, et al. Metabolic regulation in mitochondria and drug resistance. Adv Exp Med Biol. 2017;1038:149–171. doi:10.1007/978-981-10-6674-0_11.
- Lin J, Xia L, Liang J, et al. The roles of glucose metabolic reprogramming in chemo- and radio-resistance. J Exp Clin Cancer Res. 2019;38(1):218. Published 2019 May 23. doi:10.1186/s13046-019-1214-z.
- Huang C, Su L, Chen Y, et al. Ceramide kinase confers tamoxifen resistance in estrogen receptor-positive breast cancer by altering sphingolipid metabolism [published online ahead of print, 2022 nov 21]. Pharmacol Res. 2023;187:106558. doi:10.1016/j.phrs.2022.106558.
- Li X, Lu J, Kan Q, et al. Metabolic reprogramming is associated with flavopiridol resistance in prostate cancer DU145 cells. Sci Rep. 2017;7(1):5081. Published 2017 Jul 11. doi:10.1038/s41598-017-05086-6.
- Fang Y, Zhan Y, Xie Y, et al. Integration of glucose and cardiolipin anabolism confers radiation resistance of HCC. Hepatology. 2022;75(6):1386–1401. doi:10.1002/hep.32177.
- Sánchez-Castillo A, Vooijs M, Kampen KR. Linking serine/glycine metabolism to radiotherapy resistance. Cancers (Basel). 2021;13(6):1191. Published 2021 Mar 10. doi:10.3390/cancers13061191.
- Paul S, Ghosh S, Kumar S. Tumor glycolysis, an essential sweet tooth of tumor cells. Semin Cancer Biol. 2022;86(Pt 3):1216–1230. doi:10.1016/j.semcancer.2022.09.007.
- Bose S, Le A. Glucose metabolism in cancer. Adv Exp Med Biol. 2018;1063:3–12. doi:10.1007/978-3-319-77736-8_1.
- Gupta V, Bamezai RN. Human pyruvate kinase M2:a multifunctional protein. Protein Sci. 2010;19(11):2031–2044. doi:10.1002/pro.505.
- Dang J, Ye H, Li Y, et al. Multivalency-assisted membrane-penetrating siRNA delivery sensitizes photothermal ablation via inhibition of tumor glycolysis metabolism. Biomaterials. 2019;223:119463. doi:10.1016/j.biomaterials.2019.119463.
- Mitov MI, Harris JW, Alstott MC, et al. Temperature induces significant changes in both glycolytic reserve and mitochondrial spare respiratory capacity in colorectal cancer cell lines. Exp Cell Res. 2017;354(2):112–121. doi:10.1016/j.yexcr.2017.03.046.
- Moon EJ, Sonveaux P, Porporato PE, et al. NADPH oxidase-mediated reactive oxygen species production activates hypoxia-inducible factor-1 (HIF-1) via the ERK pathway after hyperthermia treatment. Proc Natl Acad Sci U S A. 2010;107(47):20477–20482. doi:10.1073/pnas.1006646107.
- Kim JW, Tchernyshyov I, Semenza GL, et al. HIF-1-mediated expression of pyruvate dehydrogenase kinase: a metabolic switch required for cellular adaptation to hypoxia. Cell Metab. 2006;3(3):177–185. doi:10.1016/j.cmet.2006.02.002.
- Ancey PB, Contat C, Meylan E. Glucose transporters in cancer - from tumor cells to the tumor microenvironment. Febs J. 2018;285(16):2926–2943. doi:10.1111/febs.14577.
- Presley T, Vedam K, Druhan LJ, et al. Hyperthermia-induced Hsp90·eNOS preserves mitochondrial respiration in hyperglycemic endothelial cells by down-regulating glut-1 and up-regulating G6PD activity. J Biol Chem. 2010;285(49):38194–38203. doi:10.1074/jbc.M110.147728.
- Chen Y, Bei J, Liu M, et al. Sublethal heat stress-induced O-GlcNAcylation coordinates the Warburg effect to promote hepatocellular carcinoma recurrence and metastasis after thermal ablation. Cancer Lett. 2021;518:23–34. doi:10.1016/j.canlet.2021.06.001.
- Wu J, Liu T, Rios Z, et al. Heat shock proteins and cancer. Trends Pharmacol Sci. 2017;38(3):226–256. doi:10.1016/j.tips.2016.11.009.
- Dayton TL, Jacks T, Vander Heiden MG. PKM2, cancer metabolism, and the road ahead. EMBO Rep. 2016;17(12):1721–1730. doi:10.15252/embr.201643300.
- Azoitei N, Becher A, Steinestel K, et al. PKM2 promotes tumor angiogenesis by regulating HIF-1α through NF-κB activation. Mol Cancer. 2016;15(1):3. Published 2016 Jan6. doi:10.1186/s12943-015-0490-2.
- Yang W, Xia Y, Hawke D, et al. PKM2 phosphorylates histone H3 and promotes gene transcription and tumorigenesis [published correction appears in cell. 2014 aug 28;158(5):1210]. Cell. 2012;150(4):685–696. doi:10.1016/j.cell.2012.07.018.
- Hou PP, Luo LJ, Chen HZ, et al. Ectosomal PKM2 promotes HCC by inducing macrophage differentiation and remodeling the tumor microenvironment. Mol Cell. 2020;78(6):1192–1206.e10. doi:10.1016/j.molcel.2020.05.004.
- Ren J, Zhang L, Zhang J, et al. Light-activated oxygen self-supplied starving therapy in near-infrared (NIR) window and adjuvant hyperthermia-induced tumor ablation with an augmented sensitivity. Biomaterials. 2020;234:119771. doi:10.1016/j.biomaterials.2020.119771.
- Chen WH, Luo GF, Lei Q, et al. Overcoming the heat endurance of tumor cells by interfering with the anaerobic glycolysis metabolism for improved photothermal therapy. ACS Nano. 2017;11(2):1419–1431. doi:10.1021/acsnano.6b06658.
- Ding XL, Liu MD, Cheng Q, et al. Multifunctional liquid metal-based nanoparticles with glycolysis and mitochondrial metabolism inhibition for tumor photothermal therapy. Biomaterials. 2022;281:121369. doi:10.1016/j.biomaterials.2022.121369.
- Yoshikawa T, Kokura S, Tainaka K, et al. The role of active oxygen species and lipid peroxidation in the antitumor effect of hyperthermia. Cancer Res. 1993;53(10 Suppl):2326–2329.
- Li Y, Wang D, Ping X, et al. Local hyperthermia therapy induces browning of white fat and treats obesity. Cell. 2022;185(6):949–966.e19. doi:10.1016/j.cell.2022.02.004.
- Feingold KR. Lipid and lipoprotein metabolism. Endocrinol Metab Clin North Am. 2022;51(3):437–458. doi:10.1016/j.ecl.2022.02.008.
- Efremov AV, Khegai II, Molokov KV, et al. Changes in lipid metabolism during walker 256 tumor growth and the therapeutic effect of hyperthermia. Bull Exp Biol Med. 2014;156(6):838–840. doi:10.1007/s10517-014-2464-6.
- Rios Garcia M, Steinbauer B, Srivastava K, et al. Acetyl-CoA carboxylase 1-Dependent protein acetylation controls breast cancer metastasis and recurrence. Cell Metab. 2017;26(6):842–855.e5. doi:10.1016/j.cmet.2017.09.018.
- Huang S. Mechanistic study on the reprogramming of tumor lipid oxidation metabolism and interaction with macrophages under heat stress. Shanghai Jiaotong University; 2015.
- Petronini PG, Caccamo AE, Alfieri RR, et al. The effect of heat shock on amino acid transport and cell volume in 3T3 cells. Amino Acids. 2001;20(4):363–380. doi:10.1007/s007260170033.
- Wischmeyer PE. Glutamine: the first clinically relevant pharmacological regulator of heat shock protein expression? Curr Opin Clin Nutr Metab Care. 2006;9(3):201–206. doi:10.1097/01.mco.0000222100.44256.6b.
- Lv H, Zhen C, Liu J, et al. Unraveling the potential role of glutathione in multiple forms of cell death in cancer therapy. Oxid Med Cell Longev. 2019;2019:3150145. Published 2019 Jun 10. doi:10.1155/2019/3150145.
- Tchouagué M, Grondin M, Glory A, et al. Heat shock induces the cellular antioxidant defenses peroxiredoxin, glutathione and glucose 6-phosphate dehydrogenase through Nrf2. Chem Biol Interact. 2019;310:108717. doi:10.1016/j.cbi.2019.06.030.
- Liu N, Li B, Gong C, et al. A pH- and thermo-responsive poly(amino acid)-based drug delivery system. Colloids Surf B Biointerfaces. 2015;136:562–569. doi:10.1016/j.colsurfb.2015.09.057.
- Lu J, Li Y, Hu D, et al. Synthesis and properties of pH-, thermo-, and Salt-Sensitive modified poly(aspartic acid)/poly(vinyl alcohol) IPN hydrogel and its drug controlled release. Biomed Res Int. 2015;2015:236745. doi:10.1155/2015/236745.
- Zhou F, Yang S, Zhao C, et al. γ-Glutamyl transpeptidase-activatable near-infrared nanoassembly for tumor fluorescence imaging-guided photothermal therapy. Theranostics. 2021;11(14):7045–7056. Published 2021 May 13. doi:10.7150/thno.60586.
- Spinelli JB, Haigis MC. The multifaceted contributions of mitochondria to cellular metabolism. Nat Cell Biol. 2018;20(7):745–754. doi:10.1038/s41556-018-0124-1.
- Nunnari J, Suomalainen A. Mitochondria: in sickness and in health. Cell. 2012;148(6):1145–1159. doi:10.1016/j.cell.2012.02.035.
- Srinivasan S, Guha M, Kashina A, et al. Mitochondrial dysfunction and mitochondrial dynamics-The cancer connection. Biochim Biophys Acta Bioenerg. 2017;1858(8):602–614. doi:10.1016/j.bbabio.2017.01.004.
- Zong WX, Rabinowitz JD, White E. Mitochondria and cancer. Mol Cell. 2016;61(5):667–676. doi:10.1016/j.molcel.2016.02.011.
- Mik EG, Balestra GM, Harms FA. Monitoring mitochondrial PO2: the next step. Curr Opin Crit Care. 2020;26(3):289–295. doi:10.1097/MCC.0000000000000719.
- Kumar PR, Moore JA, Bowles KM, et al. Mitochondrial oxidative phosphorylation in cutaneous melanoma. Br J Cancer. 2021;124(1):115–123. doi:10.1038/s41416-020-01159-y.
- Žūkienė R, Naučienė Z, Šilkūnienė G, et al. Contribution of mitochondria to injury of hepatocytes and liver tissue by hyperthermia. Medicina (Kaunas). 2017;53(1):40–49. doi:10.1016/j.medici.2017.01.001.
- Zaib S, Hayyat A, Ali N, et al. Role of mitochondrial membrane potential and lactate dehydrogenase a in apoptosis. Anticancer Agents Med Chem. 2022;22(11):2048–2062. doi:10.2174/1871520621666211126090906.
- Curley SA, Palalon F, Sanders KE, et al. The effects of non-invasive radiofrequency treatment and hyperthermia on malignant and nonmalignant cells. Int J Environ Res Public Health. 2014;11(9):9142–9153. Published 2014 Sep 3. doi:10.3390/ijerph110909142.
- Yuen WF, Fung KP, Lee CY, et al. Hyperthermia and tumour necrosis factor-alpha induced apoptosis via mitochondrial damage. Life Sci. 2000;67(6):725–732. doi:10.1016/s0024-3205(00)00656-1.
- Wang D, Liu W, Wang L, et al. Suppression of cancer proliferation and metastasis by a versatile nanomedicine integrating photodynamic therapy, photothermal therapy, and enzyme inhibition. Acta Biomater. 2020;113:541–553. doi:10.1016/j.actbio.2020.06.021.
- Ahmed K, Tabuchi Y, Kondo T. Hyperthermia: an effective strategy to induce apoptosis in cancer cells. Apoptosis. 2015;20(11):1411–1419. doi:10.1007/s10495-015-1168-3.
- Hildebrandt B, Wust P, Ahlers O, et al. The cellular and molecular basis of hyperthermia. Crit Rev Oncol Hematol. 2002;43(1):33–56. doi:10.1016/s1040-8428(01)00179-2.
- Segui B, Legembre P. Redistribution of CD95 into the lipid rafts to treat cancer cells? Recent Pat Anticancer Drug Discov. 2010;5(1):22–28. doi:10.2174/157489210789702190.
- Grassmé H, Riethmüller J, Gulbins E. Biological aspects of ceramide-enriched membrane domains. Prog Lipid Res. 2007;46(3-4):161–170. doi:10.1016/j.plipres.2007.03.002.
- Csoboz B, Balogh GE, Kusz E, et al. Membrane fluidity matters: hyperthermia from the aspects of lipids and membranes. Int J Hyperthermia. 2013;29(5):491–499. doi:10.3109/02656736.2013.808765.
- Maulucci G, Cohen O, Daniel B, et al. Fatty acid-related modulations of membrane fluidity in cells: detection and implications. Free Radic Res. 2016;50(sup1):S40–S50. doi:10.1080/10715762.2016.1231403.
- Calderwood SK, Stevenson MA, Price BD. Activation of phospholipase C by heat shock requires GTP analogs and is resistant to pertussis toxin. J Cell Physiol. 1993;156(1):153–159. doi:10.1002/jcp.1041560121.
- de Andrade Mello P, Bian S, Savio LEB, et al. Hyperthermia and associated changes in membrane fluidity potentiate P2X7 activation to promote tumor cell death. Oncotarget. 2017;8(40):67254–67268. Published 2017 Jun 21. doi:10.18632/oncotarget.18595.
- George KS, Wu S. Lipid raft: a floating island of death or survival. Toxicol Appl Pharmacol. 2012;259(3):311–319. doi:10.1016/j.taap.2012.01.007.
- Nagy E, Balogi Z, Gombos I, et al. Hyperfluidization-coupled membrane microdomain reorganization is linked to activation of the heat shock response in a murine melanoma cell line. Proc Natl Acad Sci U S A. 2007;104(19):7945–7950. doi:10.1073/pnas.0702557104.
- Zhao QL, Fujiwara Y, Kondo T. Mechanism of cell death induction by nitroxide and hyperthermia. Free Radic Biol Med. 2006;40(7):1131–1143. doi:10.1016/j.freeradbiomed.2005.10.064.
- Nasr MA, Dovbeshko GI, Bearne SL, et al. Heat shock proteins in the "hot" mitochondrion: identity and putative roles. Bioessays. 2019;41(9):e1900055. doi:10.1002/bies.201900055.
- Slimen IB, Najar T, Ghram A, et al. Reactive oxygen species, heat stress and oxidative induced mitochondrial damage. A review. Int J Hyperthermia. 2014;30(7):513–523. doi:10.3109/02656736.2014.971446.
- Kaminskyy VO, Zhivotovsky B. Free radicals in cross talk between autophagy and apoptosis. Antioxid Redox Signal. 2014;21(1):86–102. doi:10.1089/ars.2013.5746.
- Kassis S, Grondin M, Averill-Bates DA. Heat shock increases levels of reactive oxygen species, autophagy and apoptosis. Biochim Biophys Acta Mol Cell Res. 2021;1868(3):118924. doi:10.1016/j.bbamcr.2020.118924.
- Yang Y, Karakhanova S, Hartwig W, et al. Mitochondria and mitochondrial ROS in cancer: novel targets for anticancer therapy. J Cell Physiol. 2016;231(12):2570–2581. doi:10.1002/jcp.25349.
- Xie S, Sun W, Zhang C, et al. Metabolic control by heat stress determining cell fate to ferroptosis for effective cancer therapy. ACS Nano. 2021;15(4):7179–7194. doi:10.1021/acsnano.1c00380.
- Prescott DM, Charles HC, Sostman HD, et al. Therapy monitoring in human and canine soft tissue sarcomas using magnetic resonance imaging and spectroscopy. Int J Radiat Oncol Biol Phys. 1994;28(2):415–423. doi:10.1016/0360-3016(94)90065-5.
- Markezana A, Ahmed M, Kumar G, et al. Moderate hyperthermic heating encountered during thermal ablation increases tumor cell activity. Int J Hyperthermia. 2020;37(1):119–129. doi:10.1080/02656736.2020.1714084.
- Markezana A, Goldberg SN, Kumar G, et al. Incomplete thermal ablation of tumors promotes increased tumorigenesis. Int J Hyperthermia. 2021;38(1):263–272. doi:10.1080/02656736.2021.1887942.
- van Rhoon GC, Franckena M, Ten Hagen TLM. A moderate thermal dose is sufficient for effective free and TSL based thermochemotherapy. Adv Drug Deliv Rev. 2020;163-164:145–156. doi:10.1016/j.addr.2020.03.006.
- Zhou J, Li L, Li X, et al. Efficacy analysis of a novel thermochemotherapy scheme with pirarubicin for intermediate- and high-risk nonmuscle-invasive bladder cancer: a single-institution nonrandomized concurrent controlled trial. Int J Hyperthermia. 2019;36(1):868–875. doi:10.1080/02656736.2019.1646929.
- Löke DR, Kok HP, Helderman R, et al. Application of HIPEC simulations for optimizing treatment delivery strategies. Int J Hyperthermia. 2023;40(1):2218627. doi:10.1080/02656736.2023.2218627.
- Oei AL, Kok HP, Oei SB, et al. Molecular and biological rationale of hyperthermia as radio- and chemosensitizer. Adv Drug Deliv Rev. 2020;163-164:84–97. doi:10.1016/j.addr.2020.01.003.
- Dunne M, Dou YN, Drake DM, et al. Hyperthermia-mediated drug delivery induces biological effects at the tumor and molecular levels that improve cisplatin efficacy in triple negative breast cancer. J Control Release. 2018;282:35–45. doi:10.1016/j.jconrel.2018.04.029.
- Nath K, Guo L, Nancolas B, et al. Mechanism of antineoplastic activity of lonidamine. Biochim. Biophys. Acta (BBA)Bioenerg. 2016;1866:151–162.
- Huang Y, Sun G, Sun X, et al. The potential of lonidamine in combination with chemotherapy and physical therapy in cancer treatment. Cancers (Basel). 2020;12(11):3332. Pubshed 2020 Nov 11. doi:10.3390/cancers12113332.
- Bloch WE, Lokeshwar BL, Ferrell SM, et al. Enhancement of hyperthermic toxicity by lonidarnine in the dunning R3327G rat prostatic adenocarcinorna. Prostate. 1994;24(3):131–138. doi:10.1002/pros.2990240306.
- Rohm B, Holik A-K, Kretschy N, et al. Nonivamide enhances miRNA let-7d expression and decreases adipogenesis PPARγ expression in 3T3-L1 cells. J Cell Biochem. 2015;116(6):1153–1163. doi:10.1002/jcb.25052.
- Rohm B, Riedel A, Ley JP, et al. Capsaicin, nonivamide and trans-pellitorine decrease free fatty acid uptake without TRPV1 activation and increase acetyl-coenzyme a synthetase activity in caco-2 cells. Food Funct. 2015;6(1):173–185. doi:10.1039/c4fo00435c.
- Sun L, Cui ZG, Zakki SA, et al. Mechanistic study of nonivamide enhancement of hyperthermia-induced apoptosis in U937 cells. Free Radic Biol Med. 2018;120:147–159. doi:10.1016/j.freeradbiomed.2018.03.017.
- Sainero-Alcolado L, Liaño-Pons J, Ruiz-Pérez MV, et al. Targeting mitochondrial metabolism for precision medicine in cancer. Cell Death Differ. 2022;29(7):1304–1317. doi:10.1038/s41418-022-01022-y.
- Zakki SA, Cui ZG, Sun L, et al. Baicalin augments Hyperthermia-Induced apoptosis in U937 cells and modulates the MAPK pathway via ROS generation. Cell Physiol Biochem. 2018;45(6):2444–2460. doi:10.1159/000488263.
- Calderwood SK, Stevenson MA, Hahn GM. Effects of heat on cell calcium and inositol lipid metabolism. Radiat Res. 1988;113(3):414–425. doi:10.2307/3577239.
- Mikkelsen RB, Stedman T. Cytotoxic hyperthermia and Ca2+ homeostasis: the effect of heat on Ca2+ uptake by nonmitochondrial intracellular Ca2+ stores. Radiat Res. 1990;123(1):82–86. doi:10.2307/3577661.
- Itagaki Y, Akagi K, Uda M, et al. Role of intracellular calcium concentration on tumor cell death from hyperthermia. Oncol Rep. 1998;5(1):139–141. doi:10.3892/or.5.1.139.
- Chernorudskiy AL, Zito E. Regulation of calcium homeostasis by ER redox: a close-up of the ER/mitochondria connection. J Mol Biol. 2017;429(5):620–632. doi:10.1016/j.jmb.2017.01.017.
- Ansari N, Hadi-Alijanvand H, Sabbaghian M, et al. Interaction of 2-APB, dantrolene, and TDMT with IP3R and RyR modulates ER stress-induced programmed cell death I and II in neuron-like PC12 cells: an experimental and computational investigation. J Biomol Struct Dyn. 2014;32(8):1211–1230. doi:10.1080/07391102.2013.812520.
- Peng TI, Jou MJ. Oxidative stress caused by mitochondrial calcium overload. Ann N Y Acad Sci. 2010;1201(1):183–188. doi:10.1111/j.1749-6632.2010.05634.x.
- Sukovas A, Silkuniene G, Trumbeckaite S, et al. Hyperthermia potentiates cisplatin cytotoxicity and negative effects on mitochondrial functions in OVCAR-3 cells. J Bioenerg Biomembr. 2019;51(4):301–310. doi:10.1007/s10863-019-09805-8.
- Trumbeckaite S, Cesna V, Jasukaitiene A, et al. Different mitochondrial response to cisplatin and hyperthermia treatment in human AGS, caco-2 and T3M4 cancer cell lines. J Bioenerg Biomembr. 2018;50(5):329–338. doi:10.1007/s10863-018-9764-x.
- Wang Q, Zhang H, Ren QQ, et al. Sublethal hyperthermia enhances anticancer activity of doxorubicin in chronically hypoxic HepG2 cells through ROS-dependent mechanism. Biosci Rep. 2021;41(6):BSR20210442. doi:10.1042/BSR20210442.
- Spitz DR. Manipulations of redox metabolism for enhancing radiation therapy responses: a historical perspective and novel hypothesis. Semin Radiat Oncol. 2019;29(1):1–5. doi:10.1016/j.semradonc.2018.10.010.
- Kery M, Papandreou I. Emerging strategies to target cancer metabolism and improve radiation therapy outcomes. Br J Radiol. 2020;93(1115):20200067. doi:10.1259/bjr.20200067.
- Mao C, Lei G, Horbath A, et al. Assessment of lipid peroxidation in irradiated cells. Methods Cell Biol. 2022;172:37–50. doi:10.1016/bs.mcb.2022.05.003.
- Tan Z, Xiao L, Tang M, et al. Targeting CPT1A-mediated fatty acid oxidation sensitizes nasopharyngeal carcinoma to radiation therapy. Theranostics. 2018;8(9):2329–2347. Pubshed 2018 Mar 22. doi:10.7150/thno.21451.
- Souchek JJ, Baine MJ, Lin C, et al. Unbiased analysis of pancreatic cancer radiation resistance reveals cholesterol biosynthesis as a novel target for radiosensitisation. Br J Cancer. 2014;111(6):1139–1149. doi:10.1038/bjc.2014.385.
- Gunda V, Souchek J, Abrego J, et al. MUC1-Mediated metabolic alterations regulate response to radiotherapy in pancreatic cancer. Clin Cancer Res. 2017;23(19):5881–5891. doi:10.1158/1078-0432.CCR-17-1151.
- Liu KX, Everdell E, Pal S, et al. Harnessing lactate metabolism for radiosensitization. Front Oncol. 2021;11:672339. Published 2021 Jul 23. doi:10.3389/fonc.2021.672339.
- Telarovic I, Wenger RH, Pruschy M. Interfering with tumor hypoxia for radiotherapy optimization. J Exp Clin Cancer Res. 2021;40(1):197. Published 2021 Jun 21. doi:10.1186/s13046-021-02000-x.
- Collingridge DR, Rockwell S. Pentoxifylline improves the oxygenation and radiation response of BA1112 rat rhabdomyosarcomas and EMT6 mouse mammary carcinomas. Int J Cancer. 2000;90(5):256–264. doi:10.1002/1097-0215(20001020)90:5<256::AID-IJC2>3.0.CO;2-R.
- Overgaard J. Hypoxic radiosensitization: adored and ignored. J Clin Oncol. 2007;25(26):4066–4074. doi:10.1200/JCO.2007.12.7878.
- Peitzsch C, Perrin R, Hill RP, et al. Hypoxia as a biomarker for radioresistant cancer stem cells. Int J Radiat Biol. 2014;90(8):636–652. doi:10.3109/09553002.2014.916841.
- Elming PB, Sørensen BS, Oei AL, et al. Hyperthermia: the optimal treatment to overcome radiation resistant hypoxia. Cancers (Basel). 2019;11(1):60. Published 2019 Jan9. doi:10.3390/cancers11010060.
- Song CW, Park HJ, Lee CK, et al. Implications of increased tumor blood flow and oxygenation caused by mild temperature hyperthermia in tumor treatment. Int J Hyperthermia. 2005;21(8):761–767. doi:10.1080/02656730500204487.
- Sun X, Xing L, Ling CC, et al. The effect of mild temperature hyperthermia on tumour hypoxia and blood perfusion: relevance for radiotherapy, vascular targeting and imaging. Int J Hyperthermia. 2010;26(3):224–231. doi:10.3109/02656730903479855.
- Wike-Hooley JL, Haveman J, Reinhold HS. The relevance of tumour pH to the treatment of malignant disease. Radiother Oncol. 1984;2(4):343–366. doi:10.1016/s0167-8140(84)80077-8.
- Sattler UG, Meyer SS, Quennet V, et al. Glycolytic metabolism and tumour response to fractionated irradiation. Radiother Oncol. 2010;94(1):102–109. doi:10.1016/j.radonc.2009.11.007.
- Groussard C, Morel I, Chevanne M, et al. Free radical scavenging and antioxidant effects of lactate ion: an in vitro study. J Appl Physiol (1985). 2000;89(1):169–175. doi:10.1152/jappl.2000.89.1.169.
- Sattler UG, Mueller-Klieser W. The anti-oxidant capacity of tumour glycolysis. Int J Radiat Biol. 2009;85(11):963–971. doi:10.3109/09553000903258889.
- Yang X, Lu Y, Hang J, et al. Lactate-Modulated immunosuppression of Myeloid-Derived suppressor cells contributes to the radioresistance of pancreatic cancer. Cancer Immunol Res. 2020;8(11):1440–1451. doi:10.1158/2326-6066.CIR-20-0111.
- Fu Q, Huang T, Wang X, et al. Association of elevated reactive oxygen species and hyperthermia induced radiosensitivity in cancer stem-like cells. Oncotarget. 2017;8(60):101560–101571. Published 2017 Oct9. doi:10.18632/oncotarget.21678.
- Chang M, Hou Z, Wang M, et al. Recent advances in hyperthermia Therapy-Based synergistic immunotherapy. Adv Mater. 2021;33(4):e2004788. doi:10.1002/adma.202004788.
- Bienia A, Wiecheć-Cudak O, Murzyn AA, et al. Photodynamic therapy and hyperthermia in combination Treatment-Neglected forces in the fight against cancer. Pharmaceutics. 2021;13(8):1147. Published 2021 Jul 27. doi:10.3390/pharmaceutics13081147.
- Shi H, Sadler PJ. How promising is phototherapy for cancer? Br J Cancer. 2020;123(6):871–873. doi:10.1038/s41416-020-0926-3.
- Guo X, Yang N, Ji W, et al. Mito-Bomb: targeting mitochondria for cancer therapy. Adv Mater. 2021;33(43):e2007778. doi:10.1002/adma.202007778.
- Shui S, Zhao Z, Wang H, et al. Non-enzymatic lipid peroxidation initiated by photodynamic therapy drives a distinct ferroptosis-like cell death pathway. Redox Biol. 2021;45:102056. doi:10.1016/j.redox.2021.102056.
- Kelleher DK, Thews O, Scherz A, et al. Combined hyperthermia and chlorophyll-based photodynamic therapy: tumour growth and metabolic microenvironment. Br J Cancer. 2003;89(12):2333–2339. doi:10.1038/sj.bjc.6601457.
- Kadkhoda J, Tarighatnia A, Nader ND, et al. Targeting mitochondria in cancer therapy: insight into photodynamic and photothermal therapies. Life Sci. 2022;307:120898. doi:10.1016/j.lfs.2022.120898.
- Kurokawa H, Ito H, Terasaki M, et al. Hyperthermia enhances photodynamic therapy by regulation of HCP1 and ABCG2 expressions via high level ROS generation. Sci Rep. 2019;9(1):1638. Published 2019 Feb7. doi:10.1038/s41598-018-38460-z.
- Skitzki JJ, Repasky EA, Evans SS. Hyperthermia as an immunotherapy strategy for cancer. Curr Opin Investig Drugs. 2009;10(6):550–558.
- Ray S, Kassan A, Busija AR, et al. The plasma membrane as a capacitor for energy and metabolism. Am J Physiol Cell Physiol. 2016;310(3):C181–C192. doi:10.1152/ajpcell.00087.2015.
- Hu S, Zhang X, Unger M, et al. Focused Ultrasound-Induced cavitation sensitizes cancer cells to radiation therapy and hyperthermia. Cells. 2020;9(12):2595. Published 2020 Dec 3. doi:10.3390/cells9122595.